DOI:
10.1039/D2NJ04671G
(Paper)
New J. Chem., 2023,
47, 147-155
Green and facile synthesis of silicon-doped carbon dots and their use in detection of Hg2+ and visualization of latent fingerprints†
Received
21st September 2022
, Accepted 16th November 2022
First published on 17th November 2022
Abstract
Mercury (Hg) is the most toxic heavy metal and can lead to severe irreversible injury to human health and serious pollution. It is of great significance to explore precise and facile detection methods for preventing environmental pollution and ecological problems. Herein, silicon-doped carbon dots (Si-CDs) with bright blue emission were fabricated and employed as a fluorescent probe for the quantitative detection of Hg2+. Possessing high photostability and excellent solubility in water, the Si-CDs exhibit an efficient response to Hg2+ by a “on–off” switching strategy. As a sensing probe, Si-CDs exhibit a good linear relationship with Hg2+ in the concentration range of 0.008–0.4 μM with a limit of detection of 33 nM. Moreover, the proposed method was successfully applied to monitor Hg2+ in different real water samples with satisfactory recoveries of 96.2%–99.5%, demonstrating their excellent accuracy and environment tolerance. Notably, the Si-CD powder obtained by lyophilization can visualize latent fingerprints with second-level details. The results serve to underscore how CDs designed for multiple applications in detection can be exploited to provide promising multi-functional fluorescent materials.
1 Introduction
Mercury ions (Hg2+) are considered to be one of the most stable and hazardous heavy metal pollutants and can be introduced to water, resulting in the contamination of aquatic ecosystems and the environment.1–3 Trace amounts of Hg2+ can cause serious demonstrable health problems, damaging the central nervous system, endocrine system and other functional barriers.4 More terrifyingly, Hg2+ is non-biodegradable and can accumulate in human bodies through the food chain, which can cause permanent damage to the brain and organs.5 The World Health Organization (WHO) stipulates the maximum concentration of Hg2+ in drinking water must not be more than 30 nM.6 Hence, it is imperative to seek rapid, low cost, sensitive and convenient methods to monitor Hg2+ on-site and protect the global ecosystem from Hg2+ poisoning without delay.
Up to the present, a large number of conventional analytical techniques have been utilized to monitor Hg2+, such as atomic absorption spectrometry (AAS),7,8 inductively coupled plasma mass spectrometry (ICP-MS),9 anodic stripping voltammetry,10 and gas chromatography-mass spectrometry (GC-MS).11 These techniques have been successfully used to trace Hg2+ in many related samples, but the mentioned methods generally require experts to operate under complicated experiment conditions, and the analytical instruments used are expensive and time-consuming, which significantly impedes the development of real-time and on-site detection of Hg2+ in practical applications.12,13 To promote in situ testing of real samples, portable fluorescence detection has been greatly encouraged, with fast response time, high sensitivity and selectivity.14 Therefore, the design and fabrication of fluorescent probes for heavy metal ions with portable, easier operation and high sensitivity is very urgent and desirable. In recent years, fluorescent probes such as semiconductor fluorescent quantum dots,15 metal nanoclusters16 and carbon dots (CDs) have been developed for heavy metal detection.17 In particular, CDs, as novel fluorescent materials with the advantages of non-toxicity, cost-effectiveness, favorable biocompatibility, high water solubility and easy fabrication, stand out in the fields of sensing and imaging, light-emitting devices, biosensors, anti-counterfeiting, and biological imaging.18–21 With their distinguished properties and abundant surface functional groups, CDs have also become exemplary optical sensors for environmental water contaminants.22–24 For instance, Meng et al. developed boron-doped CDs via a one-step hydrothermal method, which were used for highly selective and ultrasensitive detection of Hg2+via a fluorescence quenching effect, and the B-CDs also exhibited satisfactory recovery in actual water samples.25 Liu et al. reported blue fluorescent nitrogen-doped CDs, which were fabricated using anhydrous citric acids and polyethyleneimine as precursors. The N-CDs were used as a fluorescent sensor based on an “on–off–on” mechanism, and could monitor Hg2+ and I− with limits of 3.3 nM and 8.5 nM, respectively.26 The tunable emission of the CDs endows them unique potential advantages in terms of optical properties.27 Easy superficial modification provides the CDs with abundant surface functional groups, which result in good photostability, excellent solubility, and superior selectivity and sensitivity in detection applications. Although great achievements have been made in the detection of heavy metal ions, there is great potential to be explored in the selection of precursors and synthesis strategies.
Based on their excellent optical properties and cost-effective synthesis, CDs have been explored to visualize latent fingerprints instead of conventional materials. The latent fingerprints can be recognized and individual information can be identified using CDs,28 without any possible harmful effects to the operator, which has been proven by many researchers.29 Traditional techniques for identifying fingerprints have mainly adopted lead carbonate/sulfate staining, ferric oxide staining, and powder dusting with fluorescent dye,30 some of which use toxic substances. Although these approaches are reliable techniques for fingerprinting, the injury to operators cannot be ignored.31 Therefore, it is of great significance that bio-compatible/non-toxic CDs can provide accurate detection results without any injury. The application of CDs to fingerprint (FP) testing was first proposed by Kelarakis et al. in 2015.32 Xiong and co-workers combined fluorescent red CD (R-CD) powder with starch to accurately identify several-week-old latent FPs; impressively, they realized the synthesis of CDs with kilogram-scale output, which is of great benefit for commercial application.33 To further promote the photoluminescence performance of CDs and broaden their application in the fields of sensing and fingerprint detection, silicon was selected as the doping heteroatom to obtain CDs with abundant electronic groups and a readily available powder state.34,35
Based on the above considerations, Si-CDs were synthesized as a novel dual-functional fluorescence probe for detecting Hg2+ and visualizing latent fingerprints. Blue fluorescent Si-CDs were fabricated through a facile and economical strategy, and the obtained Si-CDs exhibited a sensitive response to Hg2+ with efficient quenching of emission intensity, which resulted in good performance for the determination of Hg2+ in actual water samples. Additionally, the Si-CD powder obtained by lyophilization could be employed for latent FP detection and visualization. The facile dual-functional Si-CDs provide multiple functional applications and promising possibilities.
2 Experimental
2.1 Materials and instruments
The reagents used were of analytical grade and were used without additional purification. 5-Sulfosalicylic acid (5-SSA) was purchased from Tianjin Kai-tong Chemical Reagent Company, 3-aminopropyl triethoxysilane (APTES) was supplied by Aladdin (Shanghai), and mercuric nitrate and other chemicals were obtained from Beijing Chemical Reagent Company. Bovine serum albumin (BSA), L-methionine (Met), ascorbic acid (AA), glutathione (GSH), cysteine (Cys), glucose (GLU), lysine (Lys), citrulline (Cit), leucine (Leu), threonine (Thr), aspartic acid (Asp) and phenylalanine (Phe) were purchased from Aladdin (Shanghai). Ultrapure water (18.2 MΩ cm−1) from a Millipore water purification system was used for preparing the solutions throughout the experiments.
X-ray diffraction (XRD) data were used to explore the crystalline structure of the Si-CD nanomaterials via a D/Max-2600/PC. The structure and morphology of the Si-CDs were characterized using transmission electron microscopy (TEM) (TECNAI G2 F20, FEI Company, USA). UV-vis absorption spectra were performed on a UV-2550 spectrophotometer (SHIMADZU, Japan). Fourier transform infrared (FTIR) spectra were obtained by mixing samples with KBr and using a JASCOFT/IR-420 (Japan). X-ray photoelectron spectra (XPS) measurements for elemental component analysis of the products were conducted using an AXIS Ultra DLD spectrometer (Japan). The fluorescence spectra and luminescence decay plots of the as-prepared products were captured using a PerkinElmer LS-55 fluorescence spectrometer (Japan) and an FLS980 (America).
2.2 Preparation of Si-CDs
Blue-fluorescent Si-CDs were fabricated via a facile one-step method according to a reported method with some modifications.36 In brief, 0.8 mL APTES was dissolved in 3.2 mL of ultrapure water to produce a clear solution, and then a solution of 5-SSA was prepared by dispersing 0.3651 g 5-SSA into 6 mL ultrapure water. After mixing the two solutions and stirring for 30 min, the Si-CDs were synthesized. Subsequently, the obtained Si-CDs were dialyzed in ultrapure water using a dialysis membrane bag (1 kDa) for 24 h to remove the residual reagents. Finally, the aqueous solution of the purified Si-CDs was kept in a refrigerator (4 °C) as a stock solution for further use, and the Si-CD powder was acquired by lyophilization.
2.3 Fluorescence sensing of Hg2+
To investigate the response ability of the synthesized Si-CDs towards Hg2+, their interactions with a variety of metal cations were examined in water. First of all, the obtained Si-CDs were diluted 100 times and then used as a fluorescent probe. 200 μL of the Si-CD probe was incubated with isometric amounts of different metal ions (Zn2+, Mn2+, Ag+, Mg2+, Ni2+, Co2+, Pb2+, Cr3+, Ba2+, Cd2+, Hg2+, Cu2+, Ca2+, Fe2+, 100 μM) for 6 min in a centrifuge tube, and the fluorescence spectra were recorded under the optimum excitation wavelength of 300 nm. To evaluate the sensitivity of the probe toward Hg2+, a series of Hg2+ standard solutions (0.001–100 μM) were added to the probe Si-CDs. The experimental details for detecting different concentration gradients of Hg2+ were similar to the above operation process. All the measurements were carried out at room temperature and repeated three times to ensure the stability and reliability of the data.
2.4 Analysis of Hg2+ in environmental water samples
The detection of Hg2+ in environmental water samples, including sea water, lake water and tap water, was performed to assess the applicability of the Si-CD probe. Samples were respectively derived from Aershan Lake (Heilongjiang Province), Bohai Sea (Liaoning Province) and tap water from our laboratory. Each sample was first filtered using a 0.22 μM microporous membrane to remove particulate suspension, and then the detection of Hg2+ in real water was performed by the standard addition method.37
2.5 Fingerprint appearance
To visualize latent fingerprints, latent fingerprints were collected from volunteers. The volunteers’ fingers were washed with soap in advance and first gently wiped over the sides of their forehead and nose region. The fingerprints were then pressed on diverse substrates (including silicon wafer, copper sheet, aluminium foil and glass sheet) gently. Si-CD powder was obtained by lyophilization at −50 °C for 24 h. The obtained Si-CD powder was cautiously deposited onto the surfaces of different substrates using a light feather brush. Ultimately, the latent fingerprint images were captured using a mobile phone under a UV lamp.
3 Results and discussion
3.1 Synthesis of Si-CDs
The fabrication of Si-CDs utilized an extremely simple process, which is crucial to further applications for their convenient and large-scale synthesis. As described in Scheme 1, the synthesis of Si-CDs was carried out using 5-SSA and APTES as the carbon and silicon source, respectively. The obtained Si-CDs emitted strong blue fluorescence under a UV lamp, with optimal excitation/emission at 300 nm and 400 nm. Possessing excellent solubility and abundant surface groups, the Si-CDs were employed as an ultra-sensitive probe for monitoring Hg2+ and visualizing latent fingerprints, which revealed their promising applicability as an effective and sensitive sensor. It should be noted that the Si-CDs exhibit promising prospects in the rapid sensing of Hg2+ and latent fingerprint visualization, which broaden the detection performance of Si-CDs as fluorescent probes.
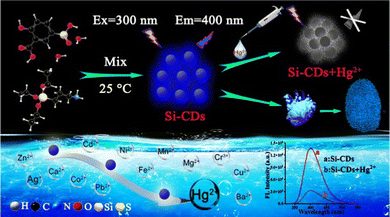 |
| Scheme 1 Strategy for the synthesis of Si-CDs and their utility in the sensing of Hg2+ and latent fingerprint visualization. | |
3.2 Characterization of Si-CDs
The as-synthesized Si-CDs were first characterized using XRD and TEM to explore their components and morphology. The XRD pattern showed an obvious peak centered at around 2θ = 21.5°, which confirmed the successful fabrication of Si-CDs with an amorphous carbon nature (Fig. 1(a)).38 The morphology and size distributions of the Si-CDs were tested using TEM. The TEM image of the Si-CDs exhibited a spherical morphology and good monodispersity with a diameter distribution of 2.24 ± 0.04 nm (Fig. 1(b)). HRTEM (inset of Fig. 1(b)) showed a clear lattice spacing of 0.21 nm, matching the (100) facet of graphite,39 indicating the exact synthesis of CDs. Subsequently, FTIR spectra were obtained to reveal the surface information of the Si-CDs (Fig. 1(c)). The peak at 3439 cm−1 was attributed to the stretching vibrations of the O–H and N–H bonds. The two sharp peaks at 1630 cm−1 and 1587 cm−1 represented the bending vibration of N–H.40 The strong peak observed at 2938 cm−1 corresponded to C–H,41 while those at 1484 cm−1 and 1433 cm−1 correspond to C–N/C–O bonds.42,43 The absorption peaks at 1377 cm−1 and 1336 cm−1 originated from the stretching vibrations of C–N. The characteristic absorption peaks at around 1125–1030 cm−1 were related to the stretching vibration of Si–O–Si;44,45 similarly, the band at 938 cm−1 corresponded to the stretching vibration of the Si–O–H group.46 The above results confirmed that a large number of hydrophilic oxygenated functional groups were distributed on the surface of the Si-CDs, resulting in a preferable water solubility of the Si-CDs and also providing the possibility of coordinating with heavy metals. It is worth noting that the vibrations of Si–O–Si, Si–O–H, and C–N/C–O showed no displacement compared to those of the original raw material APTES, but the intensity and shape exhibited obvious changes, indicating the fabrication of Si-CDs under mild conditions. Additionally, the C–N stretching vibration peak of APTES located at 1337 cm−1 was split into two peaks 1377 cm−1 and 1336 cm−1, indicating the significant variety of the C–N after the formation of CDs. The FTIR spectrum of the Si-CDs indicated that the surface groups of the CDs retained their original activity after the synthesis of Si-CDs. XPS measurements were further used to verify the elemental compositions of the Si-CDs. The full XPS spectrum of the Si-CDs (Fig. S1, ESI†) showed several predominant peaks at 102.2, 150.5, 284.6, 401.2, and 531.7 eV, indicating that the fabricated Si-CDs were composed of the elements Si, C, N, O, with element contents of C (43.94%), N (8.14%), O (36.03%) and Si (11.26%) by elemental analysis, which indicated that the carbon was the dominant constituent of the Si-CDs. Moreover, the high-resolution XPS spectrum of C 1s (Fig. 1(d)) revealed three pronounced peaks centered at binding energies of 284.5, 286.2, 287.9 eV; the peaks at 284.5 eV and 286.2 eV can be assigned to C–C and C–O/C–N, respectively. Additionally, the peak observed at 287.9 eV was assigned to C
O.47 The N 1s peak was split into three peaks at 399.2, 401.3, and 401.9 eV, which can be assigned to N–Si48 and N–H49,50 (Fig. 1(e)). The O 1s bands located at 531.3, 532.2, and 533.2 eV were attributed to C
O,49 O–Si, and C–O,48 respectively. It can be seen in Fig. 1(g) that the Si 2p peak at a binding energy of 102.1 eV was assigned to the Si–O bond,51 indicating the occurrence of Si doping. The XPS analyses were consistent with the FT-IR results, both of which indicated the presence of abundant hydrophilic oxygenated functional groups on the surface of the Si-CDs. The above results demonstrated that Si-CDs were successfully synthesized, and the abundant surface functional groups benefit the solubility and coordination with heavy metals.
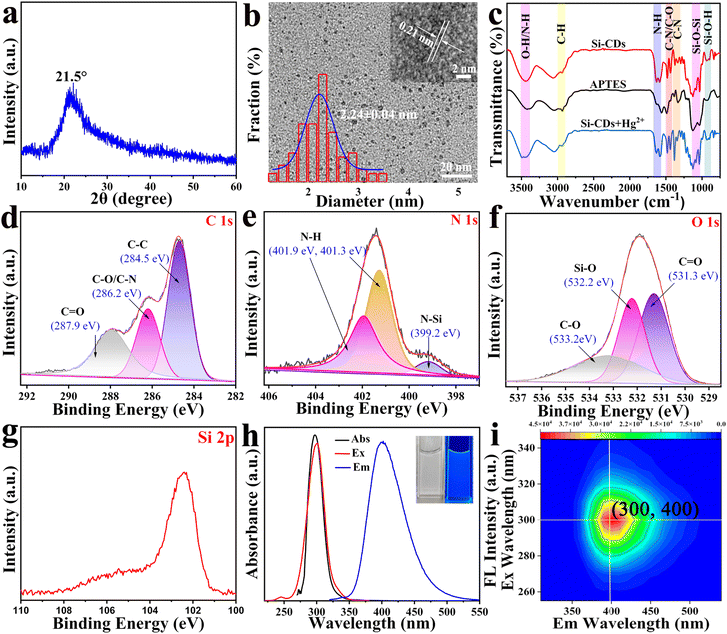 |
| Fig. 1 (a) XRD pattern of the Si-CDs. (b) TEM images of the Si-CDs, with an HRTEM image of a single Si-CD (inset right) and the corresponding distribution histogram (inset left). (c) FTIR spectra of the Si-CDs, APTES and Si-CDs with the addition of Hg2+. High-resolution XPS spectra of (d) C 1s, (e) N 1s, (f) O 1s, and (g) Si 2p, respectively. (h) UV-vis absorption, fluorescence excitation and emission spectra of the Si-CDs; inset: images of Si-CDs under daylight and a UV lamp. (i) 3D contour map of the excitation and emission wavelengths and FL Intensity. | |
3.3 Optical properties of Si-CDs
UV-vis absorption and fluorescence spectra were employed to verify the optical properties of the Si-CDs. Fig. 1(h) shows that the UV-vis absorption spectrum of the Si-CDs covers the wavelength range from 220 to 550 nm, and the obvious absorption peak located at 297 nm can be ascribed to the π–π* electron transition of the C
C band and the n–π* electron transition of the C
O groups.52,53 At the same time, under the optimal excitation wavelength of 300 nm, the maximum fluorescence emission intensity peak of the Si-CDs was centered at 400 nm. The brilliant blue fluorescence of the Si-CDs was visualized under UV light (inset of Fig. 1(h)). The quantum yield of the Si-CDs was calculated to be 23.3% with reference to quinine sulphate.54 The 3D fluorescence diagram of the Si-CDs is displayed in Fig. 1(i) and indicated the excitation-independent property of the Si-CDs,55 which can be attributed to the uniformity of the size and surface modification of the Si-CDs.56
3.4 Stability of Si-CDs
To evaluate the stability of the Si-CDs, the fluorescence of the Si-CDs under several different conditions (pH, ionic strength, and storage time) was investigated. As portrayed in Fig. S2a (ESI†), varying the pH value from 3 to 12 did not significantly affect the fluorescence intensity of the Si-CDs, which indicated that Si-CDs exhibit excellent stability and pH tolerance. Furthermore, Fig. S2b (ESI†) shows that the fluorescence intensity of Si-CDs showed no significant change over a large concentration range of 0.2 M to 1.0 M of NaCl, illustrating the good salt tolerance of the Si-CDs. The photostability with storage time was also recorded (Fig. S2c, ESI†) and exhibited negligible change, indicating the good fluorescence durability of the Si-CDs. The above results proved the excellent photostability of the Si-CDs, which endowed them with great possibility for chemical sensing.
3.5 Selectivity and anti-interference ability of Si-CDs
The Hg2+ detection performance of the Si-CDs was evaluated by testing their selectivity and sensitivity. Firstly, the incubation time of the Si-CD probe with the target ions was considered to acquire optimum detection performance. As shown in Fig. S3 (ESI†), after the addition of 100 μM of Hg2+, the fluorescence intensity of the Si-CDs was acutely decreased within 6 min, and further prolongation of the incubation time resulted in no obvious decrease; thus, the response time was determined to be 6 min. To evaluate the specificity of the Si-CDs in detecting Hg2+, the fluorescence intensity of Si-CDs interacting with 100 μM of different metal ions (Zn2+, Mn2+, Ag+, Mg2+, Ni2+, Co2+, Pb2+, Cr3+, Ba2+, Cd2+, Hg2+, Cu2+, Ca2+, Fe2+, and blank) were recorded at room temperature (Fig. 2(a)). Compared with the blank, all other metal ions showed a negligible influence on the fluorescence intensity. However, the fluorescence intensity of the Si-CDs was dramatically reduced by Hg2+, indicating the good specificity of Si-CDs towards Hg2+. Additionally, photographic images of the corresponding samples under UV-light can be observed in the Fig. 2(a) inset, which were consistent with the results of fluorescence, verifying their good selectivity. To further confirm the superior performance of the Si-CDs in the determination of Hg2+, the influence of different biomolecules (Cys, Phe, BSA, Met, GSH, Cit, AA, Lys, Leu, GLU, Thr and Asp, 200 μM) on the fluorescence intensity of Si-CDs was explored in Fig. 2(b). The results also exhibited the negligible variation in the Si-CD system, demonstrating the high selectivity of the Si-CDs toward Hg2+. Additionally, the color of the corresponding fluorescence remained bright blue, and the fluorescence could clearly be observed under UV light (Fig. 2(b) inset), indicating no interference with the detection. Moreover, when Hg2+ coexisted with potentially interfering metal ions and biomolecules (Fig. 2(c) and (d)), the detection of Hg2+ was realized without an evident effect, indicating the good anti-interference capability of the Si-CDs. These results revealed that Si-CDs could be employed to accurately monitor Hg2+ based on their strong anti-interference ability in this study.
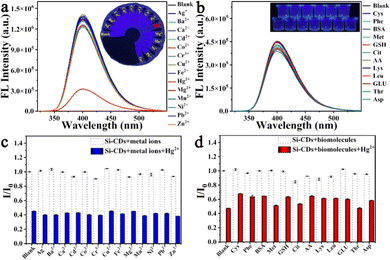 |
| Fig. 2 (a) Fluorescence spectrum response curves of Si-CDs with different metal ions; inset: corresponding images under a UV lamp. (b) Fluorescence spectrum response curves of Si-CDs after adding different biomolecules; inset: corresponding images showing the fluorescence color change under a UV lamp. (c) Histogram of the fluorescence intensity ratios (I/I0) of the Si-CDs with various metal ions without (black bar) and with Hg2+ (blue bar). (d) Histogram of the fluorescence intensity ratios (I/I0) of the Si-CDs with various biomolecules without (black bar) and with Hg2+ (red bar). | |
3.6 Sensitive assay for Hg2+
The sensitivity of the response of the Si-CDs to Hg2+ was evaluated by adding different concentrations of Hg2+ under the optimized conditions. As indicated in Fig. 3(a), the fluorescence response of the Si-CDs at the peak at around 400 nm decreased systematically with gradual increase of the Hg2+ concentration. The linear relationship could be expressed as I0/I = 0.2318x + 1.0283 over a linear range of 0.008 μM to 0.4 μM (R2 = 0.998), where I0 and I are the emission intensity before and after the addition of Hg2+ under 300 nm excitation, respectively, and the lowest detectable concentration of Hg2+ was calculated to be 33 nM on the basis of 3δ/k.57 Simultaneously, Fig. 3(c) shows that as the Hg2+ concentration was increased, the blue brightness of the Si-CDs under a UV lamp was also gradually extinguished, confirming the sensitivity of the Si-CDs toward Hg2+. The above results illustrate that the Si-CDs could be utilized for quantitative analysis of Hg2+ with good performance. For evaluation of the detection performance of the Si-CDs, a comparison with different reported sensors is presented in Table S1 (ESI†).58–64 The results illustrate that the Si-CDs had good performance comparable to or even better than that of the reported sensors.
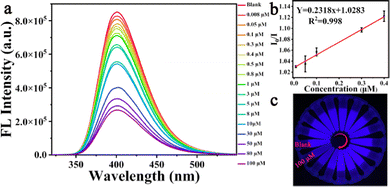 |
| Fig. 3 (a) Fluorescence spectra of the Si-CDs in the presence of different concentrations of Hg2+. (b) Linear relationship between I0/I and the Hg2+ concentration (0.008–0.4 μM). (c) Fluorescence images of the Si-CDs with Hg2+ under a UV lamp. | |
3.7 Analysis of Hg2+ in real samples
To further evaluate the feasibility of the application of the Si-CD sensing platform, the detection of Hg2+ in real water samples was carried out by spiking various concentrations of Hg2+ into natural water samples. The real water samples, which included tap water, Aershan water and sea water, were collected and tested using a standard addition method, and the detection results are presented in Table 1. Satisfactory recovery of Hg2+ in the range of 96.2–99.5% was obtained, with a relative standard deviation (RSD) of around 2.7–4.3%. The excellent analytical performance of Hg2+ demonstrated the reliability and applicability of the Si-CD sensing platform for the detection of Hg2+ in actual water samples.
Table 1 Analytical results for Hg2+ detection in real samples
Sample |
Ion |
Added (μM) |
Detected (μM) |
Recovery (%) |
RSD (%) |
Sea water |
Hg2+ |
0.1 |
0.099 |
99.5 |
2.9 |
0.12 |
0.112 |
99.1 |
2.7 |
Tap water |
Hg2+ |
0.2 |
0.192 |
96.2 |
3.3 |
0.3 |
0.296 |
98.7 |
3.2 |
Aershan water |
Hg2+ |
0.28 |
0.272 |
97.2 |
4.3 |
0.3 |
0.296 |
98.6 |
3.0 |
3.8 Possible sensing mechanism of Hg2+ by Si-CDs
To explore the possible sensing mechanism of the Si-CDs for Hg2+, a large number of characterization methods were carried out. As shown in Fig. 1(c), the FTIR spectra of the Si-CDs were characterized before and after introducing Hg2+. In the presence of Hg2+, the FTIR absorption peaks of the Si-CDs did not shift or disappear significantly, indicating that the structure of the Si-CDs was not destroyed.65 In general, the Stern–Volmer equation can be used as a common method to deduce the quenching principle to be static or dynamic quenching.66 The quenching constant (KSV) was derived from Stern–Volmer equation, F0/F = 1 + KSV[Q] = 1+ Kqτ0[Q], where F0 and F are the fluorescence intensity of the probe in the absence and presence of Hg2+, respectively. KSV is the Stern–Volmer constant, which is equal to the product of the quenching rate constant (Kq) and the lifetime of the Si-CDs without Hg2+ (τ0). [Q] represents the concentration of the Hg2+.67 As illustrated in Fig. 4(b), the value of KSV was found to be 231.8 nM−1, demonstrating either a static or a dynamic mechanism.68 To distinguish the quenching effect involved in the fluorescence quenching process in detail, the fluorescence lifetimes of the Si-CDs and Si-CDs + Hg2+ were measured (Fig. 4(a) and (b)). The results revealed that the average fluorescence lifetimes of the Si-CDs and Si-CDs + Hg2+ were calculated to be 3.453 μs and 2.520 μs, with the dramatic reduction in the lifetime indicating the occurrence of a dynamic quenching effect (DQE) process between the probe Si-CDs and Hg2+.69 Additionally, the UV-vis absorption spectra of the Si-CDs, Hg2+ and Si-CDs + Hg2+ were further obtained to verify the quenching mechanism. As shown in Fig. 4(c), upon the addition of Hg2+ to the Si-CDs system, no new peak appeared compared to the samples of Hg2+ and Si-CDs, indicating that a static quenching effect (SQE) mechanism could be excluded in the detection system, which was consistent with the above conclusion based on fluorescence lifetime.70 Further, other possible quenching mechanisms should also be proposed to illustrate the mechanism in detail. Evident spectral overlap occurred in the absorption band of the Hg2+ and the excitation spectrum of the Si-CDs, indicating that the fluorescence quenching of the Si-CDs by Hg2+ may originate from the internal filtering effect (IFE).71 However, the remarkable reduction in the lifetime demonstrated that the IFE mechanism can be excluded in the process.72 TEM characterization was performed to further reveal the mechanism. As illustrated in Fig. 4(e) and (f), comparing the Si-CDs with and without Hg2+, the TEM of the Si-CDs showed an obvious agglomeration state, indicating that the fluorescence quenching of the Si-CDs could be ascribed to aggregation-caused quenching (ACQ).73
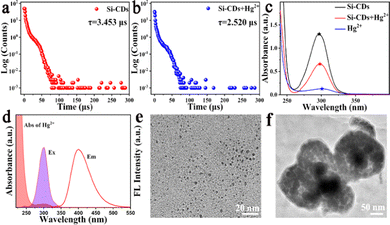 |
| Fig. 4 (a) Fluorescence lifetime of the Si-CDs. (b) Fluorescence lifetime of the Si-CDs with Hg2+. (c) UV-vis absorption spectra of Si-CDs, Si-CDs + Hg2+ and Hg2+. (d) UV-vis absorption of Hg2+ and fluorescence excitation and emission spectra of the Si-CDs. TEM images of the Si-CDs in the (e) absence and (f) presence of 100 μM Hg2+. | |
3.9 Fingerprint detection
The excellent fluorescence properties of the Si-CDs and the feasible collection of their powder inspired us to explore their utility in the visualization of latent fingerprints. Latent fingerprints are most common at crime scenes. They are not visible to the naked eye, and the visualization of latent fingerprints is of great significance to both medical and forensic research, as the residues in the fingerprints conceal unique individual information.74 Thus, the development of latent fingerprints is crucial to identifying individuals in forensic sciences. To demonstrate the widespread application of Si-CDs in fingerprint imaging, several typical substrates (copper sheet, glass sheet, silicon wafer, tinfoil) were selected for testing. As shown in Fig. 5, the obtained Si-CD powder was deposited on different substrates, and it was observed that the grain of fingerprint was clearly observed with smooth and sufficient details under a UV lamp, even though different substrate background colors easily interfered with the blue luminescence. Furthermore, the second-level details ((1) whorl, (2) delta, (3) termination, (4) bifurcation, (5) intersect, (6) short ridge, (7) island and (8) lake) could be also clearly recognized and distinguished by the naked eye. These unique feature points in the second level endowed the fingerprint with uniqueness and invariance, as the second-level details are usually confirmatory signatures for the identification of individuals.75 The above results verified that Si-CDs could obtain powerful results in the visualization of fingerprints to exhibit potential and practical application in crime scenes.
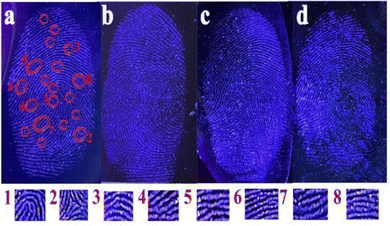 |
| Fig. 5 Images of latent fingerprints developed using the Si-CDs on a (a) copper sheet, (b) glass sheet, (c) silicon wafer, and (d) tinfoil. Details marked in (a), that is, (1) whorl, (2) delta, (3) termination, (4) bifurcation, (5) intersect, (6) short ridge, (7) island and (8) lake. | |
4 Conclusions
In summary, innovative bifunctional Si-CDs with good aqueous solubility and excellent optical properties were successfully designed and synthesized via a one-step synthesis method. The Si-CDs possessed the advantages of high sensitivity and good selectivity in the detection of Hg2+ with a limit of detection of 33 nM. The detection of Hg2+ in water samples by Si-CDs exhibited satisfactory recovery, confirming their good practicability. Moreover, the developed Si-CD powder can be employed to visualize latent fingerprints on different substrates with clear grains. The developed fingerprints exhibited high definition and contrast with second-level individual characteristics. These results indicated that Si-CDs will deliver significant performance as a sensor in the research applications of the detection heavy metals in actual water samples and visualization of fingerprints.
Author contributions
Tingting Li: conceptualization, investigation, data curation, writing-original draft, and writing-review & editing. Yuxue Ning: conceptualization, investigation, and data curation. Jingyu Pang: resources, software, and formal analysis. Lihua Chen: funding acquisition, and supervision. Fang Zhang: funding acquisition, supervision, and validation. Fang Chai: supervision, writing-review & editing, project administration, and funding acquisition.
Conflicts of interest
There are no conflicts to declare.
Acknowledgements
This work was financially supported by the National Natural Science Foundation of China (22171060) and China Scholarship Council (No. 201908230064), Graduate Innovation Fund of Harbin Normal University (HSDSSCX2022-14), The open project of the Chemistry Department of Qingdao University of Science and Technology (QUSTHX202002).
Notes and references
- C. Lai, S. Y. Liu, C. Zhang, G. M. Zeng, D. L. Huang, L. Qin, X. G. Liu, H. Yi, R. Z. Wang, F. L. Huang, B. S. Li and T. Y. Hu, ACS Sens., 2018, 3, 2566–2573 CrossRef CAS PubMed.
- L. B. Li, J. Y. Zhang, W. L. Zhao, X. H. Liu, L. J. Luo, X. Y. Bi, L. Cheng and T. Y. You, ACS Appl. Nano Mater., 2021, 4, 1009–1018 CrossRef CAS.
- P. A. Kobielska, A. J. Howarth, O. K. Farha and S. Nayak, Coord. Chem. Rev., 2018, 358, 92–107 CrossRef CAS.
- C. C. Long, X. Li, Z. X. Jiang, P. Zhang, Z. H. Qing, T. P. Qing and B. Feng, J. Hazard. Mater., 2021, 413, 125470 CrossRef CAS PubMed.
- J. H. Lin, S. J. Chen, J. N. Lee, W. Y. Chu, C. J. Yu, C. C. Chang and C. F. Chen, Chem. Eng. J., 2022, 430, 133070 CrossRef CAS.
- Z. L. Wang, Y. Zhang, J. Yin, Y. Q. Yang, H. Luo, J. Song, X. Xu and S. F. Wang, ACS Sustainable Chem. Eng., 2020, 8, 12348–12359 CrossRef CAS.
- S. L. C. Ferreira, M. A. Bezerra, A. S. Santos, W. N. L. dos Santos, C. G. Novaes, O. M. C. de Oliveira, M. L. Oliveira and R. L. Garcia, TrAC, Trends Anal. Chem., 2018, 100, 1–6 CrossRef CAS.
- M. Shirani, F. Salari, S. Habibollahi and A. Akbari, Microchem. J., 2020, 152, 104340 CrossRef CAS.
- R. Tabaraki and O. Abdi, J. Mol. Liq., 2018, 251, 77–82 CrossRef CAS.
- A. Giacomino, A. R. Redda, S. Squadrone, M. Rizzi, M. C. Abete, C. L. Gioia, R. Toniolo, O. Abollino and M. Malandrino, Food Chem., 2017, 221, 737–745 CrossRef CAS PubMed.
- D. E. León-Pérez, A. M. Muñoz-Jiménez and C. Jiménez-Cartagena, Anal. Methods, 2015, 8, 2383–2391 CrossRef.
- A. K. Singh, V. K. Singh, M. Singh, P. Singh, S. R. Khadim, U. Singh, B. Koch, S. H. Hasan and R. K. Asthana, J. Photochem. Photobiol., A, 2019, 376, 63–72 CrossRef CAS.
- L. L. Peng, M. H. Yang, M. Zhang and M. Y. Jia, Food Chem., 2022, 392, 133265 CrossRef CAS.
- R. Y. Xie, Y. Y. Qu, M. Y. Tang, J. Q. Zhao, S. Chua, T. T. Li, F. Zhang, A. E. H. Wheatley and F. Chai, Food Chem., 2021, 364, 130366 CrossRef CAS PubMed.
- B. A. Kairdolf, A. M. Smith, T. H. Stokes, M. D. Wang, A. N. Young and S. Nie, Annu. Rev. Anal. Chem., 2013, 6, 143–162 CrossRef CAS.
- J. L. Yang, N. Z. Song and Q. Jia, Nanoscale, 2019, 11, 21927 RSC.
- Y. H. Liu, H. Huang, W. J. Cao, B. D. Mao, Y. Liu and Z. H. Kang, Mater. Chem. Front., 2020, 4, 1586–1613 RSC.
- Z. J. Ye, X. Geng, L. Wei, Z. H. Li, S. Lin and L. H. Xiao, ACS Nano, 2021, 15, 934–943 CrossRef CAS.
- Y. L. Xu, Y. Yang, S. Lin and L. H. Xiao, Anal. Chem., 2020, 92, 15632–15638 CrossRef CAS PubMed.
- Z. J. Ye, L. Wei, X. Geng, X. Wang, Z. H. Li and L. H. Xiao, ACS Nano, 2019, 13, 11593–11602 CrossRef CAS PubMed.
- F. J. Bai, H. W. Wang, L. Y. Lin and L. S. Zhao, New J. Chem., 2022, 46, 1972–1983 RSC.
- Y. J. Chung, J. Kim and C. B. Park, ACS Nano, 2020, 14, 6470–6497 CrossRef CAS PubMed.
- Y. S. Wu, D. M. Qin, Z. Luo, S. Meng, G. C. Mo, X. H. Jiang and B. Y. Deng, ACS Sustainable Chem. Eng., 2022, 10, 5195–5202 CrossRef CAS.
- V. Sharma, P. Tiwari, N. Kaur and S. M. Mobin, Environ. Chem. Lett., 2021, 19, 3229–3241 CrossRef CAS.
- A. L. Meng, Y. Zhang, X. H. Wang, Q. H. Xu, Z. J. Li, L. Y. Sheng and L. J. Yan, Colloids Surf., A, 2022, 648, 129150 CrossRef CAS.
- Y. L. Liu, P. H. Zhou, Y. L. Wu, X. Y. Su, H. J. Liu, G. F. Zhu and Q. X. Zhou, Sci. Total Environ., 2022, 827, 154357 CrossRef CAS PubMed.
- B. Y. Wang, H. J. Cai, G. I. N. Waterhouse, X. L. Qu, B. Yang and S. Y. Lu, Small Sci., 2022, 2200012 CrossRef CAS.
- D. Zhao, W. T. Ma and X. C. Xiao, Nanomaterials, 2018, 8, 612 CrossRef PubMed.
- A. Kathiravan, A. Gowri, V. Srinivasan, T. A. Smith, M. Ashokkumar and M. A. Jhonsi, Analyst, 2020, 145, 4532–4539 RSC.
- I. Milenkovic, M. Algarra, C. Alcoholado, M. Cifuentes, J. M. Lázaro-Martínez, E. Rodríguez-Castellón, D. Mutavdžić, K. Radotić and T. J. Bandosz, Carbon, 2019, 144, 791–797 CrossRef CAS.
- A. Shabashini, S. K. Panja and G. C. Nandi, Chem. – Asian J., 2021, 16, 1057–1072 CrossRef CAS PubMed.
- D. Fernandes, M. J. Krysmann and A. Kelarakis, Chem. Commun., 2015, 51, 4902–4905 RSC.
- X. Y. Dong, X. Q. Niu, Z. Y. Zhang, J. S. Wei and H. M. Xiong, ACS Appl. Mater. Interfaces, 2020, 12, 29549–29555 CAS.
- Z. S. Qian, X. Y. Shan, L. J. Chai, J. J. Ma, J. R. Chen and H. Feng, ACS Appl. Mater. Interfaces, 2014, 6, 6797–6805 CrossRef CAS.
- J. Zhou, H. Zhou, J. B. Tang, S. Deng, F. Yan, W. J. Li and M. H. Qu, Microchim. Acta, 2017, 184, 343–368 CrossRef CAS.
- B. Y. Zhu, G. J. Ren, M. Y. Tang, F. Chai, F. Y. Qu, C. G. Wang and Z. M. Su, Dyes Pigm., 2018, 149, 686–695 CrossRef CAS.
- J. Y. Pang, R. Y. Xie, S. Chua, Y. Zou, M. Y. Tang, F. Zhang and F. Chai, Spectrochim. Acta, Part A, 2021, 261, 120035 CrossRef CAS PubMed.
- J. Y. Chen, W. R. Liu, Y. J. Li, X. K. Zou, W. Li, J. R. Liang, H. R. Zhang, Y. L. Liu, X. J. Zhang, C. F. Hu and B. F. Lei, Chem. Eng. J., 2022, 428, 131168 CrossRef CAS.
- V. D. Dang, A. B. Ganganboina and R. A. Doong, ACS Appl. Mater. Interfaces, 2020, 12, 32247–32258 CrossRef CAS.
- H. L. Ye, Y. Shang, H. Y. Wang, Y. L. Ma, X. W. He, W. Y. Li, Y. H. Li and Y. K. Zhang, Talanta, 2021, 230, 122294 CrossRef CAS PubMed.
- C. J. Pan, Q. Q. Wen, L. F. Ma, X. Z. Qin and S. X. Feng, New J. Chem., 2021, 45, 12528–12537 RSC.
- M. Bhatt, S. Bhatt, G. Vyas, I. H. Raval, S. Haldar and P. Paul, ACS Appl. Nano Mater., 2020, 3, 7096–7104 CrossRef CAS.
- S. Rajendran, D. V. Ramanaiah, S. Kundu and S. K. Bhunia, ACS Appl. Nano Mater., 2021, 4, 10931–10942 CrossRef CAS.
- Q. L. Zhao, Y. Wang, M. Q. Zhang, D. H. Wu, J. Sun and X. R. Yang, Biosens. Bioelectron., 2022, 214, 114504 CrossRef CAS PubMed.
- Y. G. Ma, H. Mei, Y. Y. Li, P. P. Zhou, G. Y. Mao, H. L. Wang and X. D. Wang, Food Chem., 2022, 379, 132155 CrossRef CAS PubMed.
- M. H. Zan, C. Li, D. M. Zhu, L. Rao, Q. F. Meng, B. Chen, W. Xie, X. W. Qie, L. Li, X. J. Zeng, Y. R. Li, W. F. Dong and W. Liu, J. Mater. Chem. B, 2020, 8, 919–927 RSC.
- W. J. Wang, J. M. Xia, J. Feng, M. Q. He, M. L. Chen and J. H. Wang, J. Mater. Chem. B, 2016, 4, 7130–7137 RSC.
- J. Y. Liu, J. B. Zhang, Y. Zhang, Y. Wang, M. K. Wang, Z. W. Li, G. N. Wang and X. G. Su, Talanta, 2022, 237, 122956 CrossRef CAS PubMed.
- C. X. Chen, D. Zhao, B. Wang, P. J. Ni, Y. Y. Jiang, C. H. Zhang, F. Yang, Y. Z. Lu and J. Sun, Anal. Chem., 2020, 92, 4639–4646 CrossRef CAS.
- L. Li, L. H. Shi, J. Jia, O. Eltayeb, W. J. Lu, Y. H. Tang, C. Dong and S. M. Shuang, Sens. Actuators, B, 2021, 332, 129513 CrossRef CAS.
- Q. Q. Wen, C. J. Pan, X. Z. Qin, Q. J. Ma and S. X. Feng, Anal. Methods, 2021, 13, 390–398 RSC.
- H. Huang, C. G. Li, S. J. Zhu, H. L. Wang, C. L. Chen, Z. R. Wang, T. Y. Bai, Z. Shi and S. H. Feng, Langmuir, 2014, 30, 13542–13548 CrossRef CAS.
- T. Y. Wang, C. Y. Chen, C. M. Wang, Y. Z. Tan and W. S. Liao, ACS Sens., 2017, 2, 354–363 CrossRef CAS PubMed.
- Q. Y. Li, Y. Q. Wang, M. Jiang, Y. Y. Cui, X. Yu and L. Xu, Food Chem., 2022, 366, 130629 CrossRef CAS PubMed.
- M. Y. Tang, G. J. Ren, B. Y. Zhu, L. Y. Yu, X. D. Liu, F. Chai, H. B. Wu and C. G. Wang, Anal. Methods, 2019, 11, 2072–2081 RSC.
- H. Liu, X. Geng, X. Wang, L. Wei, Z. H. Li, S. Lin and L. H. Xiao, CCS Chem., 2021, 3, 3081–3093 Search PubMed.
- P. F. Fan, C. Liu, C. C. Hu, F. F. Li, X. Lin, F. B. Xiao, H. Liang, L. Li and S. Y. Yang, New J. Chem., 2022, 46, 877–882 RSC.
- S. Pattnayak, U. Sahoo, S. Choudhury and G. Hota, Colloids Surf., A, 2022, 648, 129377 CrossRef CAS.
- Y. Xu, H. Y. Li, B. Wang, H. C. Liu, L. Zhao, T. Y. Zhou, M. T. Liu, N. Huang, Y. Li, L. Ding and Y. H. Chen, Microchim. Acta, 2018, 185, 252 CrossRef PubMed.
- H. Huang, Y. H. Weng, L. H. Zheng, B. X. Yao, W. Weng and X. C. Lin, J. Colloid Interface Sci., 2017, 506, 373–378 CrossRef CAS PubMed.
- M. Zhang, W. T. Wang, P. Yuan, C. Chi, J. Zhang and N. L. Zhou, Chem. Eng. J., 2017, 330, 1137–1147 CrossRef CAS.
- Y. Wang, P. D. Mao, W. N. Wu, X. J. Mao, Y. C. Fan, X. L. Zhao, Z. Q. Xu and Z. H. Xu, Sens. Actuators, B, 2018, 255, 3085–3092 CrossRef CAS.
- E. Bozkurt and H. I. Gul, Sens. Actuators, B, 2018, 255, 814–825 CrossRef CAS.
- J. Zhang and S. H. Yu, Nanoscale, 2014, 6, 4096–4101 RSC.
- B. Y. Zhu, M. Y. Tang, L. Y. Yu, Y. Y. Qu, F. Chai, L. H. Chen and H. B. Wu, Anal. Methods, 2019, 11, 3570–3577 RSC.
- C. J. Pan, Q. Q. Wen, L. F. Ma, X. Z. Qin and S. X. Feng, New J. Chem., 2022, 46, 12729–12738 RSC.
- X. P. Yang, F. M. Tian, S. H. Wen, H. Xu, L. Zhang and J. Zeng, Processes, 2021, 9, 170 CrossRef CAS.
- W. B. Lu, X. Y. Qin, S. Liu, G. H. Chang, Y. W. Zhang, Y. L. Luo, A. M. Asiri, A. O. Al-Youbi and X. P. Sun, Anal. Chem., 2012, 84, 5351–5357 CrossRef CAS.
- Y. Yan, J. H. Liu, R. S. Li, Y. F. Li, C. Z. Huang and S. J. Zhen, Anal. Chim. Acta, 2019, 1063, 144–151 CrossRef CAS PubMed.
- L. N. Zhang, Y. R. Xu, J. Xu, H. J. Zhang, T. Q. Zhao and L. Jia, J. Hazard. Mater., 2022, 430, 128478 CrossRef CAS.
- X. H. Peng, Y. Wang, Q. Y. Wang, J. J. Tang, M. S. Zhang and X. P. Yang, Spectrochim. Acta, Part A, 2022, 279, 121454 CrossRef CAS.
- X. C. Li, Y. Z. Fu, S. J. Zhao, J. F. Xiao, M. H. Lan, B. H. Wang, K. Zhang, X. Z. Song and L. T. Zeng, Chem. Eng. J., 2022, 430, 133101 CrossRef CAS.
- X. C. Li, S. J. Zhao, B. L. Li, K. Yang, M. H. Lan and L. T. Zeng, Coord. Chem. Rev., 2021, 431, 213686 CrossRef CAS.
- R. Bahadur, M. K. Kumawat, M. Thakur and R. Srivastava, J. Lumin., 2019, 208, 428–436 CrossRef CAS.
- H. J. Wang, W. Y. Hou, J. Kang, X. Y. Zhai, H. L. Chen, Y. W. Hao and G. Y. Wan, Dalton Trans., 2021, 50, 12188–12196 RSC.
|
This journal is © The Royal Society of Chemistry and the Centre National de la Recherche Scientifique 2023 |