DOI:
10.1039/D3NH00137G
(Communication)
Nanoscale Horiz., 2023,
8, 1568-1576
Automated mechanical exfoliation technique: a spin pumping study in YIG/TMD heterostructures†
Received
10th April 2023
, Accepted 23rd August 2023
First published on 1st September 2023
Abstract
Spintronics devices rely on the generation and manipulation of spin currents. Two-dimensional transition-metal dichalcogenides (TMDs) are among the most promising materials for a spin current generation due to a lack of inversion symmetry at the interface with the magnetic material. Here, we report on the fabrication of Yttrium Iron Garnet(YIG)/TMD heterostructures by means of a crude and fast method. While the magnetic insulator single-crystalline YIG thin films were grown by magnetron sputtering, the TMDs, namely MoS2 and MoSe2, were directly deposited onto YIG films using an automated mechanical abrasion method. Despite the brute force aspect of the method, it produces high-quality interfaces, which are suitable for spintronic device applications. The spin current density and the effective spin mixing conductance were measured by ferromagnetic resonance, whose values found are among the highest reported in the literature. Our method can be scaled to produce ferromagnetic materials/TMD heterostructures on a large scale, further advancing their potential for practical applications.
New concepts
In order to advance the field of nanotechnology and its potential industrial applications, it is crucial to have a simple and efficient method for producing layered materials on a large scale. We report on a simple, affordable, and scalable method to produce transition-metal dichalcogenides (TMDs) thin films with a few layers thick onto Yttrium Iron Garnet (YIG) films based on an automated mechanical abrasion method. This room-temperature method allows the deposition of TMDs thin films with excellent uniformity in a large area and different types of substrates. The effectiveness of this technology was demonstrated by producing practical spintronics devices consisting of high-quality interface YIG/TMD heterostructures and investigating the spin current injection from the magnetic insulator YIG into Mo-based TMDs. The spin current was evaluated by ferromagnetic resonance spin pumping driven by the inverse Rashba–Edelstein effect, which originated from the loss of inversion symmetry breaking at the TMD/YIG interface. The innovative, cost-effective nature of this TMD film growth process paves the way for new areas of research and application involving 2D materials.
|
1 Introduction
Transition-metal dichalcogenides (TMDs) have been studied for a long time in their bulk form.1,2 Over the past few years, layered TMDs have gained increasing importance due to their electronic band structures, showing unique electrical, mechanical, and optical properties and becoming one of the most studied materials. Semiconducting TMDs materials (e.g., MoS2, MoSe2, and WS2) have emerged as promising candidates for nano-electronic, optoelectronics, and spintronic applications.3–10 For instance, the sharp and well-defined interfaces of TMDs heterojunctions have positioned them as compelling contenders for spin tunneling layers in magnetic tunnel junctions, offering distinct advantages over conventional three-dimensional ferromagnetic metals and oxides.11–13
The most promising methods to prepare low-dimensional TMDs are chemical vapor deposition (CVD) and mechanical exfoliation from bulk single crystals.14–22 The CVD is a synthesis method where monolayers are sequentially grown on a substrate, usually quartz, silicon, or silicon oxide.23–25 These CVD-grown TMDs have smaller grain sizes, a few microns large, typically in a triangular shape. Still, the grains have several internal boundaries, which promotes an oxidation process. The degradation is accelerated by humidity and UV irradiation.26 Another drawback of this method is that it requires high temperature for growth. Despite efforts to reduce the temperature,27 the high values can still compromise the structure and properties of the substrate, especially if it is magnetic. An alternative solution is to grow over a suitable substrate and then transfer the TMD to the desired substrate.28 Unfortunately, the transfer process can damage the TMDs and introduce defects and impurities, resulting in a low-quality interface.29–32
Furthermore, the initial investment in the CVD method is expensive and often requires catalysts, introducing unnecessary contamination.33–35 In addition, the growth process also emits several hazardous exhaust gas, such as arsine and phosphine.36,37 Alternatively, mechanical exfoliation of bulk single crystals avoids the temperature and interface issues, but it is very time-consuming and not scalable for industrial production.38,39 Therefore, the development of the deposition method for the TMDs, layer control, and process scalability is of great interest to both basic science and industrial applications.
Recently, it was discovered that some TMDs have a high potential for spin-to-charge conversion.40–42 Due to the layered structure of TMDs, an inversion symmetry breaking leads to spin splitting and considerable spin-momentum locking that give rise to the Rashba–Edelstein effect (REE).43–45 Several studies show that TMDs are more suitable for spin-to-charge conversion than the commonly studied 3-dimensional materials with high spin–orbit coupling. For instance, Zhang et al.46 observed a current-induced spin transfer torque in Py/MoS2. Shao et al.40 reported a spin–orbit torque generated by the REE in MoS2/CoFeB, and Bangar et al.47 studied the spin–orbit coupling dependency on the spin-to-charge conversion in Py/TMDs.
For spintronics device applications, the TMDs must have a few layers and a good interface, especially when electric or spin current injection is involved, such as in spin pumping and spin transfer torque experiments.48–51 The spin pumping is one of the most widely used effects to probe the material's potential for spin-to-charge conversion and highly depends on the interface quality. It occurs when the magnetization precession of an adjacent layer injects angular momentum into the adjacent material. This injection acts as a new dissipative channel and enhances the Gilbert damping (dissipation) of the ferromagnetic material.52–54
Among the several materials to perform the spin pumping, the ferrimagnetic yttrium iron garnet (Y3Fe5O12/YIG) emerges as one of the best alternatives, mainly due to its very low Gilbert damping and electrical insulator behavior that allows a pure spin current generation avoiding issues associated with charge currents.55–63 Hence, the heterostructures comprising the stacking of three-dimensional ferrimagnetic insulator layers and two-dimensional materials stand out as highly promising candidates for feasible devices.64 It is worth noting that for YIG to exhibit these desirable characteristics, it needs to be carefully grown monocrystalline and in the direction (111).65 In addition, after preparation, when heated, these characteristics are lost. For this reason, growing TMD directly on YIG, by CVD, for example, is not the best alternative.
Herein, we report on a large spin pumping, spin mixing conductance, and spin current density from a sputtered YIG film to a few layers of MoS2 and MoSe2. The TMDs were grown directly on the YIG film by an automated method based on mechanical abrasion.66 It is interesting to remark that the technique allows the preparation of large surface coating, larger than 5 cm2, of oriented TMD thin layers (≈5 nm) without inhomogeneities. Despite the brute force aspect of the mechanical abrasion, the method is fast and efficient, providing a high-quality YIG/TMD interface. Still, this technique avoids high temperatures and preserves the crystal quality of the YIG film. The spin pumping, spin mixing conductance, and YIG's magnetic properties were obtained through ferromagnetic resonance. High-resolution transmission electron microscopy (TEM) was performed to confirm the YIG/TMD interface quality and TMDs layered structure. X-ray diffraction (XRD) and Raman spectroscopy were used to probe the TMDs and YIG crystallinity. It is relevant to highlight that both YIG's sputtering growth and the automated mechanical abrasion method are free of hazardous exhaust gas and mass-scalable, practical, and low-priced production.
2 Results and discussion
Fig. 1 shows a scheme of a TMD heterostructure deposited on a YIG film. The YIG thin film was grown by RF magnetron sputtering; it is 50 nm thick and 5 × 5 mm2 large. It was grown on gadolinium gallium garnet single-crystal substrate oriented in the (111) direction. Ex situ annealing followed by a growth procedure was performed to ensure YIG crystallization in the direction of the substrate, which is confirmed by the presence of (111) family peaks in the X-ray pattern, see Fig. 2(a). Our YIG films exhibit very low damping (α ≈ 5 × 10−4) and a very smooth surface with root mean square surface roughness of 0.23 nm, measured by atomic force microscopy. These properties are essential for good interfacial quality and, therefore, the measure of the spin pumping effect. More details about the YIG growth and annealing can be found elsewhere.65
 |
| Fig. 1 Schematic representation of the deposition system and the lithographed YIG/TMD heterostructure. | |
 |
| Fig. 2 (a) shows the X-ray diffraction of the YIG/GGG substrate. The MoS2/MoSe2 Raman measurements are shown in (b) and (d), and the X-ray diffraction pattern is in (c) and (e). Red/green represents the thin layers TMDs film, and black is the powder precursor. | |
TMDs with a few thick monolayers were deposited on YIG films by means of an automated technique based on mechanical abrasion.66 Basically, we use a soft polymer, namely polydimethylsiloxane (PDMS), to cover a tip, which is coupled to a computer numerical control (CNC). The deposition pad is then pressed into a TMD powder precursor. The pad-CNC acts as an XY writing pad, making mechanical exfoliation and leaving TMD by the path, providing the deposition. To achieve an excellent level of uniformity, the CNC is coupled to a piezoelectric sensor, where the z coordinate is controlled. This technique is affordable, fast, and low-cost, allowing the deposition of TMD thin films in large-area substrates without damaging the substrate surface. The control of film characteristics is obtained through the control of the parameters of the deposition system. The thickness of the film is influenced by the scanning speed of the deposition pad, and primarily by the number of exfoliations of the material on the surface of the substrate. Consequently, this approach made it possible to obtain a certain thickness by adjusting the number of exfoliations. The roughness and film homogeneity control are achieved by adjusting the pressure exerted by the deposition pad on the substrate, highlighting the significance of the piezoelectric component. This capability allows for producing films with low roughness, which is desirable for studying interfacial effects. Nevertheless, certain technological applications benefit from the use of rough films. This abrasion process occurs easily due to the van der Waals structure of the TMDs.
One of the most important features of the technique is the possibility of depositing films over large areas with excellent uniformity and a high-quality interface. The results of large spin pumping mean an excellent YIG/TMDs interface quality. Additionally, the XRD, TEM, and Raman analyses show the excellent crystalline structure quality of both TMD film and the YIG/TMD interface.
From a more general point of view, the present method enables the deposition of continuous thin film on different types of substrates (flexible or non-flexible). The process is easily adapted to virtually any TMD, on large or small areas of the substrate to be coated, and to the desired thickness or number of TMDs layers. Moreover, this process prevents the production of harmful gases, resulting in a safer production. Details concerning parameters, different substrate types, reproducibility, interface quality, thickness, and can be found in ESI.†
Raman spectroscopy is sensitive to the vibrational modes and, therefore, gives information on the crystalline and electronic structures. Specifically, for the TMDs, it is well known that the vibrational modes are highly sensitive to thickness due to van der Waals bonds between the layers.67,68 Hence, in order to identify the properties of the TMD that were directly deposited on YIG, we conducted a Raman study in the TMDs under investigation, namely MoS2 and MoSe2. Fig. 2(b) and (d) show representative Raman spectra obtained from the MoS2 (red), MoSe2 (green), and for the powder precursor in black. One can notice that for the MoS2 (in Fig. 2(b)), the in-plane phonon mode
was recorded at 384.8 cm−1, while the out-of-plane
mode is at 409.9 cm−1 for the few layers of MoS2 film. Compared to the powder precursor, it has a 2.8 cm−1 shift in the
mode and 2.1 cm−1 for the
mode. The difference between the peak positions is 25.1 cm−1, indicating that the film has approximately 6 layers.69 In addition, the full width at the half-maximum value of the E′ mode is 1.1 cm−1, indicating high crystallinity and no amorphous phase. Fig. 2(e) shows the representative Raman spectrum of a few layers of MoSe2 film. The peaks shows the out-of-plane
mode at 241 cm−1 and the in-plane
mode at 285.5 cm−1. These peaks are shifted 2.6 cm−1 for
and 5.2 cm−1 for
modes compared to the powder precursor. This result indicates that the thickness of MoSe2 was reduced and suggests that the film has more than 10 layers.70,71
In order to certify that the TMDs do not present changes in the structural phase or contamination, grazing incidence X-ray diffraction was performed in the powder precursor and in TMDs films. Fig. 2(c) and (e) show the XRD of MoS2 and MoSe2, which correspond to the hexagonal phase and to the crystallographic group P63/mmc.72–75 One can notice that some diffraction peaks present in the powder precursor (black) are not present in the films (red and green), and the peak family (001) presents higher intensity. This absence is expected due to the monocrystalline organization along the c-axis of the hexagon structure, meaning that the film is formed by layers of TMD coupled by van der Waals interaction.
Being the interface quality of utmost importance for observing the spin pumping effect, we focused on investigating the interface quality between YIG and TMDs by high-resolution transmission electron microscopy. Fig. 3(a) shows an image of the YIG/MoS2 interface obtained from transmission electron microscopy (TEM) in cross-section mode, which shows the MoS2 thickness of 6 to 8 layers. As can be seen from the inset, a high-resolution image does not present any defect, amorphization, or formation of oxides. This image clearly shows the ferromagnetic and the TMD in direct contact, which is suitable for a high spin current injection. Fig. 3(b) shows the high-resolution scanning transmission electron microscopy image from the YIG/MoSe2 interface. It is possible to observe that MoSe2 has between 12 and 16 layers. Also, the interface between YIG and MoSe2 presents a clean contact between the TMD and the YIG. It is important to highlight that the YIG stays monocrystalline and orientated along the (111) direction after the deposition, despite all the friction produced by the mechanical abrasion. The thicknesses of the TMDs obtained through the TEM images are in agreement with the ones obtained by the Raman spectra.
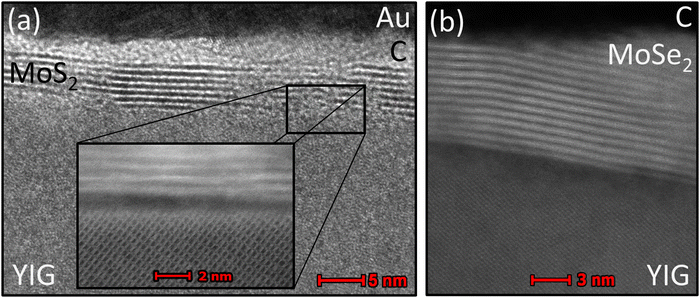 |
| Fig. 3 Transmission electron microscopy images of the YIG/MoS2 (a) and YIG/MoSe2 (b) interfaces. The inset shows the interface in higher resolution. | |
After the TMD deposition, it is important to verify whether YIG's magnetic properties were not compromised during the process because the magnetic properties of the YIG film are sensitive to structural and surface changes. To do so, we used ferromagnetic resonance (FMR) to measure the effective magnetization and the spin pumping. Fig. 4(a) shows the FMR signal recorded at 10 GHz for YIG/MoS2 in red, YIG/MoSe2 in green, and bare YIG in blue. The FMR data was obtained by applying several RF frequencies and sweeping the external magnetic field. Each sample was measured before and after the TMD deposition. Lorentzian derivative function fits the data, yielding the full-width at half maximum (ΔH, linewidth) and magnetic resonance field (Hr) parameters. From the frequency dependency of Hres, one can obtain the effective magnetization (4πMeff) by fitting Kittel's equation.53,54,65 As can be seen from Fig. 4(b), the MoS2 (red) and the MoSe2 (green) are almost perfectly coincident with the bare YIG data (blue). Therefore, the effective magnetization (see Table 1) remains unchanged after the deposition. This Meff suggests that the in-plane magnetization has not changed and indicates that the material's anisotropy has not changed.
 |
| Fig. 4 Ferromagnetic resonance and spin pumping measurements, the blue circles represent the bare YIG, the green lozenges the MoSe2, and the red squares the MoS2. (a) shows the field dependence of the derivative of radio-frequency absorption at 10 GHz fitted by a Lorentzian function. (b) shows the frequency dependence of the magnetic resonance field fitted by Kittel's equation. (c) shows the FMR linewidth in the function of the frequency and the linear adjust yields in the Gilbert damping. | |
Table 1 Magnetic and spin pumping properties. Values of effective magnetization (4πMeff), enhancement of the Gilbert damping (αSP), effective spin mixing conductance (g↑↓), and spin current density (js)
TMD |
4πMeff (G) |
α
SP × 10−4 |
g
↑↓ × 1018 m−2 |
j
s × 107 Am−2 |
MoS2 |
2358 |
5.9 ± 0.5 |
3.7 ± 0.3 |
8.6 ± 0.7 |
MoSe2 |
2238 |
4.8 ± 0.4 |
2.9 ± 0.3 |
18 ± 1 |
The second parameter obtained from the FRM is ΔH, closely related to the Gilbert damping (α) through the equation:53,54,65
|  | (1) |
where
ωRF is the frequency, Δ
H0 denotes film inhomogeneity line broadening, and |
γ| = 2.802 MHz Oe
−1 is the electron gyromagnetic ratio.
Despite the fact that TEM images show excellent crystalline and YIG/TMD interface quality, it probes a tiny region. On the other hand, spin pumping measures large regions. A high enough strength of spin pumping, i.e., when compared to YIG/Pt, means high-quality YIG/TMD interface and crystallinity, besides other contributions. The Gilbert damping (α) is obtained from the slope of the linear fit of the ΔH vs. ωRF data. As can be seen from Fig. 4(c), the slope of the YIG/MoS2 (red dots) and YIG/MoSe2 (green) is clearly higher when compared to the bare YIG (blue). This enhancement is expected in the spin pumping because the magnetization precession injects angular momentum into the adjacent material, here the TMD, providing an extra spin relaxation channel. The spin pumping is commonly defined by αsp = αeff − αYIG, where αeff is the YIG/TMD Gilbert damping and αYIG is the Gilbert damping of the same sample before the TMD deposition. Since the strength of the spin pumping depends on the Gilbert damping enhancement. For MoS2, the increase generated by the YIG spin pumping measured is (5.9 ± 0.5) × 10−4. For the YIG/MoSe2, the spin pumping found is (4.8 ± 0.4) × 10−4. For the sake of comparison, for YIG/Pt, the system with the highest αsp reported so far, one founds values close to ≈9 × 10−4.65 The factor 2 still classifies YIG/MoS2 and MoSe2 as good candidates for spintronic device applications. Furthermore, it is noteworthy that the spin pumping remains consistent even for thicker samples, as shown in Fig. S6 (ESI†) for MoS2 with a thickness of 27 nm. This observation reinforces the effectiveness of the deposition method in providing consistent coverage, not only for few-layer samples but also for thicker films.
Furthermore, to compare with other systems, this enhancement in the Gilbert damping is often reported as the spin current density (js) and the effective spin mixing conductance (g↑↓), both calculated by the spin pumping theory from the FMR measurements,76–80
|  | (2) |
where
γ is the gyromagnetic ratio,
ħ is the reduced Planck's constant,
e is the electronic charge, 4π
Meff is the effective saturation magnetization,
αeff is the effective Gilbert damping. And,
g↑↓ is the effective spin mixing conductance at the YIG/TMD interface, which is calculated through the equation:
|  | (3) |
where
tf is the ferromagnetic thickness,
geff is the effective Landé factor, and
μB is the Bohr magneton. The magnetic and spin pumping measured data are summarized in
Table 1.
The g↑↓ values obtained in this work for YIG/MoS2 and YIG/MoSe2 are close to the ones obtained for YIG/HM systems, where HM are 3D heavy metals such as Ag, Ta, W, Au, and Pt.81 However, a more accurate comparison between 2D and 3D systems should be made through the pseudo-spin Hall angle, which is inversely proportional to the thickness, and, therefore, favor the spin-to-charge conversion in 2D systems.82 Comparing with other YIG/2d-systems, as reported by Mendes et al., g↑↓ as 4 × 1017 m−2 for YIG/SLG (single-layered graphene),83 and 5 × 1017 m−2 for YIG/MoS2,84 these results are slightly smaller but still close to our findings.
Comparing TMDs coupled to conductor ferromagnetic layers (Py, Co, CoFeB, CoFeAl), like Py/TMD, for instance, the g↑↓ values reported by other groups47,85–88 are close to the ones shown in Table 1. However, due to the extremely low YIG's Gilbert damping, js is orders of magnitude higher.47,88 Also, js for our Mo-based TMDs are two orders of magnitude higher than pure Mo.89 When compared to other Rashba interfaces such as NiFe/Ag/(Bi and Sb)77,82 and NiFe/x/Bi2O3 (x = Ag, Au, Al, and Cu),78 our YIG/MoSe2 has a bit higher spin current density.
3 Conclusions
In summary, we have developed an automated mechanical abrasion method to deposit TMDs with a few layers directly onto YIG. We have fabricated YIG/MoS2 and YIG/MoSe2 heterostructures with good crystallinity and high-quality interfaces suitable for spintronic device applications. We characterized the spin current injection by ferromagnetic resonance spin pumping in the YIG/MoS2 with 6–8 MoS2 layers and YIG/MoSe2 with MoSe2 12–16 layers. Our measured effective spin mixing conductance of 3.7 × 1018 m−2 for the YIG/MoS2 and spin current density of 18 × 107 Am−2 for the YIG/MoSe2 are among the highest reported in the literature for TMDs and Rashba interfaces. The proposed fabrication method can be up-scalable, making it possible to produce these YIG/TMD without any safety concerns, and the TMD can virtually be of any type, providing a large potential for practical device applications in the field of spintronics.
4 Experimental section
4.1 Yttrium iron garnet growth
The Yttrium iron garnet (YIG) thin films were grown by magnetron sputtering on the top of a 5× 5 mm2 lithographed area of the Gadolinium Gallium Garnet (GGG) substrate, both oriented in the (111) easy-axis direction. The sputtering chamber was evacuated to 9.0 × 10−8 Torr, and the working argon pressure and flow were 10 mTorr and 15 sccm, respectively. The RF power was 75 W yielding a 0.42 Å s−1 deposition rate 10 cm distant from the target. After deposition, an ex situ annealing was performed with oxygen flow. More details about YIG annealing and lithographing can be found in our previous work.65
4.2 Automated mechanical abrasion method
The TMDs materials were deposited using a novel automatic technique of mechanical abrasion, which in addition to being accessible, fast, and low-cost like conventional exfoliation, also allows depositing films over large areas with excellent uniformity. A computer numerical control machine (CNC) coupled to a piezoelectric sensor was used to deposition TMDs layers. We used a CNC metallic cylindrical tip (designed for this purpose) coated with a viscoelastic polymer (polydimethylsiloxane-PDMS), which was then pressed into a bulk van der Waals (vdW) material powder. This procedure guarantees that the vdW micro-particles adhere to the polymer, which allows it to be used as a writing pad. The deposition process consists of 2 simple steps. The first PDMS pad is coated with 2 mg of vdW powder, then the material is rubbed onto the YIG film using CNC programming with constant pressure and the number of repetitions depending on the number of layers desired. The piezoelectric sensor has the function of controlling the pressure between the CNC pad and the substrate. By controlling the pressure and the number of repetitions in which the tip is rubbed against the surface of the substrate, it becomes possible to reproduce the characteristics of deposited films, such as thickness and uniformity. MoS2 (69860-100G), and MoSe2 (778087-5G) powders were purchased from Sigma-Aldrich, which have average grain sizes of the order of 6 μm and 44 μm, respectively. After loading the deposition pad with the vdW material powder, a constant pressure of 758 kPa was applied between the pad and the YIG surface. Subsequently, 100 exfoliation cycles were performed within a designated area of 5 × 5 mm2. The results of a reproducibility test conducted on five MoS2 samples can be found in ESI.†
4.3 Raman spectroscopy
The Raman measurements were performed by employing a Horiba LabRAM Evolution micro spectrometer at room temperature using a 532 nm laser line (∼1.07 mW).
4.4 X-Ray diffraction
The X-ray diffraction measurements were performed on a Panalytical X-ray diffractometer using Cu Kα1 from 30 to 130 degrees in a Bragg–Brentano configuration for the GGG/YIG and from 10 to 65 degrees in a grazing incidence for the TMDs.
4.5 Transmission electron microscopy
Before TEM analysis, the lamella was prepared from the bulk sample using a focused ion beam and scanning electron microscope (FIB-SEM). Since YIG and GGG are insulators and the TMDs are semiconductors, a 5 nm carbon and a 20 nm layer of gold were deposited on the surface of the heterostructure before lamella preparation to prevent charge accumulation during the process. The samples were analyzed in high-resolution scanning transmission electron microscopy (HRSTEM) and transmission electron microscopy (TEM) using a TITAN 80–300 electron microscope (FEI, Netherlands (300 kV)).
4.6 Ferromagnetic resonance and spin pumping
The ferromagnetic resonance (FMR) measurements were obtained using a broadband coplanar waveguide, exciting the sample with a fixed radio-frequency (RF) from 4 GHz up to 14 GHz and sweeping the magnetic field, and therefore, each frequency results in a spectrum. Since the setup utilizes a lock-in detection, an AC field modulation of 45 MHz and 0.5 Oe was used. Those spectra are fitted by a Lorentzian derivative extracting Hres and ΔH. The effective magnetization (4πMeff) was obtained by fitting Kittel's equation:53,54,65 |  | (4) |
where ωRF is the radio-frequency, |γeff| is the effective gyromagnetic ratio, Hres is the resonance magnetic field correspondent to the RF, and 4πMeff is the effective magnetization. As discussed above, the Gilbert damping is obtained through the frequency dependency of the ΔH. The αsp = αeff − αYIG is the difference in the Gilbert damping of the YIG/TMD system and bare YIG. To calculate the spin current density, one needs the FMR data and the RF magnetic field generated by the RF current (hrf = 0.85 G) calculated from:47,82 |  | (5) |
where μ0 is the magnetic permeability, PRF is the RF power, Z is the impedance of the coplanar waveguide, w is the width of the signal line, and d is the distance between the waveguide and the sample.
Author contributions
R. T. V. contributed to the conceptualization, investigation, and writing the original draft. J. F. R. M., B. S. A., and D. A. D. contributed to the investigation. L. C. S., F. G., and J. F. F. contributed to conceptualization, formal analysis, project administration, and supervision. All authors contributed to the formal analysis, writing, review, and editing of the manuscript.
Conflicts of interest
There are no conflicts to declare.
Acknowledgements
The authors thank the Conselho Nacional de Desenvolvimento Científico e Tecnológico (CNPq), Fundação de Amparo à Pesquisa do Estado do Rio de Janeiro (FAPERJ), and Financiadora de Estudos e Projetos (FINEP) for financial support. R. Torrao is thankful for grant no. E-26/201.677/2021 from FAPERJ. Gratefulness to Bruno Pimentel and Igor Evangelista for helpful discussions, Raquel Goncalves for the assistance in the LABNANO, and CBPFs multi-users Laboratories: X-ray Laboratory, Laboratory of Surface and Nanostructures, and LABNANO. Jorlandio F. Felix acknowledges Fundação de Apoio à Pesquisa do Distrito Federal (FAPDF) for financial support (193.00001823/2022-10). In addition, discussions with Dr L. Roncaratti are gratefully acknowledged.
Notes and references
- R. Frindt and A. Yoffe, Proc. R. Soc. London, Ser. A, 1963, 273, 69–83 Search PubMed.
- J. A. Wilson and A. Yoffe, Adv. Phys., 1969, 18, 193–335 CrossRef CAS.
- S. Wu, C. Huang, G. Aivazian, J. S. Ross, D. H. Cobden and X. Xu, ACS Nano, 2013, 7, 2768–2772 CrossRef CAS PubMed.
- A. Splendiani, L. Sun, Y. Zhang, T. Li, J. Kim, C.-Y. Chim, G. Galli and F. Wang, Nano Lett., 2010, 10, 1271–1275 CrossRef CAS PubMed.
- O. Lopez-Sanchez, D. Lembke, M. Kayci, A. Radenovic and A. Kis, Nat. Nanotechnol., 2013, 8, 497–501 CrossRef CAS PubMed.
- S. Hamza Safeer, A. S. V. Ore, A. R. Cadore, V. O. Gordo, P. G. Vianna, I. C. Carvalho, V. Carozo and C. J. de Matos, J. Appl. Phys., 2022, 132, 024301 CrossRef CAS.
- B. Radisavljevic, A. Radenovic, J. Brivio, V. Giacometti and A. Kis, Nat. Nanotechnol., 2011, 6, 147–150 CrossRef CAS PubMed.
- W. Choi, M. Y. Cho, A. Konar, J. H. Lee, G.-B. Cha, S. C. Hong, S. Kim, J. Kim, D. Jena and J. Joo,
et al.
, Adv. Mater., 2012, 24, 5832–5836 CrossRef CAS PubMed.
- X. Li, L. Yang, M. Si, S. Li, M. Huang, P. Ye and Y. Wu, Adv. Mater., 2015, 27, 1547–1552 CrossRef CAS PubMed.
- E. Kim, J.-W. Cho, B. R. Kim, T. T. T. Nguyen, Y.-H. Nam, S.-K. Kim, S. Yoon, Y. S. Kim, J.-H. Lee and D.-W. Kim, Adv. Mater. Interfaces, 2018, 5, 1701637 CrossRef.
- V. Zatko, M. Galbiati, S. M.-M. Dubois, M. Och, P. Palczynski, C. Mattevi, P. Brus, O. Bezencenet, M.-B. Martin and B. Servet,
et al.
, ACS Nano, 2019, 13, 14468–14476 CrossRef CAS PubMed.
- W. Zhu, H. Lin, F. Yan, C. Hu, Z. Wang, L. Zhao, Y. Deng, Z. R. Kudrynskyi, T. Zhou and Z. D. Kovalyuk,
et al.
, Adv. Mater., 2021, 33, 2104658 CrossRef CAS PubMed.
- W. Zhu, S. Xie, H. Lin, G. Zhang, H. Wu, T. Hu, Z. Wang, X. Zhang, J. Xu and Y. Wang,
et al.
, Chin. Phys. Lett., 2022, 39, 128501 CrossRef.
- I. Bilgin, F. Liu, A. Vargas, A. Winchester, M. K. Man, M. Upmanyu, K. M. Dani, G. Gupta, S. Talapatra and A. D. Mohite,
et al.
, ACS Nano, 2015, 9, 8822–8832 CrossRef CAS PubMed.
- J.-H. Ahn, W. M. Parkin, C. H. Naylor, A. C. Johnson and M. Drndić, Sci. Rep., 2017, 7, 4075 CrossRef PubMed.
- Y. Zhang, Y. Yao, M. G. Sendeku, L. Yin, X. Zhan, F. Wang, Z. Wang and J. He, Adv. Mater., 2019, 31, 1901694 CrossRef CAS PubMed.
- V. K. Kumar, S. Dhar, T. H. Choudhury, S. Shivashankar and S. Raghavan, Nanoscale, 2015, 7, 7802–7810 RSC.
- P.-C. Shen, Y. Lin, H. Wang, J.-H. Park, W. S. Leong, A.-Y. Lu, T. Palacios and J. Kong, IEEE Trans. Electron Devices, 2018, 65, 4040–4052 CAS.
- Q. Zhang, L. Mei, X. Cao, Y. Tang and Z. Zeng, J. Mater. Chem. A, 2020, 8, 15417–15444 RSC.
- A. Y. S. Eng, A. Ambrosi, Z. Sofer, P. Simek and M. Pumera, ACS Nano, 2014, 8, 12185–12198 CrossRef CAS PubMed.
- J. N. Coleman, M. Lotya, A. ONeill, S. D. Bergin, P. J. King, U. Khan, K. Young, A. Gaucher, S. De and R. J. Smith,
et al.
, Science, 2011, 331, 568–571 CrossRef CAS PubMed.
- J. Peng, J. Wu, X. Li, Y. Zhou, Z. Yu, Y. Guo, J. Wu, Y. Lin, Z. Li and X. Wu,
et al.
, J. Am. Chem. Soc., 2017, 139, 9019–9025 CrossRef CAS PubMed.
- S. Najmaei, Z. Liu, W. Zhou, X. Zou, G. Shi, S. Lei, B. I. Yakobson, J.-C. Idrobo, P. M. Ajayan and J. Lou, Nat. Mater., 2013, 12, 754–759 CrossRef CAS PubMed.
- J. Park, N. Choudhary, J. Smith, G. Lee, M. Kim and W. Choi, Appl. Phys. Lett., 2015, 106, 012104 CrossRef.
- S. M. Eichfeld, L. Hossain, Y.-C. Lin, A. F. Piasecki, B. Kupp, A. G. Birdwell, R. A. Burke, N. Lu, X. Peng and J. Li,
et al.
, ACS Nano, 2015, 9, 2080–2087 CrossRef CAS PubMed.
- J. Gao, B. Li, J. Tan, P. Chow, T.-M. Lu and N. Koratkar, ACS Nano, 2016, 10, 2628–2635 CrossRef CAS PubMed.
- S. H. Safeer, M. V. Moutinho, A. R. Barreto, B. S. Archanjo, O. G. Pandoli, M. Cremona, M. E. Huguenin Maia da Costa, F. L. Freire and V. Carozo, ACS Appl. Nano Mater., 2021, 4, 4172–4180 CrossRef CAS.
- S. H. Safeer, T. L. Vasconcelos, B. S. Oliveira, B. S. Archanjo, M. Nazarkovsky, C. Vilani, F. L. Freire and V. Carozo, J. Phys. Chem. C, 2021, 125, 21011–21017 CrossRef CAS.
- S. A. Han, A. Sohn and S.-W. Kim, FlatChem, 2017, 6, 37–47 CrossRef CAS.
- Y.-C. Lin, W. Zhang, J.-K. Huang, K.-K. Liu, Y.-H. Lee, C.-T. Liang, C.-W. Chu and L.-J. Li, Nanoscale, 2012, 4, 6637–6641 RSC.
- X. Wang, H. Feng, Y. Wu and L. Jiao, J. Am. Chem. Soc., 2013, 135, 5304–5307 CrossRef CAS PubMed.
- Y. Shi, H. Li and L.-J. Li, Chem. Soc. Rev., 2015, 44, 2744–2756 RSC.
- W. Choi, N. Choudhary, G. H. Han, J. Park, D. Akinwande and Y. H. Lee, Mater. Today, 2017, 20, 116–130 CrossRef CAS.
- J. Yu, J. Li, W. Zhang and H. Chang, Chem. Sci., 2015, 6, 6705–6716 RSC.
- D. L. Plata, A. J. Hart, C. M. Reddy and P. M. Gschwend, Environ. Sci. Technol., 2009, 43, 8367–8373 CrossRef CAS PubMed.
- T. Kang, T. W. Tang, B. Pan, H. Liu, K. Zhang and Z. Luo, ACS Mater. Au, 2022, 2, 665–685 CrossRef CAS PubMed.
- H. Pedersen, S. T. Barry and J. Sundqvist, J. Vac. Sci. Technol., A, 2021, 39, 051001 CrossRef CAS.
- F.-K. Wang and T.-Y. Zhai, Rare Met., 2020, 39, 327–329 CrossRef CAS.
- D. M. Soares, S. Mukherjee and G. Singh, Chem. – Eur. J., 2020, 26, 6320–6341 CrossRef CAS PubMed.
- Q. Shao, G. Yu, Y.-W. Lan, Y. Shi, M.-Y. Li, C. Zheng, X. Zhu, L.-J. Li, P. K. Amiri and K. L. Wang, Nano Lett., 2016, 16, 7514–7520 CrossRef CAS PubMed.
- R. Bansal, A. Kumar, N. Chowdhury, N. Sisodia, A. Barvat, A. Dogra, P. Pal and P. Muduli, J. Magn. Magn. Mater., 2019, 476, 337–341 CrossRef CAS.
- T. S. Ghiasi, A. A. Kaverzin, P. J. Blah and B. J. Van Wees, Nano Lett., 2019, 19, 5959–5966 CrossRef CAS PubMed.
- M. Isasa, M. C. Martnez-Velarte, E. Villamor, C. Magén, L. Morellón, J. M. De Teresa, M. R. Ibarra, G. Vignale, E. V. Chulkov and E. E. Krasovskii,
et al.
, Phys. Rev. B, 2016, 93, 014420 CrossRef.
- P. Gambardella and I. M. Miron, Philos. Trans. R. Soc., A, 2011, 369, 3175–3197 CrossRef CAS PubMed.
-
S. M. Rezende and S. M. Rezende, Fundamentals of Magnonics, 2020, pp.287–352 Search PubMed.
- W. Zhang, J. Sklenar, B. Hsu, W. Jiang, M. B. Jungfleisch, J. Xiao, F. Y. Fradin, Y. Liu, J. E. Pearson and J. B. Ketterson,
et al.
, APL Mater., 2016, 4, 032302 CrossRef.
- H. Bangar, A. Kumar, N. Chowdhury, R. Mudgal, P. Gupta, R. S. Yadav, S. Das and P. K. Muduli, ACS Appl. Mater. Interfaces, 2022, 14, 41598–41604 CrossRef CAS PubMed.
- A. Azevedo, L. Vilela Leão, R. Rodriguez-Suarez, A. Oliveira and S. Rezende, J. Appl. Phys., 2005, 97, 10C715 CrossRef.
- E. Saitoh, M. Ueda, H. Miyajima and G. Tatara, Appl. Phys. Lett., 2006, 88, 182509 CrossRef.
- D. C. Ralph and M. D. Stiles, J. Magn. Magn. Mater., 2008, 320, 1190–1216 CrossRef CAS.
- T. Kawahara, K. Ito, R. Takemura and H. Ohno, Microelectron. Reliab., 2012, 52, 613–627 CrossRef.
- O. Mosendz, J. Pearson, F. Fradin, G. Bauer, S. Bader and A. Hoffmann, Phys. Rev. Lett., 2010, 104, 046601 CrossRef CAS PubMed.
- A. Gonçalves, I. Barsukov, Y.-J. Chen, L. Yang, J. Katine and I. Krivorotov, Appl. Phys. Lett., 2013, 103, 172406 CrossRef.
- A. Gonçalves, F. Garcia, H. Lee, A. Smith, P. Soledade, C. Passos, M. Costa, N. Souza-Neto, I. Krivorotov and L. Sampaio,
et al.
, Sci. Rep., 2018, 8, 2318 CrossRef PubMed.
- C. Dubs, O. Surzhenko, R. Linke, A. Danilewsky, U. Brückner and J. Dellith, J. Phys. D: Appl. Phys., 2017, 50, 204005 CrossRef.
- N. Beaulieu, N. Kervarec, N. Thiery, O. Klein, V. Naletov, H. Hurdequint, G. De Loubens, J. B. Youssef and N. Vukadinovic, IEEE Magn. Lett., 2018, 9, 1–5 Search PubMed.
- C. Hauser, T. Richter, N. Homonnay, C. Eisenschmidt, M. Qaid, H. Deniz, D. Hesse, M. Sawicki, S. G. Ebbinghaus and G. Schmidt, Sci. Rep., 2016, 6, 20827 CrossRef CAS PubMed.
- S. Li, W. Zhang, J. Ding, J. E. Pearson, V. Novosad and A. Hoffmann, Nanoscale, 2016, 8, 388–394 RSC.
- T. Liu, H. Chang, V. Vlaminck, Y. Sun, M. Kabatek, A. Hoffmann, L. Deng and M. Wu, J. Appl. Phys., 2014, 115, 17A501 CrossRef.
- H. Chang, P. Li, W. Zhang, T. Liu, A. Hoffmann, L. Deng and M. Wu, IEEE Magn. Lett., 2014, 5, 1–4 CAS.
- Y. Sun, Y.-Y. Song, H. Chang, M. Kabatek, M. Jantz, W. Schneider, M. Wu, H. Schultheiss and A. Hoffmann, Appl. Phys. Lett., 2012, 101, 152405 CrossRef.
- J. Ding, T. Liu, H. Chang and M. Wu, IEEE Magn. Lett., 2020, 11, 1–5 Search PubMed.
- V. Vlaminck, J. E. Pearson, S. D. Bader and A. Hoffmann, Phys. Rev. B: Condens. Matter Mater. Phys., 2013, 88, 064414 CrossRef.
- Y. Cao, X. Zhang, X.-P. Zhang, F. Yan, Z. Wang, W. Zhu, H. Tan, V. N. Golovach, H. Zheng and K. Wang, Phys. Rev. Appl., 2022, 17, L051001 CrossRef CAS.
- R. Torrão, O. Alves, B. Archanjo, L. Sampaio and F. Garcia, J. Alloys Compd., 2022, 923, 166300 CrossRef.
- D. Nutting, J. F. Felix, E. Tillotson, D.-W. Shin, A. De Sanctis, H. Chang, N. Cole, S. Russo, A. Woodgate and I. Leontis,
et al.
, Nat. Commun., 2020, 11, 3047 CrossRef CAS PubMed.
- U. Dasgupta, S. Chatterjee and A. J. Pal, Sol. Energy Mater. Sol. Cells, 2017, 172, 353–360 CrossRef CAS.
- C. Lee, H. Yan, L. E. Brus, T. F. Heinz, J. Hone and S. Ryu, ACS Nano, 2010, 4, 2695–2700 CrossRef CAS PubMed.
- H. Terrones, E. Del Corro, S. Feng, J. M. Poumirol, D. Rhodes, D. Smirnov, N. R. Pradhan, Z. Lin, M. A. Nguyen, A. L. Elías, T. E. Mallouk, L. Balicas, M. A. Pimenta and M. Terrones, Sci. Rep., 2014, 4, 1–9 Search PubMed.
- N. Zhang, T. Khan, H. Guo, S. Shi, W. Zhong and W. Zhang, Adv. Mater. Sci. Eng., 2019, 2019, 1354150 Search PubMed.
- P. Tonndorf, R. Schmidt, P. Böttger, X. Zhang, J. Börner, A. Liebig, M. Albrecht, C. Kloc, O. Gordan and D. R. Zahn,
et al.
, Opt. Express, 2013, 21, 4908–4916 CrossRef CAS PubMed.
- W. Schutte, J. De Boer and F. Jellinek, J. Solid State Chem., 1987, 70, 207–209 CrossRef CAS.
- G. Pradhan and A. K. Sharma, Mater. Res. Bull., 2018, 102, 406–411 CrossRef CAS.
- S. Cwik, D. Mitoraj, O. Mendoza Reyes, D. Rogalla, D. Peeters, J. Kim, H. M. Schütz, C. Bock, R. Beranek and A. Devi, Adv. Mater. Interfaces, 2018, 5, 1800140 CrossRef.
- C. H. Ravikumar, G. V. Nair, S. Muralikrishna, D. Nagaraju and R. G. Balakrishna, Mater. Lett., 2018, 220, 133–135 CrossRef CAS.
- M. Weiler, M. Althammer, M. Schreier, J. Lotze, M. Pernpeintner, S. Meyer, H. Huebl, R. Gross, A. Kamra and J. Xiao,
et al.
, Phys. Rev. Lett., 2013, 111, 176601 CrossRef PubMed.
- W. Zhang, M. B. Jungfleisch, W. Jiang, J. E. Pearson and A. Hoffmann, J. Appl. Phys., 2015, 117, 17C727 CrossRef.
- H. Tsai, S. Karube, K. Kondou, N. Yamaguchi, F. Ishii and Y. Otani, Sci. Rep., 2018, 8, 5564 CrossRef PubMed.
- K. Ando, S. Takahashi, J. Ieda, Y. Kajiwara, H. Nakayama, T. Yoshino, K. Harii, Y. Fujikawa, M. Matsuo and S. Maekawa,
et al.
, J. Appl. Phys., 2011, 109, 103913 CrossRef.
- O. Mosendz, V. Vlaminck, J. Pearson, F. Fradin, G. Bauer, S. Bader and A. Hoffmann, Phys. Rev. B: Condens. Matter Mater. Phys., 2010, 82, 214403 CrossRef.
- C. Du, H. Wang, P. C. Hammel and F. Yang, J. Appl. Phys., 2015, 117, 172603 CrossRef.
- J. Sánchez, L. Vila, G. Desfonds, S. Gambarelli, J. Attané, J. De Teresa, C. Magén and A. Fert, Nat. Commun., 2013, 4, 1–7 Search PubMed.
- J. Mendes, O. A. Santos, L. Meireles, R. Lacerda, L. Vilela-Leão, F. Machado, R. Rodrguez-Suárez, A. Azevedo and S. Rezende, Phys. Rev. Lett., 2015, 115, 226601 CrossRef CAS PubMed.
- J. Mendes, A. Aparecido-Ferreira, J. Holanda, A. Azevedo and S. Rezende, Appl. Phys. Lett., 2018, 112, 242407 CrossRef.
- S. Husain, S. Pal, X. Chen, P. Kumar, A. Kumar, A. K. Mondal, N. Behera, N. K. Gupta, S. Hait and R. Gupta,
et al.
, Phys. Rev. B, 2022, 105, 064422 CrossRef CAS.
- C. He, A. Razavi, J. Wei, H. Xu, G. Yu, K. L. Wong, H. Wu, S. Shen, Q. Chen and Z. Zeng,
et al.
, Appl. Phys. Lett., 2020, 117, 172406 CrossRef CAS.
- S. Hait, N. K. Gupta, N. Sharma, L. Pandey, N. Kumar, V. Barwal, P. Kumar and S. Chaudhary, J. Appl. Phys., 2022, 132, 133901 CrossRef CAS.
-
C. Cheng, M. Collet, J.-C. R. Sánchez, V. Ivanovskaya, B. Dlubak, P. Seneor, A. Fert, H. Kim, G. H. Han and Y. H. Leeet al., arXiv, arXiv:1510.03451, 2015, preprint.
- S. Husain, A. Kumar, P. Kumar, A. Kumar, V. Barwal, N. Behera, S. Choudhary, P. Svedlindh and S. Chaudhary, Phys. Rev. B, 2018, 98, 180404 CrossRef CAS.
|
This journal is © The Royal Society of Chemistry 2023 |
Click here to see how this site uses Cookies. View our privacy policy here.