DOI:
10.1039/D2NA00691J
(Paper)
Nanoscale Adv., 2023,
5, 2897-2910
Enhanced detoxification of Cr6+ by Shewanella oneidensis via adsorption on spherical and flower-like manganese ferrite nanostructures†
Received
7th October 2022
, Accepted 31st December 2022
First published on 16th January 2023
Abstract
Maximizing the safe removal of hexavalent chromium (Cr6+) from waste streams is an increasing demand due to the environmental, economic and health benefits. The integrated adsorption and bio-reduction method can be applied for the elimination of the highly toxic Cr6+ and its detoxification. This work describes a synthetic method for achieving the best chemical composition of spherical and flower-like manganese ferrite (MnxFe3−xO4) nanostructures (NS) for Cr6+ adsorption. We selected NS with the highest adsorption performance to study its efficiency in the extracellular reduction of Cr6+ into a trivalent state (Cr3+) by Shewanella oneidensis (S. oneidensis) MR-1. MnxFe3−xO4 NS were prepared by a polyol solvothermal synthesis process. They were characterised by powder X-ray diffraction (XRD), transmission electron microscopy (TEM), X-ray photoelectron spectrometry (XPS), dynamic light scattering (DLS) and Fourier transform-infrared (FTIR) spectroscopy. The elemental composition of MnxFe3−xO4 was evaluated by inductively coupled plasma atomic emission spectroscopy. Our results reveal that the oxidation state of the manganese precursor significantly affects the Cr6+ adsorption efficiency of MnxFe3−xO4 NS. The best adsorption capacity for Cr6+ is 16.8 ± 1.6 mg Cr6+/g by the spherical Mn0.22+Fe2.83+O4 nanoparticles at pH 7, which is 1.4 times higher than that of Mn0.8Fe2.2O4 nanoflowers. This was attributed to the relative excess of divalent manganese in Mn0.22+Fe2.83+O4 based on our XPS analysis. The lethal concentration of Cr6+ for S. oneidensis MR-1 was 60 mg L−1 (determined by flow cytometry). The addition of Mn0.22+Fe2.83+O4 nanoparticles to S. oneidensis MR-1 enhanced the bio-reduction of Cr6+ 2.66 times compared to the presence of the bacteria alone. This work provides a cost-effective method for the removal of Cr6+ with a minimum amount of sludge production.
1. Introduction
Chromium (Cr) is a common environmental pollutant coming from several industries such as wood preservation,1 leather tanning,2 steel production,3,4 wool dyeing,5 painting,6 refractories,4 lasers,7 and electroplating,8 among others. End-of-life products such as unwanted steel, wood,1 leather and textiles, among other materials are extra sources of Cr leakage in the environment. The release of Cr in the environment was also attributed to mining activities,9 and improper waste treatment associated with industrial processes.10 Various Cr-bearing minerals, including chromite, are available in the soil, and bedrock also releases natural Cr into the environment.10 It mainly occurs in two valence states, which are highly toxic carcinogenic11 Cr6+ and less toxic Cr3+. Various technologies have been developed to tackle the presence of Cr6+, including membranes,12 coagulation,13 photocatalysis,14 electrochemical treatments,13 adsorption15,16 and biological treatments.17,18 Integrating both adsorption and biological reduction of Cr6+ together has been proposed as a promising solution.19 Applying such combined methods can overcome the accessibility of certain technologies,20 using less toxic chemicals and reducing the production of contaminated toxic waste.19 The recovered chromium can be used in metallurgical industries and minimize the contaminated landfill.21,22
Microbial reduction of Cr6+ has been regarded as a suitable Cr remediation approach because of being more eco-friendly than the conventional physico-chemical strategies, which are often costly. Recently, many types of bacteria have been reported to detoxify Cr6+ to less toxic Cr3+, including dissimilatory metal-reducing bacteria such as Shewanella oneidensis MR-1.23,24 Under anaerobic conditions, S. oneidensis can use Cr6+ as a terminal electron acceptor,25 however cells exposed to Cr6+ exhibited a loss in their enzymatic activity and cell lysis.26 The bactericidal concentration of Cr6+ was reported to be ∼42–65 mg L−1 for S. oneidensis MR-1.26,27 A lethal effect of heavy metals on the microbes during respiration26,29–31 was considered as a potential limitation for the bio-remediation of Cr6+.26 Compared with physical and chemical materials, the concentration of Cr6+ that can be reduced by bacteria is much lower, and it is a great challenge to improve the efficiency of bioremediation.28
Enhancing the bacterial tolerance to Cr6+ is an effective way to improve the reduction of Cr6+. Zero-valent iron nanoparticles (ZVI NPs) can easily be oxidised to ferric oxides and hydroxides in water. The active surface of ZVI NPs can be decreased due to the attached layers of iron oxides and hydroxides. Shewanella, as iron-reducing bacteria, can reduce the adsorbed Fe3+ to Fe2+, which reverses the oxidation of ZVI NPs, as shown in a review by Dong et al.32 Hematite (α-Fe2O3) particles enhanced the bio-reduction of Cr6+ bio-reduction by S. oneidensis MR-1, but they cause cytotoxicity to such kind of bacteria.33 The reduction of Cr6+ by S. oneidensis was enhanced by goethite (α-FeOOH) and humic acid through the bio-reduction of Fe3+ to Fe2+. The reactivity of magnetite (Fe3O4) was increased by microbial Fe3+ reduction to form Fe2+, which then can reduce Cr6+.34,35
A biocompatible material such as manganese ferrite (MnFe2O4)36 was considered for enhancing microbial respiration of Cr6+. This ferrite was used to accelerate extracellular electron transfer in the microbial fuel cell,37,38 and it showed the highest adsorption capacity among other ferrites for Cr6+.39 The maximum adsorption capacity of MnFe2O4 NPs for Cr6+ was reported to be ranging from 31 to 35 mg g−1.36,39,40
The influence of structural features of MnxFe3−xO4 NPs on Cr6+ adsorption has not been thoroughly explored. The effect of the oxidation state of Mn precursors on the chemical structure, morphological and magnetic properties of MnxFe3−xO4 NPs prepared by scalable polyol solvothermal method has been studied in a few reports41,42 but not in relation to their adsorption efficiency for heavy metals.
Herein, we report syntheses and characterization of the most suitable chemical structure of MnxFe3−xO4 NPs and nanoflowers (NFs) for the best adsorption capacity of Cr6+. The impact of the oxidation states of Mn precursors and variation in Mn doping levels on the chemical structural and morphological characteristics of MnFe2O4 NPs prepared by polyol solvothermal route has been investigated. The nature of Cr6+ adsorption by doped and undoped ferrite NPs and, subsequently, the bio-detoxification of Cr6+ by S. oneidensis have been studied.
2. Results and discussion
2.1 Synthesis of nanomaterials
In polyol synthesis, metal precursors are reduced at a high temperature by alcohols (polyols), which can act as a capping agent, solvent and reductant. Then metal nuclei form, grow and controllably coalesce together to produce the desired particles.43 In such a non-aqueous solvent, the metal oxide NPs were proposed to be formed via two steps. In the first step, solvolysis of the precursor involved an interaction between tetraethylene glycol (TEG) and the selected metal acetylacetonate, causing the generation of metal carboxylate.44 The second step is a condensation reaction in which carboxylate reacts with iron leading to the formation of an oxo-bridge between metal (metal–oxygen–metal clusters) and ultimately resulting in the formation of metal oxide nanocrystals.44
2.2 Characterization of MnxFe3−xO4 NPs
2.2.1 Morphology of MnxFe3−xO4 NPs.
The prepared MnxFe3−xO4 NPs using precursor ratios 0.14 ≤ [Mn(acac)2 or 3]/[Fe(acac)3] ≤ 3 have nearly spherical shape and are well dispersed on TEM grids, with sufficient interparticle distances as shown in Fig. 1A–C and S1.†DTEM (particle diameter determined by TEM) ranged from 5 to 12.5 nm with polydispersity indexes between 0.14 and 0.21, except for 0.33 and 0.6 ratios of [Mn(acac)3]/[Fe(acac)3], prepared at 200 °C and 250 °C, respectively (see Fig. S2†).
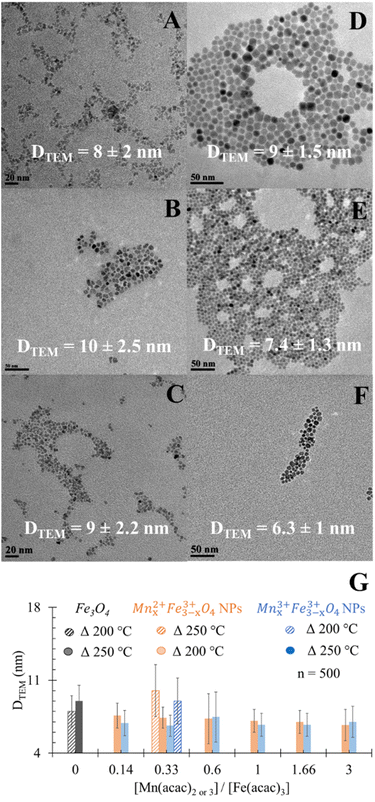 |
| Fig. 1 TEM images for the spherical (A) undoped Fe3O4 NPs, (B) MnxFe3−xO4 of precursor ratio [Mn(acac)2]/[Fe(acac)3] = 0.33, (C) [Mn(acac)3]/[Fe(acac)3] = 0.33 prepared at 200 °C as reaction temperatures, (D) undoped Fe3O4 NPs and (E) and (F) MnxFe3−xO4 NPs prepared with the same precursor ratio but at 250 °C. (G) Impact of reaction temperatures on DTEM of MnxFe3−xO4 NPs prepared from [Mn(acac)2]/[Fe(acac)3] = 0.33. *P < 0.05. | |
Doping Mn had astatistically insignificant change in the DTEM of MnxFe3−xO4 NPs compared to undoped Fe3O4 NPs (Fig. 1D). In the case of using the divalent Mn precursor, a statistically insignificant change in DTEM was observed when increasing the ratio of precursors. This is in agreement with what was reported by Garcia-Soriano et al.45
2.2.2 Crystal structure of MnxFe3−xO4 NPs.
XRD of Fe3O4 NPs and MnxFe3−xO4 NPs, which were prepared at a temperature of 200 °C, are shown in Fig. S3.† XRD of the undoped Fe3O4 NPs, which were formed at 250 °C with an ageing time of 6 h, are shown in Table S1.† The main peaks at the diffractogram of these NPs appear at 21.5° (111), 35.1° (220), 41.4° (311), 50.4° (400), 62.8° (422), 67.3° (511), and 74.1° (440). These peak locations matched ICDD PDF card no. 01-086-2344, revealing the formation of iron oxide (FeO–Fe2O3).46–48 Incorporating Mn ions into the Fe3O4 lattices as substitutional atoms was then implemented with both Mn(acac)2, and Mn(acac)3 precursors. By increasing the ratio of [Mn(acac)2 or 3] to [Fe(acac)3], a small shift of the peaks towards a lower 2θ value (closer to the reference peak of MnFe2O4) was observed in the XRD patterns (Fig. 2 and S4†). Using Mn(acac)3 (Fig. S4†) caused a relocation of XRD peak positions closer to the reference peak positions of MnFe2O4 (2θ = 40.8°). This can be attributed to a further inclusion of Mn3+ into the spinel iron oxide lattice due to the similar ionic radii between Mn3+ and Fe3+ (0.64 Å for both (ref. 49)), which are smaller than that of Mn2+ (0.80 Å).49
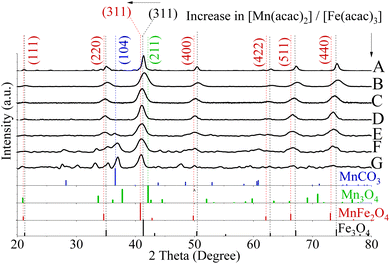 |
| Fig. 2 XRD patterns for fcc lattice of Fe3O4 (A) and MnxFe3−xO4 NPs where [Mn(acac)2]/[Fe(acac)3] were 0.14 (B), 0.33 (C), 0.6 (D), 1 (E), 1.66 (F), 3 (G). The horizontal arrow pointed out the shifting in the peak of 311 from the reference Fe3O4 (PDF card no. 01-089-0688) towards the lower diffraction angle of MnFe2O4 (PDF card no. 00-010-0319) in response to the increase in [Mn(acac)2]/[Fe(acac)3]. A secondary phase of MnCO3 (Reference ICDD PDF card no. 00-044-1472) was found for NPs prepared from (1 ≤ [Mn(acac)2 or 3]/[Fe(acac)3] ≤ 3) (E)–(G). The synthesis temperature for all NPs was 250 °C. The vertical arrow indicated the gradual increase in [Mn(acac)2]/[Fe(acac)3] from (A)–(G) Mn3O4 (PDF card no. 01-080-0382). | |
With an increase in the Mn precursor concentration, the slight broadening of full-width half maximum (FWHM) of the most intense XRD peaks (311), was observed, which implies a reduction in crystal size.50 Calculated size (in diameters) is summarized in Table S1.†
The use of 0.33 ≤ [Mn(acac)2 or 3]/[Fe(acac)3] ≤ 0.6 resulted in the formation of MnFe2O4 with an fcc structure as verified by XRD patterns and presented in Fig. 2B–D and S4A–C.† The XRD peaks appearing matched with ICDD PDF card no. 00-010-0319 of MnFe2O4. The lattice planes correspond to the cubic spinel structure of MnFe2O4 (ICDD card no. 00-010-0319). For NPs prepared using 1 ≤ [Mn(acac)2]/[Fe(acac)3] < 7 in Fig. 2E–G, the distinct additional peaks at 2θ values of 36.8, 36.9° and 37.0°, respectively, indicated the formation of a secondary phase that was indexed to the (104) Miller plane of MnCO3 (ICDD card no. 00-044-1472). In Fig. 2F and G, peaks attributed to the tetraethyleneglycol (TEG) molecule appeared at 2θ equal to 27.8° and 27.6° respectively, as shown in the XRD of TEG compound alone before and after thermal treatment (Fig. S5†) as reported by Vamvakidis et al.49 as well as Khanna and Verma.51 Peaks were noticed at 30.2°, 33.1° which were assigned to MnOOH (ICDD PDF card no. 01-074-1631, data are not shown), and MnO2 (ICDD PDF card no. 00-024-0735, data are not shown) correspondingly. The presence of multiple phases of Mn oxides/hydroxides was attributed to the formation of H2O and Mn2O3 (the products of thermal decomposition of Mn(acac)2),52 which can lead to oxidation of Mn3+ into Mn4+ and hydroxylation of Mn3+ oxides.
The increase in the [Mn(acac)2 or 3]/[Fe(acac)3] led to a slight broadening in the 311 peaks, which indicated a possible alteration of the crystal size,50,53 as determined by measuring the FWHM and summarized in Table S1.†42,53 The calculated crystal size obtained from XRD of samples (6.5–7 and 5–7.5 nm for NPs prepared from divalent and trivalent Mn precursors, respectively, see PDI of crystal size in Fig. S6†) were within the range of the average particle size derived from TEM. Therefore, these NPs were considered to be single crystalline. However, the XRD analysis indicated the presence of MnCO3 for NPs synthesised with precursor ratios in the range of 1 ≤ [Mn(acac)2 or 3]/[Fe(acac)3] ≤ 3, and the crystal sizes determined by XRD were also within the size range observed by TEM.
2.3 Characterization of MnxFe3−xO4 NFs
The preparation of MnxFe3−xO4 NFs was implemented through a modified solvothermal method, and the morphology was precisely regulated by varying the ratios between [Mn(acac)3]/[Fe(acac)3] precursors as well as the reaction temperature as shown in Fig. 3A and B and [Mn(acac)2]/[Fe(acac)3] in Fig. S7.†
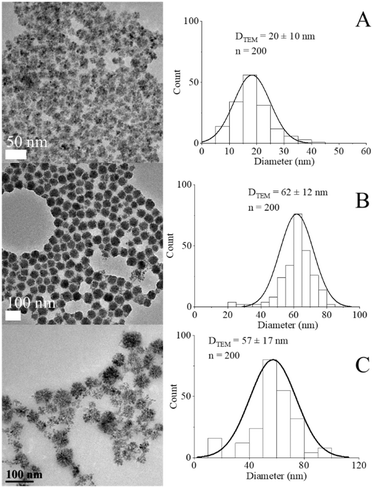 |
| Fig. 3 TEM images and histograms for DTEM of NFs prepared from [Mn(acac)3]/[Fe(acac)3] = (A) and (B) 1 & 3, respectively at 200 °C, (C) ratio = 7 at 250 °C. | |
2.3.1 Morphology of MnxFe3−xO4 NFs.
At 200 °C, the polyol solvothermal method resulted in a well-defined flower-like structure with a narrow size distribution, as shown in Fig. 3A and B. The morphology of MnxFe3−xO4 NFs (Fig. 3B) matched the shape of CoFe2O4 NFs, which was reported by Fu et al., but ours were smaller in size (DTEM of MxFe3−xO4 NFs = 60 ± 12 nm vs. DTEM of CoFe2O4 NFs = 164.8 ± 20.7 nm).54 The smaller diameter of MnxFe3−xO4 NFs than the reported CoFe2O4 NFs by 2.75 folds can be attributed to sodium hydroxide used during the solvothermal preparation of CoFe2O4 NFs,54 which accelerated the hydrolysis of the precursors and promoted the formation of larger oxide clusters.55 TEM analysis of NPs synthesized at 250 °C with a ratio [Mn(acac)3]/[Fe(acac)3] equal to 7 showed the formation of aggregated crystalline particles in clusters (Fig. 3C).
2.3.2 Crystal structure of MnxFe3−xO4 NFs.
Fig. 4 shows diffraction patterns of MnxFe3−xO4 with crystal size of NFs being in the range 6–8 nm. The small crystal size in comparison to DTEM of NFs (Fig. 3) implied the formation of primary nanocrystals, which do not grow significantly. The primary nanocrystals aggregated into larger secondary particles and coarsening, as shown in Fig. 3A–C and as described by Gavilan.56 The generation of MnCO3 accelerated the hydrolysis of the precursors and promoted the formation of larger oxide clusters.54 In our case, nano-clusters were prepared in a single step which included the synthesis of nanoparticles and their coalescence. Shifting in the peak of 311 from the reference Fe3O4 (PDF card no. 01-089-0688) towards a lower diffraction angle of MnFe2O4 (PDF card no. 00-010-0319) in response to the increase in [Mn(acac)3]/[Fe(acac)3] from 1 to 3 was observed, being also an indicator for inclusion of Mn ions into the Fe3O4 lattices.
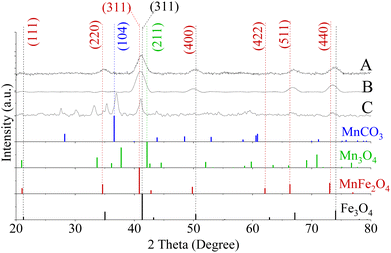 |
| Fig. 4 XRD patterns of MnxFe3−xO4 NFs prepared from [Mn(acac)3]/[Fe(acac)3] ratio equal to (A) 1 and (B) 3 at 200 °C, (C) 7 at 250 °C. A secondary phase matched MnCO3 (Reference ICDD PDF card no. 00-044-1472) was found for NFs prepared from a precursor ratio equal to 7 at 250 °C. No detected peaks matched Mn3O4 (PDF card no. 01-080-0382). | |
In the case of preparing NPs with [Mn(acac)3]/[Fe(acac)3] ratio equal to 7 at 250 °C, the XRD pattern revealed the formation of a polycrystalline material corresponding to a mixture of phases. As shown in Fig. 4D, the peaks at 36.9° (104) and 27.7° (102) diffraction peaks were indexed to MnCO3 (JCPDS card no. 00-044-1472). The 2θ Bragg reflections at 21.5° (111), 35.4° (220), 41.0° (311), 50.1° (400), 66.6° (511), and 73.5° (440) confirmed the formation of MnFe2O4 (JCPDS card no. 00-010-0319). A peak at 27.5° was assigned to TEG51, which was supported by our results, as shown in Fig. S5.† Also, peaks appeared at 2θ equal to 30.1°, 33.2° and 35.3° were related to MnOOH (JCPDS card no. 01-074-1631) and MnO2 (JCPDS card no. 00-024-0735). Our results revealed that the increase in ratios between the used precursors led to the formation of nano-clusters of MnxFe3−xO4, which matched what was reported for MnxFe3−xO4 (ref. 57) and other ferrites by a solvothermal method.58 The inability of nanocluster formation using [Mn(acac)2]/[Fe(acac)3] equal to 7 (Fig. S7†) can be attributed to the relative thermal stability of Mn(acac)2 which limits its decomposition.52
2.4 Elemental analysis of MnxFe3−xO4 NPs
Results of elemental analyses are presented in Fig. 5A, showing a significant positive relationship between the Mn doping level and [Mn(acac)2 or 3]/[Fe(acac)3] ratios. The doping level of Mn in MnxFe3−xO4 NPs was probably the reason behind the small shifts in XRD patterns from the reference peak of Fe3O4 towards a lower diffraction angle of MnFe2O4 when [Mn(acac)2 or 3]/[Fe(acac)3] increases as shown in Fig. 2, S3 and S4.† The variation in the Mn doping levels was not significantly affected by the oxidation state of Mn precursor except in the cases when [Mn(acac)2 or 3]/[Fe(acac)3] ratios were equal to 0.14 and 0.33. The faster thermal decomposition of Mn(acac)3 than Mn(acac)2 (ref. 52) resulted in more Mn-rich NPs that were prepared by the trivalent Mn precursor than those prepared by the divalent Mn precursor.
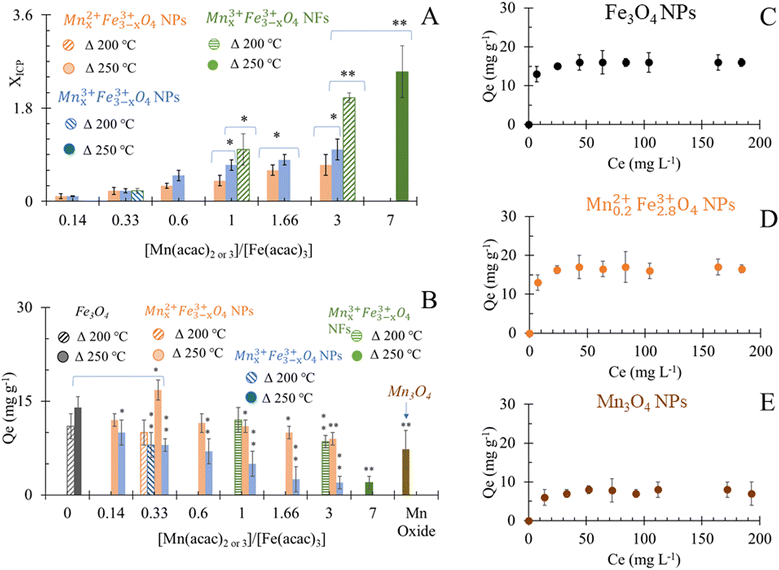 |
| Fig. 5 (A) Elemental analysis of MnxFe3−xO4 NS that were prepared at 200 °C and 250 °C. *P < 0.05 and **P < 0.01 in comparison to Mnx3+Fe3−x3+O4 NPs of similar precursor ratios; (B) adsorption capacity of NPs for Cr6+, *P < 0.05 and **P < 0.01 in comparison to Fe3O4 NPs; (C)–(E) adsorption isotherm of Cr6+ by Fe3O4, Mn0.22+Fe2.83+O4 and Mn3O4 NPs respectively. | |
Overall, at 250 °C, the change in the oxidation state and the ratios between the precursors did not show a variation in the morphology of NPs, but it significantly affected the Mn doping level. While at a synthesis temperature of 200 °C, the oxidation state and the ratios between the precursors affected both the Mn doping level and resulted in different shapes of MnxFe3−xO4 NPs and NFs.
2.5 Functionalisation of NPs and NFs
The advantage of applying a small molecule like citrate as a ligand is that a smaller hydrodynamic radius (DHD) of NPs can be obtained compared to polymeric ligands, which are reflected in hydrodynamic size (Fig. S8†). Yet, the hydrodynamic shell size of citrate-coated NPs was large enough to maintain a physical barrier leading to good dispersibility. The obtained stable dispersions of nano-colloids were attributed to the negative charges induced by the citrate59 as determined by ζ-potentials (Fig. S9†).
The most negative value of ζ-potential was observed for MnxFe3−xO4 NPs of [Mn(acac)2 or 3]/[Fe(acac)3] = 3. The colloidal stability was attributed to a weak base (MnCO3), as expected from XRD patterns (Fig. 2E–G and 4A) and the negative charge of citrate. For other MnxFe3−xO4 NPs and NFs, the Mn doping level did not show any crucial impact on their ζ-potentials.
The FTIR measurements, as shown in Fig. S10,† confirmed that TEG ligand was exchanged by trisodium citrate, similarly observed by Chakraborty et al.60 Carboxylates exhibited strong absorptions for infra-red spectrum due to their characteristic asymmetric (at 1620–1560 cm−1) and symmetric carbonyl stretching (1440 to 1310 cm−1). Bands in the region of 1280–1027 cm−1 represented the deformation of C–H.61–63
2.6 Adsorption of Cr6+ by NS
The adsorption of Cr6+ by MnxFe3−xO4 NPs and NFs was studied as a function of the chemical composition and morphology of nanostructures. The quantities of Cr6+ (Qe) adsorbed onto citrate-coated Fe3O4 NPs were estimated to be 14 ± 1.7 mg g−1 at room temperature and pH 7 at equilibrium (Fig. 5B). The smaller size of the NPs presented herein can probably explain the 1.4 fold higher adsorption efficiency compared to that reported by Luther et al.64 In aqueous solution, Cr6+ mainly was present in oxyanion form of chromate (Cr2O7)2− species and formed sphere complexes with iron oxide via surface hydroxyl exchange,15,36,40,65 resulting in the generation of monodentate complexes, with simultaneous desorption of surface hydroxyl groups from the metal oxide surface sites.15,36,40,65 Upon evaluating commercially available Mn3O4 as a control material under the same conditions, the binding capacity of Cr6+ (7 ± 2.6 mg g−1) was significantly lower than to Fe3O4 which was attributed to the physisorption affinity of Cr6+ to such material.66
The higher adsorption capacity of NFs in comparison to NPs made of similar [Mn(acac)3]/[Fe(acac)3] ratio (1 and 3) but at different synthesis temperatures (200 °C vs. 250 °C) was attributed to the higher surface area which allows Cr6+ to penetrate into NFs.67 Increasing the doping level of Mn in NFs causes a decrease in Cr6+ adsorption as Mn0.83+Fe2.83+O4 NFs that was prepared from [Mn(acac)3]/[Fe(acac)3] = 1 can adsorb 12 ± 2 mg g−1, while using [Mn(acac)3]/[Fe(acac)3] = 3 resulted in NFs with adsorption capacity equal to 8.5 ± 2 mg g−1.
Under the employed conditions, only Mn0.22+Fe2.83+O4 (x = 0.2), prepared at 250 °C was comparable to adsorption capacity to Fe3O4 NPs (16.8 ± 1.6 vs. 14 ± 1.7 mg g−1). The use of Mn0.22+Fe2.83+O4 NPs improved the Cr6+ adsorption by over 2 fold compared to Mn3O4, as shown in Fig. 5B. The adsorption of chromate anions was due to the formation of weak bonds with MnxFe3−xO4 substrate.40 The adsorption capacity of Cr6+ by stoichiometric MnFe2O4 NPs was reported to be higher than by non-stoichiometric Mn1−xCoxFe2O4 (x = 0.2, 0.4 and 0.6).68 Our results are in agreement with what was stated by Martinez-Vargas et al.69 as non-stoichiometric Mn0.25Fe2.75O4 NPs exhibited the best adsorption capacity to As3+. The surface of Mn0.22+Fe2.83+O4 has been reported to be rich in hydroxyl groups69 which favour Cr6+ adsorption. The colloidal dispersion of our NPs and their small diameter can explain the reason behind their better adsorption capacity compared to other reported MnFe2O4 NPs (15 mg g−1 (ref. 36) and 13.54 mg g−1 (ref. 70)), while it is also comparable with results from other reports (18.02 mg g−1).40 The increase in the Co substitution for iron in magnetite (Fe3−xCoxO4, 0 ≤ x ≤ 1) enhanced the adsorption capacity of NPs to Cr6+ slightly.71 Increasing the zinc content in magnetite (Fe3−xZnxO4, x = 0, 0.25, 0.49) has been reported to initially decrease the Cr removal efficiency, but Fe2.26Zn0.74O4 and Fe2.1Zn0.99O4 led to its improvement.72
Except Mn0.22+Fe2.83+O4, MnxFe3−xO4 NPs prepared from both Mn sources showed an inverse trend for adsorption capacity of Cr6+ with the increase of x in comparison to Fe3O4 NPs. The inverse relationship between Mn concentration in the ferrite composition and adsorption of heavy metals was also observed in the case of arsenic adsorption by MnxFe3−xO4 NPs and was attributed to low binding affinity to the As.69 Introducing Mn into ferrite reduced the adsorption capacity of Fe3O4 to Cr6+ from 15.9 mg g−1 to 8.54–8.9 mg g−1.64,70 It was suggested that the release of Mn cations into the solution as a result of reduction of Cr6+ (ref. 64) alters the surface structure. The decrease in Mn doping in the Mn1−xCoxFe2O4 (x = 0.2, 0.4 and 0.6) induced a progressive, positive impact on the adsorption efficiency of Cr6+. Since Mn2+ ions have larger ionic radii than Co2+ (0.8 Å vs. 0.7 Å), the increase in x turned the overcoming of energy barriers for ion exchange interaction more difficult.68 Given that the physical mechanism of Cr6+ adsorption on the surface of oxide was reported to be a combination of electrostatic interactions between charged oxides and Cr6+ and ion exchange in the aqueous solution,40 the increase in Mn doping level showed a negative impact on Cr6+ adsorption by Mn1−xCoxFe2O4.68 At higher dopant concentration x = 0.8, more CoFe2O4 was proposed to be formed on the surface.68 Considering the larger ionic radii of Mn2+ cation than Fe3+ (0.80 Å vs.49 0.64 (ref. 49)), our results can be explained on the basis of the reverse impact of Mn doping level on the adsorption capacity of MnxFe3−xO4 NPs to Cr6+. Yet, in the case of Mnx3+Fe3−x3+O4, the ionic radius of Mn3+ is smaller than Mn2+ and approximately equal to Fe3+ radius but Mnx3+Fe3−x3+O4 NPs showed lower adsorption capacities than their Mn0.22+Fe2.83+O4 NPs counterparts. The inferiority of Mn3+ in the adsorption of Cr6+ could be attributed to the lower redox potential of Mn3+/Mn2+ (+1.5 V) than Fe3+/Fe2+ (+1.9 V).49 Mn2+ has half-filled 3d orbital ([Ar] 3d5 4S0), which makes it more stable than Mn3+ ([Ar] 3d4 4S0). While, oxidizing Fe2+ ([Ar] 3d6 4S0) of Fe3O4 NPs into more stable Fe3+ ([Ar] 3d5 4S0) is favored and can support the possible redox-based adsorption of Cr6+.
The adsorption of Cr6+ by the selected citrate-coated adsorbents that showed the best Qe at pH 7 at room temperature can be described by Langmuir isotherm model as a function of the initial Cr6+ concentrations (Fig. 5C–E). Hence, the surface of nano-sorbents has homogeneous energy distribution via a monolayer sorption process. The calculated maximum adsorption capacity (Qmax) by Langmuir isotherm model fitted the results of Qe.
2.7 The Raman spectrum of Mn0.22+Fe2.83+O4 NPs
Raman spectroscopy is also a useful tool that provides further structural details.73 Raman spectra of representative Fe3O4 and Mn0.22+Fe2.83+O4 NPs were recorded. The Raman spectrum of Fe3O4 (Fig. S11A†) expressed 5 Raman active modes including 190 (T2g(1)), 340 (Eg), 490 (T2g(2)), 540 (T2g(3)) and 670 cm−1 (A1g). For Mn0.22+Fe2.83+O4 NPs, the broad A1g band involved two modes centered at 595 and 670 cm−1 due to the presence of Mn and Fe cations. The Raman shift at 220 cm−1 in Fig. S11B† showed an induced phase transition at the surface of the Mn0.22+Fe2.83+O4 NPs due to the laser's power.74
2.8 The oxidation state of Mn and Fe in Mn0.22+Fe2.83+O4 and Mnx3+Fe3−x3+O4 NPs
XPS was utilized to gain insights into the chemical composition and oxidation state of the selected MnxFe3−xO4 NPs, which have either maximum or minimum Cr6+ adsorption capacity (Fig. 5). Binding energies (BE) were used to identify different elements and their valence states. In Fig. S12,† the wide-scan spectra of Mn0.22+Fe2.83+O4 and Mnx3+Fe3−x3+O4 NPs indicate the presence of carbon (C) and oxygen (O) elements besides Mn and Fe. Using the relative area under the deconvoluted XPS bands, a semi-quantitative estimation of the valence states of the elements in the mixed-valence compounds was achieved.
The presence of C was identified by BEs of C 1s around 284.6, characteristic energies correspond to C–C, C–O–C, O–C
O, and C
O bonds which are due to the presence of surfactant. The presence of O in the XPS spectra was attributed to the metal oxide itself, hydroxyl bonded to metal or adsorbed H2O as was expressed by BE of O 1s at 529.7 eV. Other BE appeared at 531.0, 532.3, and 535.3 eV were ascribed to C
O and C–O bonds coming from the ligand.
2.8.1 The oxidation state of Mn.
In Fig. 6A, Mn 2p was fitted by 5 contributions at around 640.2, 642.1, 645.2, 651.6 and 653.4 eV. The broadening of peaks demonstrated that Mn is present in an oxide form rather than a metallic one.75,76 The asymmetric Mn 2p3/2 main metal peak at 640.2 eV was subjected to a 2p3/2 to 2p1/2 splitting of FWHM 2.7 and 2.1 eV, respectively. Binding energies of 640.2 and 651.1 eV were reported to be related to Mn 2p3/2 and Mn 2p1/2 of Mn2+ correspondingly.40,75–77 The small satellite peak at 645.2 eV (≈14%, 2.9 eV FWHM, Table S2†) was assigned to MnO. Since stoichiometric MnxFe3−xO4 can be expressed as MnO–Fe2O3, this points to the formation of MnxFe3−xO4 NPs75 in agreement with XRD results (Fig. 2B).
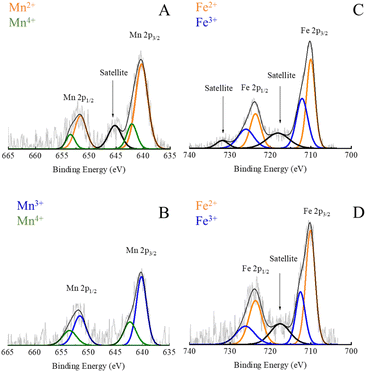 |
| Fig. 6 High-resolution XPS spectra of Mn 2p in Mn0.22+Fe2.83+O4 (A); Mn 2p in Mnx3+Fe3−x3+O4 NPs (B); Fe 2p in Mn0.22+Fe2.83+O4 (C) and Fe 2p in Mnx3+Fe3−x3+O4 NPs (D). | |
Nevertheless, in the case of Mnx3+Fe3−x3+O4 NPs, the BE of Mn 2p was fitted by 4 peaks which are located at 640.2, 642.1, 651.6 and 653.4 eV, as shown in Fig. 6B. The absence of a small satellite peak at 645.2 was reported due to the formation of either Mn3+ or Mn4+.30
A partial oxidation of Mn2+ to Mn3+ has been reported for MnxFe3−xO4 NPs prepared via polyol solvothermal method using Mn(acac)2 precursor42 due to the oxidative atmosphere inside the autoclave.49 Both divalent and trivalent Mn cations were reported to be present in MnxFe3−xO4 NPs with a strong preference for tetrahedral sites and octahedral positions for Mn2+ and Mn3+, respectively.42 Hence, trivalent Mn was supposed to be formed during the synthesis of NPs as suggested by XRD and matched MnCO3 ICDD PDF card no. 00-010-0319. The relatively lower amount of Mn2+ in Mn3+-substituted ferrites could account for the fact that Mnx3+Fe3−x3+O4 NPs showed lower Cr6+ adsorption capacity than Mn2+-substituted ferrites (Fig. 5B).
2.8.2 The oxidation state of Fe.
For both samples, as shown in Fig. 6C and D, a peak of Fe 2p3/2 for Fe3+ was spotted at 710 eV, and its satellite appeared at 718 eV. The asymmetric peaks are situated at 723.6 eV, attributed to Fe3+ 2p1/2. For Fe 2p1/2, another satellite peak was observed at 729.5 eV. The peak of Fe 2p1/2 was wider and weaker than Fe 2p3/2 peak, and the FWHM of Fe 2p1/2 peak is smaller than that of Fe 2p3/2 because of spin–orbit (j–j) coupling. From the calculated FWHM (Table S3†), the FWHM of Fe 2p peaks at 712 eV was slightly smaller than its counterpart at 712 eV, which can serve as an indicator for the presence of both Fe2+ and Fe3+ in these two samples.76,78 This interpretation matched the elemental analysis results by ICP (Fig. 5A) for the formation of non-stoichiometric MnxFe3−xO4. Furthermore, the absence of the satellite peak at 732 eV, as shown in Fig. 6D, was attributed to the presence of Fe3O4.78
Mn0.22+Fe2.83+O4 NPs adsorb Cr6+via an ion exchange between the hydroxyl groups on the surface of NPs and chromate (Cr2O7)2− oxyanion.15,36,40,65 The adsorption of Cr6+ on iron oxides/hydroxides was reported to generate inner-sphere coordination complexes,79,80 in which chromates are linked to a central metal atoms (or ions) by covalent bonds. Fe forms monodentate (one covalent bond) and bidentate (two covalent bonds) complexes with chromates.79,80 The inner-sphere complex is strong and non-reversible.79 The reduction of adsorbed Cr6+ to Cr3+ by Fe2+ or Mn2+ resulted in the formation of precipitated Cr(OH)3 or CrxFe1−x(OH)3.72 So, there is a possibility of the presence of Cr6+ and Cr3+ on the surface of NS.
Due to the highest adsorption capacity of Mn0.22+Fe2.83+O4 NPs, this sample was selected to explore its enhancement impact on the bio-reduction of Cr6+ by S. oneidensis MR-1 in comparison to undoped Fe3O4 and Mn3O4 NPs.
2.9 The lethal dose of Cr6+ for the tested Shewanella
Shewanella bacterial species are considered metal-reducing and resistant bacteria.26 In our work, results revealed that the minimum inhibition concentration (MIC) of Cr6+ for the tested wild-type Shewanella (S. oneidensis and S. loihica PV-4, see the molecular identification (Table S4†)) was 60 mg L−1 and 70 mg L−1 respectively, being slightly higher than what was reported previously.26 For S. oneidensis JG1486 and JG3355 (molecular identification at ESI†), MICs were 20 mg L−1 and 5 mg L−1, respectively. The bactericidal effect of Cr6+ was documented because of being taken up by Shewanella intracellularly and caused cell lysis. In fact, the toxic effect of Cr3+ appeared to be associated with extracellular interactions, leading to stress-associated cell morphology and then to a lethal effect.26,81–83 Before reacting with Cr6+, the wild-type S. oneidensis MR-1 and S. loihica PV-4 cells were reported to be regular small rod-shaped with smooth surfaces.26,81–83 Meanwhile, the bacterial cells changed to be atrophic with a shrunken-surface shape and crack formation was also observed after the reaction.26,81,83 However, S. loihica PV-4 cells were reported to be elongated and exhibited a rough surface upon exposure to Cr6+,82 which can explain why S. loihica PV-demonstrated higher resistance and reduction ability for Cr6+. As hazardous metal ions could damage microbial DNA when they entered the cells, extracellular reduction benefitted Shewanella for their survival.82 The low resistance of mutants was due to the inability of bio-reduction of Cr6+ for JG1486,84 but the possibility of the presence of other stress regulators made such mutants more resistant regarding JG3355.85,86
2.10 Effect of the selected NPs on the viability of Shewanella in response to the sublethal concentration of Cr6+
In the absence of Cr6+, tri-sodium citrate alone, citrate-coated Mn0.22+Fe2.83+O4, Fe3O4 and Mn3O4 can sustain the viability of bacteria. At a sub-lethal concentration of Cr6+, the viability of wild-type bacteria was improved in the tested groups amended by citrate alone (only 10–12%), as illustrated in Fig. 7A. Bencheikh-Latmani et al.87 explained a similar observation as a result of the complexation between the product of bio-reduction (Cr3+) and citrate, which consequently limits the availability of the toxic metal to bacterial cells.87 In response to Cr6+ toxicity, Mn0.2Fe2.8O4, Fe3O4 and Mn3O4 NPs improved the viability of S. oneidensis JG1486 strain by 3.3, 2.5, 1.3 folds, and of S. oneidensis JG5533 strain by 1.2, 0.5, 0.2 folds, respectively.
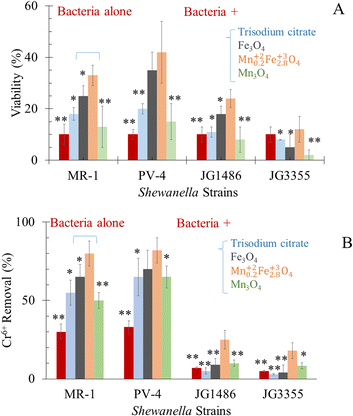 |
| Fig. 7 In the presence of the tested agents (A) viability of tested Shewanella under sublethal dose of Cr6+ (B) removal of Cr6+ by Shewanella strains. *P < 0.05 and **P < 0.01 in relation to the impact of Mn0.22+Fe2.83+O4 NPs in each data set separately. | |
2.11 Bio-reduction of Cr6+ by tested Shewanella
For safe removal of Cr6+, such hexavalent cations should be detoxified by reduction to Cr3+. The capability of S. oneidensis83 to respire Cr6+ was affected by the initial concentration of the heavy metal.83,88,89 These results were credited for the chromate dose-dependent toxicity, which causes growth and viability inhibition.83,88 This occurred in the presence of Cr6+ alone83,88,89 or in the presence of goethite and humic acid34 or ferric oxyhydroxide mediators.83,88 Hence, our experiments were designed at a high concentration of Cr6+, i.e. sub-lethal dose.
Our results (Fig. 7B) revealed a significant drop in the concentration of Cr6+ in media supplemented by both wild-type of Shewanella which included the strain of interest (S. oneidensis MR-1) and positive control (S. loihica PV-4); this was attributed to the respiration of Cr6+ into Cr3+ form81,90,91 or bio-sorption92,93 by bacterial cells. The drop in the concentration of Cr6+ in the medium exposed to S. oneidensis JG1486 and JG3355 was significantly lower than those supplemented by both wild type bacteria.
The tested Shewanella oxidized lactate (electron source), and the liberated electrons are transferred via the respiratory chain to be directed to an externally available terminal electron acceptor (Cr6+). The redox potential of Cr6+ (1.33 V vs. standard hydrogen electrode; SHE) has been reported to be higher than the redox potential of oxygen (1.23 V) and the electron source (−0.19 V).94 So, Cr6+ was considered a favourable electron acceptor for bacteria in the process of respiration as bacteria gain more energy.94
Cr6+ can be reduced extracellularly82 and also transported into the cell interior and then reduced in the cytoplasm.82 The ability of Shewanella to transfer electrons to metal ions was known to take place via one of four porin–cytochrome conduits; the MtrCAB complex,95 the MtrFED complex,96 the DmsEFA dimethyl sulfoxide reductase system97 and the SO4359–SO4360 system.96 The superiority of S. loihica PV-4 in the respiration of Cr6+ in our experiment was thanks to their higher content of c-type cytochrome genes in the metal reductase-containing locus than S. oneidensis MR-1.98S. oneidensis JG1486 (ΔmtrCAB/ΔmtrFED/ΔomcA/ΔdmsE/ΔSO4360/ΔcctA/ΔrecA) lacks the responsible genes for extracellular metal reduction.84 The recombination between the expression of outer membrane cytochromes (controlled by lac promoters) and periplasmic electron carriers was stopped by the deletion of RecA gene.84 Such mutants showed the lowest removal of Cr6+ as a result of the inability of bioreduction.84S. oneidensis JG3355 lacked both ClpX and ClpP genes.86 The role of ClpXP has been revealed for regulating Fe2+ stress in anaerobic bacteria86 and stress regulation (ClpP) in response to 24 h Cr6+ exposure.85 Therefore, the inability of S. oneidensis JG3355 to respire metal could be the result of losing the bacterial viability as was reported before29–31 and presented in Fig. 7A.
2.12 Enhancement of respiration of Cr6+ by Shewanella in the presence of selected materials
The respiration of Cr6+ (at sub-lethal concentration) was improved in the tested groups supplied by citrate alone (only 0.83 folds), as illustrated in Fig. 7B. Bencheikh-Latmani et al.87 explained a similar observation as a result of the possible complexation between the product of bio-reduction (Cr3+) and citrate, which consequently limits the availability of the toxic metal to bacterial cells.87
In the presence of a sublethal concentration of Cr6+, the alive cell extent was the highest in the group of citrate coated Mn0.22+Fe2.83+O4, Fe3O4 and Mn3O4 (in descending order). The presence of Mn0.22+Fe2.83+O4 as adsorbent was beneficial to microbial survival, which was positively related to enhanced Cr6+ bio-reduction by 2.5–3.6 folds. The increase in the percentage of bacterial viability may be attributed to the adsorption of Cr6+ by NPs, which led to the decrease of stress on the strains themselves. In addition, the possible continuous adsorption–desorption rate of Cr6+ was based on Langmuir adsorption isotherm of the equilibrium between the adsorbate and adsorbent system (Fig. 5C–E).
The presence of manganese in the chemical structure of NPs improved the antioxidant activity and, in turn, the viability of cells and the ability to respire metal.99 Mn2+ ions can act as antioxidants which helps enzymatic systems to act against oxidative stress. For Fe-rich and Mn-poor cells such as S. oneidensis MR-1, death at low doses of ionizing radiation might not be caused by DNA damage inflicted during irradiation but instead by the release of Fe2+ and the subsequently formed toxic by-product of energy-metabolism after irradiation.100
The electron transfer from cells to the acceptor101 occurred via redox cycling of the electron-donating and accepting functional groups via direct electron transfer through NPs. The affinity of MnFe2O4 NPs to bind proteins on the bacterial outer membrane can improve the contact area between a single bacterium cell and an external electron acceptor.37 There are some explanations for NP-enhanced bio-reduction of Cr6+ to Cr3+by S. oneidensis MR-1; however, the exact mechanism is not fully unravelled.102 NPs can act as a bridge between the bacterial cell and Cr6+ to promote electron transfer.102 Mn0.22+Fe2.83+O4 NPs can adsorb Cr6+via ion exchange40,103 and covalent bonding of Cr6+ on their surfaces.36 The adsorption of Cr6+ on the surface of NPs and its reduction to Cr3+ decreases the availability and toxicity of Cr6+, which improves the efficiency of microbial respiration.90,104 Since MnFe2O4 NPs have electrochemical properties,37,38 they can link S. oneidensis MR-1 with Cr6+ as an electron mediator from the cell to Cr6+, a terminal electron acceptor. In MnFe2O4, the existence of Mn and Fe in different oxidation states facilitates the redox processes on the NP surface.103 Finally, S. oneidensis MR-1 can reduce Fe3+of Mn0.22+Fe2.83+O4 NPs to Fe2+, which can further reduce Cr6+ to Cr3+.90 The bio-genic Fe2+ can reduce Cr6+ leading to releasing Fe3+ into the medium and the dissolution of NPs.88
3. Experimental section
3.1 Materials
All chemicals were used as received without further purification.
3.1.1 For nanoparticle synthesis.
Hydrochloric acid (HCl, 34%), and acetone (C3H6O, >99%), were bought from VWR Chemicals, UK. Anhydrous sodium hydroxide (NaOH, 98%), iron(III) acetylacetonate (Fe(acac)3, 99.9%), manganese(II) acetylacetonate (Mn(acac)2, 99.9%), manganese(III) acetylacetonate (Mn(acac)3, 99.9%), tetraethylene glycol (TEG, 99%), manganese(II,III) oxide (Mn3O4, 97%), manganese standard for inductively coupled plasma (ICP), TraceCERT®, 1000 mg L−1 Mn in HNO3, iron standard for ICP (TraceCERT®, 1000 mg L−1 Fe in HNO3), iron chloride tetrahydrate (FeCl2·4H2O ≥ 99%), nitric acid (HNO3, 70%), potassium bromide (KBr, FTIR grade 99%), tri-sodium citrate dihydrate (Na3C6H5O7·2H2O), hydroxylamine hydrochloride (ACS reagent, 98.0%), sodium acetate (Na-acetate, anhydrous, ReagentPlus®, ≥99.0%) and 1,10-phenanthroline monohydrate (titration ≥ 99.5%) were obtained from Sigma-Aldrich (UK). Absolute ethanol (C2H5 OH, 99.9%) was purchased from HaymanKimia, UK.
3.1.2 For Cr6+ adsorption.
1,5-Diphenylcarbazide (DPC, ≥98.0%) was obtained from Sigma-Aldrich (UK), and potassium dichromate (K2CrO4) was purchased from VWR Chemicals, UK.
3.1.3 For microbiological studies.
Both forward primer (1369F, CGGTGAATACGTTCYCGG) and reverse primer (1492R, GGWTACCTTGTTACGACTT) were obtained from Integrated DNA Technology (UK) in dry forms. Vitamin Supplement (ATCC® MD-VS™) and trace Mineral Supplement (ATCC® MD-TMS™) were procured from American Type Culture Collection (ATCC, UK). M9 minimal salts (2×) medium, Invitrogen Qubit assay kits, LIVE/DEAD BacLight Bacterial viability assay, and Invitrogen ultrapure DNase/RNase-free sterile distilled water were purchased from Thermo Fisher, UK. Luria–Bertani agar (LB) medium was supplied from Oxoid, UK. 4-(2-Hydroxyethyl)-1-piperazine-ethanesulfonic acid (HEPES, ≥99.5%), sodium DL-lactate (Na-lactate, ≥99.0%), and sodium fumarate dibasic (Na-fumarate, ≥99.0%) were obtained from Sigma-Aldrich (UK). FastDNA Spin Kit for Soil was purchased from MP Biomedicals, UK, and Luna Universal qPCR Master Mix was obtained from New England Biolabs, UK. Microbank™ cryobeads was purchased from Pro-Lab Diagnostics, UK.
3.1.4 Sources of bacteria of interest.
Freeze-dried cultures of Shewanella oneidensis MR-1 (strain number LMG 19005) were purchased from Belgian Coordinated Collections of Microorganisms/Laboratory for Microbiology of the Ghent University (BCCM/LMG). Shewanella loihica PV-4 (strain number DSMZ 17748) was obtained from Leibniz-Institut DSMZ-Deutsche Sammlung von Mikroorganismen und Zellkulturen GmbH and used as a positive biological control for microbial reduction of Cr6+. S. oneidensis JG1486 and JG3355 were kindly provided as LB agar stabs cryovials by Professor Jeffrey A. Gralnick at University of Minnesota, USA and used as negative biological controls. The dried bacteria were recovered via streaking on LB agar plate and incubated at 37 °C for 18 h. Colonial growth from all cultures was stored after being preserved on cryobeads at −20 °C.
3.2 Synthetic methodology
MnxFe3−xO4 nanostructures (NS) were prepared by a polyol solvothermal synthetic procedure42,49 with some modifications. The impact of the oxidation state of Mn precursors, i.e. Mn(acac)2 and Mn(acac)3, the molar ratio between [Mn precursor] to [Fe(acac)3] and reaction temperature on the nanoparticle properties were studied. Based on previous experience from our research group, using 15 wt%/vol as a total dissolved precursor concentration resulted in NPs with narrow size distribution.46
3.2.1 Synthesis of MnxFe3−xO4 NPs.
The desired amounts of precursors with ratios [Mn(acac)2 or 3]/[Fe(acac)3] equal to 0, 0.14, 0.33, 0.6, 1, 1.66 and 3 were mixed in 20 mL of TEG as a solvent. The resulting mixture was processed by vortexing for 10 min, then sonicated for 30 min to be homogenized, followed by its transfer into a 45 mL Teflon-lined stainless-steel autoclave. The autoclave was placed in an oven (Memmert, model UFP400) at room temperature, and the reaction temperature was raised for 30 min to 250 °C, which was maintained for 6 h. For the ratio [Mn(acac)2 or 3]/[Fe(acac)2] equal to 0 and 0.33, the temperature was raised up to 200 °C only. The resulting black dispersion was separated by a magnet and washed with 1
:
10 v/v of acetone, followed by ethanol and water three times for each solvent. Then, the nanomaterials were ready for characterization and functionalization.
3.2.2 Synthesis of MnxFe3−xO4 NFs.
MnxFe3−xO4 NFs were prepared following the above-described protocol for MnxFe3−xO4 NPs and literatures54 with some modifications. The autoclave was inserted in the oven, which was heated up to 200 °C for 6 h, and the tested ratios between precursors [Mn(acac)3]/[Fe(acac)3] were 1 and 3 while [Mn(acac)2 or 3]/[Fe(acac)3] ratio of 7 was kept at 250 °C for 6 h.
3.2.3 Functionalization of MnxFe3−xO4 NS.
In order to exchange the initial ligand TEG, 1 mL of the dispersions of the prepared nanomaterials and 10 mL of 1 M aqueous tri-sodium citrate solution was mixed for 48 h at room temperature under stirring. Immobilization of citrate on the surface of the commercially available Mn3O4 (used as Mn-rich and iron-free ferrite control) was carried out by dispersing 0.1 g of the metal oxide in 10 mL of 1 M aqueous tri-sodium citrate solution under similar mentioned conditions. The functionalized nanostructures were purified by magnetic separation, followed by washing with acetone three times, after that ethanol washing was performed three times, and finally the particles were dispersed in de-ionized water.
3.2.4 Characterization of MnxFe3−xO4 NS.
For the prepared nanomaterials, the shape and diameter of the core were determined by a JEOL JEM 1200-EX microscope operating at an acceleration voltage of 120 kV. The crystal phase and the average crystallite size were analyzed by X-ray diffractometer (XRD; PANalytical XPERT PRO MPD) coupled with Co Kα radiation source (λ = 1.789 Å) and an X'Celerator detector operated at 40 kV and 40 mA. The crystalline phases were identified using the International Centre for Diffraction Data Powder Diffraction File (ICDD PDF) database. The crystal domain size (DXRD) was calculated using Scherrer's equation at the most intense X-ray peaks (311). The chemical composition of MnxFe3−xO4 was determined by an Optima 3100 XL PerkinElmer Inductively Coupled Plasma Atomic Emission (ICP-AES) spectrometer. The oxidation states of Mn and Fe in selected MnxFe3−xO4 NPs that produce the lowest and the highest Qe were analysed by X-ray photoelectron spectrometry (XPS), a Kratos Analytical AXIS Ultra DLD system with aluminium monochromatic X-ray source (AlKα = 1486.6 eV), under ultra-high vacuum conditions (10−9 Torr). The experimental curves were best fitted by a combination of Gaussian (70%) and Lorentzian (30%) distributions. Over the range 150–2000 cm−1, Raman spectra were collected for powder samples of the selected MnxFe3−xO4 materials prepared using [Mn(acac)2]/[Fe(acac)3] equal to 0 and 0.33 at 250 °C for 6 h. A Renishaw InVia micro-Raman spectrometer was used and experiments were conducted at room temperature and excited by green Ar-laser for excitation (λ = 514.5 nm) of photon energy 2.4 eV and diffraction grating 2400 grating.
3.2.5 Surface characterization of MnxFe3−xO4 NS.
In order to study the surface coordination of the capping agents, a PerkinElmer FTIR; Spectrum 100 instrument with a Ge/Ge universal attenuated total reflectance (ATR) was used. The samples were prepared by air drying at room temperature overnight to yield a fine powder and then directly placed on an ATR crystal. The measurement window for the recorded spectra was in the range 4000–600 cm−1, with a 2 cm−1 resolution, using 40 scan accumulation. The hydrodynamic diameter (DHD) of citrate-functionalized NPs was evaluated by DLS measurements performed with a Nanosizer ZS instrument (He–Ne 633 nm laser) from Malvern Instruments Ltd, Worcestershire, UK). The ζ-potentials of the functionalized NPs were determined using a disposable capillary cell (DTS1070) at 25 °C by DLS. For iron content quantification of the functionalized nanomaterials dispersed in water, a colourimetric phenanthroline method was applied for the acid-digested tested agent.105 The concentration of Mn in the Mn3O4 dispersion was estimated by inductively coupled plasma atomic emission spectroscopy (ICP-AES) spectrometer.
3.2.6 Measurement of the Cr6+ adsorption capacity of nanostructures.
Equal volumes of aqueous dispersed citrate-coated nanostructures (adsorbents) and Cr6+ aqueous solution were mixed and incubated for 6 h at pH 7 at room temperature. Both citrate-capped Fe3O4 NPs and Mn3O4 NPs were used as the control group, and 0.01 M of trisodium citrate served as a background. The amounts of adsorbed Cr6+ per unit mass of adsorbent (Qe; mg g−1) were calculated using eqn (1): | 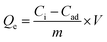 | (1) |
Ci and Cad were the initial concentration of Cr6+, which was equal to 30 mg L−1, and the concentration of Cr6+ in the solution at the equilibrium, respectively. The total volume of the reactants mixture (V) was 2 mL, and the mass of adsorbents (m) was represented in g with respect to Mn mass fractions. The concentration of Cr6+ was quantified by measuring the optical density of the colour generated by the Cr3+ DPC (diphenylcarbazide) complex method at λ 545 nm.106,107
The adsorption isotherms of Cr6+ by the selected Mn0.22+Fe2.83+O4 NPs were studied due to their superior Qe. The isotherm was measured at room temperature by varying the initial Cr6+ concentration from 10–250 mg L−1 at pH 7 for 6 h contact time. The concentration of the adsorbents was adjusted to 1 mg mL−1. For Mn0.22+Fe2.83+O4, the mass of the adsorbent was calculated in respect to both Fe and Mn fractions which were 0.68 and 0.05, respectively. Adsorption isotherms were fitted by both Langmuir and Freundlich models.108
3.2.7 Bacteria identification.
The genome DNA of bacteria was extracted by boiling a single colony in ultra-pure water for 10 min at 95 °C. To deliver the highest DNA yield from the tested colony, FastDNA Spin Kit for Soil was applied to the boiled broth following the manufacturer's instructions. Using a Qubit 3.0 fluorometer (Life Technologies, UK), the quantity of DNA was evaluated using Qubit dsDNA broad range (2 to 1000 ng) assay kit from Invitrogen (UK). 16S rRNA genes of all strains were amplified by polymerase chain reaction (PCR) using pair primers; 1369F and 1492R primers and Luna Universal qPCR Master Mix.109 Using thermocycler (Cole-Parmer), cycling conditions included initial denaturation at 94 °C for 5 min, followed by 30 cycles of denaturation at 94 °C for 45 s, heating at 52 °C for 45 s and extension at 72 °C for 90 s. The final extension was tested at 72 °C for 90 s. Both the purification of amplified PCR products and Sanger sequencing were implemented using the commercial service of Source Bioscience, Cambridge, UK. The sequenced data were assigned for matching identity for species with the highest fitting 96–100% by nucleotide BLAST (Basic Local Alignment Search Tool) from the National Centre for Biotechnology Information (NCBI) database (https://blast.ncbi.nlm.nih.gov).
3.2.8 Minimum inhibition concentration of Cr6+.
To assess the impact of Cr6+ on the viability of the tested Shewanella, a Guava easyCyte® flow cytometer (Merck, UK) was used. 10 μL of homogeneous bacterial cell suspensions with OD measured at the wavelength of 600 nm equal to 0.1 was added to 80 μL of M9 minimal salts (2×) medium.110 This medium was supplemented by 20 mM sodium lactate as a sole electron source, 5 mL L−1 each of vitamins and minerals and pH was adjusted to 7.2 by 10 mM HEPES buffer.111 The viability of cells was counted in response to serial dilutions of Cr6+ (1 to 100 mg L−1) as a terminal electron source alone. Sodium fumarate (20 mM) was used as an alternative terminal electron acceptor to Cr6+. In all cases, media were purged with nitrogen gas for 5 min after bacterial inoculation. The proportion of live cells was quantified in relation to the total number of cells via the Live Dead BacLight Bacterial viability assay. Populations of living and/or dead bacteria were gated according to fluorescence minus one (FMO) controls using single stains of SYTO9 and propidium iodide (PI).112 All data are expressed as means ± standard deviation. The MIC of any agent was defined as its lowest concentration that inhibits the growth of bacteria after overnight incubation.
3.2.9 Effect of the Mn0.22+Fe2.83+O4 NPs on the bio-removal of Cr6+.
Measuring the impact of the tested NPs on the bio-removal of Cr6+ efficiency was tested at 50 mg L−1 as a sub-lethal dose of Cr6+ for both S. oneidensis and S. loihica while using 10 and 1 mg L−1 of Cr6+ for the biological controls (S. oneidensis JG1486 and JG3355, respectively). The concentration of citrate was 0.1 mM, and citrate-coated Fe3O4, Mn0.22+Fe2.83+O4 NPs, MnFe2O4 NFs and Mn3O4 NPs were adjusted to be in the range of 1 mg mL−1. The remaining soluble Cr6+ in the supernatant (Crs) was filtered through a 0.45 μm membrane and quantified using Cr3+–DPC complex method.106,107 The percentage of Cr6+ bio-reduction was calculated in relation to the initial concentration (Cri) of Cr6+ following eqn (2):26,81 | 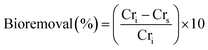 | (2) |
4. Conclusions
Adsorption of hexavalent chromium (Cr6+) on manganese ferrite (MnxFe3−xO4) nanostructures enhanced the bio-detoxification of Cr6+ by S. oneidensis MR-1. A synthetic platform for achieving the most suitable chemical structure of MnxFe3−xO4 nanoparticles (NPs) and nanoflowers (NFs) acting as Cr6+ adsorption agents was presented. At 250 °C, both divalent or trivalent manganese precursors formed spherical NPs, whereas, at 200 °C, nanoflowers were obtained using a trivalent precursor. Mn0.22+Fe2.83+O4 NPs that were prepared from divalent manganese precursor showed the highest Cr6+ adsorption capacity (16.8 ± 1.6 mg g−1) and led to 3.3 times improvement in the viability of S. oneidensis MR-1 in the presence of Cr6+ and 2.66 times an enhancement in Cr6+ bio-detoxification. This will open up a new venue of research using nanomaterials for boosting the bio-reduction of Cr6+ using bacteria.
Author contributions
N. T. K. T. and L. C. devised and coordinated the project and provided resources. D. S. R. designed and did most of the experiments and wrote the manuscript. I. T. assisted in particle synthesis and data analysis. S. A., P. W. and M. C. helped with the microbiology work. N. T. K. T. and S. M. provided expertise, corrected the manuscript and helped to acquire funding. K. S., A. M. and D. K. carried out XPS characterization, processed data and corrected the manuscript. G. V. provided resources for characterization. L. B. helped to acquire funding.
Conflicts of interest
The authors declare no competing financial interest.
Acknowledgements
D. S. R. acknowledges funding from Newton Mosharafa scholarship given to Egyptian Petroleum Research Institute. The authors acknowledge Jeffrey A. Gralnick at University of Minnesota, for kindly providing S. oneidensis JG1486 and JG3355. The authors would like to thank EPSRC (EP/M015157/1) for financial support. We thank UCL Grand Challenges and UCL Small Grant for funding. Adam Strange was acknowledged for participation in writing UCL Small Grant application. We thank Ana Alvarez Prendes for contributing in the data analysis of some TEM images. Thithawat Trakoolwilaiwan is acknowledged for the ICP analysis. Rachel Duan is thanked for some functionalization of NPs. We acknowledge Chris Howard and Szymon Bartus at UCL, for using Raman spectroscopy facility.
Notes and references
- A. S. Jones, J. Marini, H. M. Solo-Gabriele, N. M. Robey and T. G. Townsend, Waste Manage., 2019, 87, 731–740 CrossRef CAS PubMed
.
- S. Famielec, Materials, 2020, 13, 1533–1545 CrossRef CAS PubMed
.
- B. Wang, B. Liu, J. Gu and M. A. J. Somers, Surf. Coat. Technol., 2022, 438, 128408–128422 CrossRef CAS
.
- H. Tang, Z. Peng, F. Gu, L. Yang, W. Tian, Q. Zhong, M. Rao, G. Li and T. Jiang, Ceram. Int., 2021, 47, 10809–10818 CrossRef CAS
.
- J. Xing and M. T. Pailthorpe, J. Soc. Dyers Colour., 2000, 116, 91–93 CAS
.
- Z. Wang, C. Bao, K. Yan, Y. Song and W. Li, J. Appl. Polym. Sci., 2021, 138, 1–10 Search PubMed
.
- A. Sennaroglu and Y. Morova, Appl. Phys. B: Lasers Opt., 2022, 128, 1–25 CrossRef
.
- A. Bratovcic, H. Buksek, C. Helix-Nielsen and I. Petrinic, Chem. Eng. J., 2022, 431, 133918–133927 CrossRef CAS
.
- V. Bolaños-Benítez, E. D. van Hullebusch, J. L. Birck, J. Garnier, P. N. L. Lens, M. Tharaud, C. Quantin and Y. Sivry, Chem. Geol., 2021, 561, 120000–120011 CrossRef
.
- M. A. Islam, M. J. Angove and D. W. Morton, Environ. Nanotechnol., Monit. Manage., 2019, 12, 100267–100287 Search PubMed
.
-
International Agency for Research on Cancer, List of Classifications–IARC Monographs on the Identification of Carcinogenic Hazards to Humans, 2020, vol. 1 Search PubMed
.
- N. A. Awang, W. N. W. Salleh, A. F. Ismail, N. Yusof, F. Aziz and J. Jaafar, Ind. Eng. Chem. Res., 2019, 58, 720–728 CrossRef CAS
.
- A. D. Villalobos-lara, F. Alvarez, Z. Gami, R. Navarro and J. M. Peralta-hern, Chemosphere, 2021, 264, 128491–128499 CrossRef CAS PubMed
.
- F. I. El-Dib, D. E. Mohamed, O. A. A. El-Shamy and M. R. Mishrif, Egypt. J. Pet., 2020, 29, 1–7 CrossRef
.
- Z. Q. Id, W. Dong, Y. Chen, G. Dong, S. Zhu, Y. Yu and D. Bian, PLoS One, 2020, 15, 1–17 Search PubMed
.
- V. E. Pakade, T. Tavengwa and L. M. Madikizela, RSC Adv., 2019, 9, 26142–26164 RSC
.
- A. B. Mpofu, O. O. Oyekola and P. J. Welz, J. Cleaner Prod., 2021, 296, 126490–126504 CrossRef CAS
.
- R. Hern, V. Y. Mena-cervantes, E. Ruiz-baca, E. E. Neri-torres, I. Chairez, S. M. García-solares and J. Vazquez-arenas, J. Environ. Chem. Eng., 2021, 9, 104626–104637 CrossRef
.
- G. Wu, F. Wan, H. Fu, N. Li and H. Gao, J. Bacteriol., 2015, 197, 3563–3572 CrossRef CAS PubMed
.
- L. Cheng, R. He, D. Min, W. Li, D. Liu and H. Yu, ACS ES&T Engg, 2021, 1, 842–850 Search PubMed
.
- F. Asdrubali, F. D. Alessandro and S. Schiavoni, Sustainable Mater. Technol., 2020, 4, 1–17 Search PubMed
.
- A. Zuazua-ros, M. Vidaurre-arbizu and P. Silvia, J. Cleaner Prod., 2021, 291, 125960–125971 CrossRef
.
- M. Naveenkumar and K. Senthilkumar, Biomass Bioenergy, 2021, 149, 106082–106089 CrossRef CAS
.
- H. Gang, C. Xiao, Y. Xiao, W. Yan, R. Bai, R. Ding, Z. Yang and F. Zhao, Environ. Int., 2019, 127, 94–102 CrossRef CAS PubMed
.
- A. Elahi and A. Rehman, J. King Saud Univ., Sci., 2019, 31, 1005–1013 CrossRef
.
- D. L. Parker, P. Borer and R. Bernier-Latmani, Front. Microbiol., 2011, 2, 1–14 Search PubMed
.
- M. Kheirabadi, R. Mahmoodi, N. Mollania and M. Kheirabadi, Int. J. Environ. Sci. Technol., 2020, 17, 143–152 CrossRef CAS
.
- X. Tang, Y. Huang, Y. Li, L. Wang, X. Pei, D. Zhou, P. He and S. S. Hughes, Ecotoxicol. Environ. Saf., 2021, 208, 111699–111711 CrossRef CAS PubMed
.
- A. Carra, P. W. Villalta, J. He, X. Yao, R. J. Hamers, S. Balbo, Z. Vivian and C. L. Haynes, Chem. Sci., 2020, 11, 11244–11258 RSC
.
- S. L. Mitchell, N. V Hudson-Smith, M. S. Cahill, B. N. Reynolds, S. D. Frand, C. M. Green, C. Wang, M. N. Hang, R. T. Hernandez and R. J. Hamers, Chem. Sci., 2019, 10, 9768–9781 RSC
.
- M. N. Hang, I. L. Gunsolus, H. Wayland, E. S. Melby, A. C. Mensch, K. R. Hurley, J. A. Pedersen, C. L. Haynes and R. J. Hamers, Chem. Mater., 2016, 28, 1092–1100 CrossRef CAS
.
- H. Dong, L. Li, Y. Lu, Y. Cheng, Y. Wang, Q. Ning, B. Wang, L. Zhang and G. Zeng, Environ. Int., 2019, 124, 265–277 CrossRef CAS PubMed
.
- C. Qu, S. Qian, L. Chen, Y. Guan, L. Zheng, S. Liu, W. Chen, P. Cai and Q. Huang, Environ. Sci. Technol., 2019, 53, 8147–8156 CrossRef CAS PubMed
.
- K. Zhang, N. Li, P. Liao, Y. Jin, Q. Li, M. Gan, Y. Chen, P. He, F. Chen, M. Peng and J. Zhu, Environ. Pollut., 2021, 286, 117227–117236 CrossRef CAS PubMed
.
- A. Sundman, A. L. Vitzthum, K. Adaktylos-surber, A. I. Figueroa, G. van der Laan, B. Daus, A. Kappler, J. M. Byrne, G. Van Der Laan, B. Daus, A. Kappler and J. M. Byrne, J. Hazard. Mater., 2020, 384, 121450–121456 CrossRef CAS PubMed
.
- B. Eyvazi, A. Jamshidi-zanjani and A. Khodadadi, Environ. Pollut., 2020, 265, 113685–113695 CrossRef CAS PubMed
.
- Y. Ma, X. Wu, Z. Shi, X. Li, S. Qian, X. Sun, W. Sun, C. Guo and C. M. Li, ACS Sustainable Chem. Eng., 2022, 10, 3355–3362 CrossRef CAS
.
- S. Khilari, S. Pandit, J. L. Varanasi, D. Das and D. Pradhan, ACS Appl. Mater. Interfaces, 2015, 7, 20657–20666 CrossRef CAS PubMed
.
- J. Hu, I. M. C. Lo and G. Chen, Sep. Purif. Technol., 2007, 56, 249–256 CrossRef CAS
.
- J. Hu, I. M. C. Lo and G. Chen, Langmuir, 2005, 21, 11173–11179 CrossRef CAS PubMed
.
- M. Li, Q. Gao, T. Wang, Y. S. Gong, B. Han, K. S. Xia and C. G. Zhou, Mater. Des., 2016, 97, 341–348 CrossRef CAS
.
- X. Lasheras, M. Insausti, J. M. De La Fuente, I. Gil De Muro, I. Castellanos-Rubio, L. Marcano, M. L. Fernández-Gubieda, A. Serrano, R. Martín-Rodríguez, E. Garaio, J. A. García and L. Lezama, Dalton Trans., 2019, 48, 11480–11491 RSC
.
-
O. Antonoglou and C. Dendrinou-Samara, in Reducing Agents in Colloidal Nanoparticle Synthesis, 2021, pp. 51–72 Search PubMed
.
- N. T. K. Thanh, N. Maclean and S. Mahiddine, Chem. Rev., 2014, 114, 7610–7630 CrossRef CAS PubMed
.
- D. García-Soriano, R. Amaro, N. Lafuente-Gómez, P. Milán-Rois, Á. Somoza, C. Navío, F. Herranz, L. Gutiérrez and G. Salas, J. Colloid Interface Sci., 2020, 578, 510–521 CrossRef PubMed
.
- R. Hachani, M. Lowdell, M. Birchall, A. Hervault, D. Mertz, S. Begin-Colin and N. T. B. D. K. Thanh, Nanoscale, 2016, 8, 3278–3287 RSC
.
- D. S. Mathew and R. S. Juang, Chem. Eng. J., 2007, 129, 51–65 CrossRef CAS
.
- H. Sharifi Dehsari, V. Ksenofontov, A. Möller, G. Jakob and K. Asadi, J. Phys. Chem. C, 2018, 122, 28292–28301 CrossRef
.
- K. Vamvakidis, M. Katsikini, G. Vourlias, M. Angelakeris, E. C. Paloura and C. Dendrinou-Samara, Dalton Trans., 2015, 44, 5396–5406 RSC
.
- S. A. Ahmed, Results Phys., 2017, 7, 604–610 CrossRef
.
- L. Khanna and N. K. Verma, Phys. B, 2013, 427, 68–75 CrossRef CAS
.
- F. Branda, A. Buri, A. Marotta and S. Saiello, Thermochim. Acta, 1984, 93, 65–68 Search PubMed
.
- K. Vamvakidis, D. Sakellari, M. Angelakeris and C. Dendrinou-Samara, J. Nanopart. Res., 2013, 15, 1743–1753 CrossRef
.
- S. Fu, R. Yang, J. Ren, J. Liu, L. Zhang, Z. Xu, Y. Kang and P. Xue, ACS Nano, 2021, 15, 11953–11969 CrossRef CAS PubMed
.
- J. Ge, Y. Hu, M. Biasini, W. P. Beyermann and Y. Yin, Angew. Chem., Int. Ed., 2007, 46, 4342–4345 CrossRef CAS PubMed
.
- H. Gavilán, E. H. Sánchez, M. E. F. Brollo, L. Asín, K. K. Moerner, C. Frandsen, F. J. Lázaro, C. J. Serna, S. Veintemillas-Verdaguer, M. P. Morales and L. Gutiérrez, ACS Omega, 2017, 2, 7172–7184 CrossRef PubMed
.
- Y. Xie, C. Tian, W. Chen, C. Wu, Z. Liu, P. Ning, H. Deng and Z. Lin, Environ. Sci.: Nano, 2019, 6, 1406–1417 RSC
.
- S. Xuan, F. Wang, Y. J. Wang, C. Yu and K. C. Leung, J. Mater. Chem., 2010, 20, 5086–5094 RSC
.
- A. Qureashi, A. H. Pandith, A. Bashir, T. Manzoor, L. A. Malik and F. A. Sheikh, Surf. Interfaces, 2021, 23, 101004–101019 CrossRef CAS
.
- I. Chakraborty, D. Majumder, S. Talukdar, S. Roy and K. Mandal, Surf. Interfaces, 2017, 9, 154–159 CrossRef CAS
.
- D. Wyrzykowski and L. Chmurzyński, J. Therm. Anal. Calorim., 2010, 102, 61–64 CrossRef CAS
.
- M. Matzapetakis, N. Karligiano, A. Bino, M. Dakanali, C. P. Raptopoulou, V. Tangoulis, A. Terzis, J. Giapintzakis and A. Salifoglou, Inorg. Chem., 2000, 39, 4044–4051 CrossRef CAS PubMed
.
- M. Justi, M. P. de Freitas, J. M. Silla, C. A. Nunes and C. A. Silva, J. Mol. Struct., 2021, 1237, 130405–130417 CrossRef CAS
.
- S. Luther, N. Brogfeld, J. Kim and J. G. Parsons, J. Colloid Interface Sci., 2013, 400, 97–103 CrossRef CAS PubMed
.
- W. G. Gao, X. C. Liu and M. F. Chen, RSC Adv., 2017, 7, 41011–41016 RSC
.
- Y. Cantu, A. Remes, A. Reyna, D. Martinez, J. Villarreal, H. Ramos, S. Trevino, C. Tamez, A. Martinez, T. Eubanks and J. G. Parsons, Chem. Eng. J., 2014, 254, 374–383 CrossRef CAS PubMed
.
- H. Kumar, K. L. Maurya, A. K. Gehlaut, D. Singh, S. Maken, A. Gaur and S. Kamsonlian, Appl. Water Sci., 2020, 10, 1–10 CrossRef
.
- K. Ahalya, N. Suriyanarayanan and V. Ranjithkumar, J. Magn. Magn. Mater., 2014, 372, 208–213 CrossRef CAS
.
- S. Martinez-Vargas, A. I. Martínez, E. E. Hernández-Beteta, H. H.-F. O. F. Mijangos-Ricardez, V. Vázquez-Hipólito, C. Patiño-Carachure and J. López-Luna, J. Mater. Sci., 2017, 52, 6205–6215 CrossRef CAS
.
- J. Wang, Q. Xu, W. Yin, J. Hou, S. Wang and X. Wang, Ecotoxicol. Environ. Saf., 2021, 217, 112209–112216 CrossRef CAS PubMed
.
- Y. Li, G. Wei, C. Zhang, X. Liang, W. Chu, H. He, J. W. Stucki, L. Ma, X. Lin and J. Zhu, Sci. Total Environ., 2019, 656, 400–408 CrossRef CAS PubMed
.
- J. Zhang, C. Zhang, G. Wei, Y. Li, X. Liang, W. Chu, H. He, D. Huang, J. Zhu and R. Zhu, J. Colloid Interface Sci., 2017, 500, 20–29 CrossRef CAS PubMed
.
- N. Antonatos, D. Bouša, S. Shcheka, S. M. Beladi-Mousavi, M. Pumera and Z. Sofer, Inorg. Chem., 2019, 58, 10227–10238 CrossRef CAS PubMed
.
- M. Testa-Anta, M. A. Ramos-Docampo, M. Comesaña-Hermo, B. Rivas-Murias and V. Salgueiriño, Nanoscale Adv., 2019, 1, 2086–2103 RSC
.
- M. C. Biesinger, B. P. Payne, A. P. Grosvenor, L. W. M. Lau, A. R. Gerson and R. S. C. Smart, Appl. Surf. Sci., 2011, 257, 2717–2730 CrossRef CAS
.
- Z. Zhang, Y. Wang, Q. Tan, Z. Zhong and F. Su, J. Colloid Interface Sci., 2013, 398, 185–192 CrossRef CAS PubMed
.
- I. Desai, M. N. Nadagouda, M. Elovitz, M. Mills and B. Boulanger, Mater. Sci. Energy Technol., 2019, 2, 150–160 Search PubMed
.
- T. Yamashita and P. Hayes, Appl. Surf. Sci., 2008, 254, 2441–2449 CrossRef CAS
.
- J. Han, M. Kim and H. M. Ro, Environ. Chem. Lett., 2020, 18, 631–662 CrossRef CAS
.
- J. Xie, X. Gu, F. Tong, Y. Zhao and Y. Tan, J. Colloid Interface Sci., 2015, 455, 55–62 CrossRef CAS PubMed
.
- C. Ri, J. Tang, F. Liu, H. Lyu and F. Li, J. Environ. Sci., 2022, 113, 12–25 CrossRef CAS PubMed
.
- G. Wang, B. Zhang, S. Li, M. Yang and C. Yin, Bioresour. Technol., 2017, 227, 353–358 CrossRef CAS PubMed
.
- A. Mohamed, L. Yu, Y. Fang, N. Ashry, Y. Riahi, I. Uddin, K. Dai and Q. Huang, Chemosphere, 2020, 247, 125902–125914 CrossRef CAS PubMed
.
- D. Coursolle and J. A. Gralnick, Front. Microbiol., 2012, 3, 1–11 Search PubMed
.
- K. Chourey, M. R. Thompson, J. Morrell-Falvey, N. C. VerBerkmoes, S. D. Brown, M. Shah, J. Zhou, M. Doktycz, R. L. Hettich and D. K. Thompson, Appl. Environ. Microbiol., 2006, 72, 6331–6344 CrossRef CAS PubMed
.
- B. D. Bennett, K. E. Redford, J. A. Gralnick and A. Gralnick, J. Bacteriol., 2018, 200, 1–14 CrossRef CAS PubMed
.
- R. Bencheikh-Latmani, A. Obraztsova, M. R. Mackey, M. H. Ellisman and B. M. Tebo, Environ. Sci. Technol., 2007, 41, 214–220 CrossRef CAS PubMed
.
- X. Liu, G. Chu, Y. Du, J. Li and Y. Si, World J. Microbiol. Biotechnol., 2019, 35, 1–8 CrossRef CAS PubMed
.
- S. Viamajala, B. M. Peyton, R. K. Sani, W. A. Apel and J. N. Petersen, Biotechnol. Prog., 2004, 20, 87–95 CrossRef CAS PubMed
.
- A. Mohamed, B. Sun, C. Yu, X. Gu, N. Ashry, Y. Riahi, K. Dai and Q. Huang, J. Environ. Chem. Eng., 2021, 9, 105096–105106 CrossRef CAS
.
- R. Elmeihy, X. C. Shi, P. L. Tremblay and T. Zhang, Chemosphere, 2021, 263, 128281–128289 CrossRef CAS PubMed
.
- J. Cheng, J. Gao, J. Zhang, W. Yuan, S. Yan, J. Zhou, J. Zhao and S. Feng, Water, Air, Soil Pollut., 2021, 232, 1–14 CrossRef
.
- Y. Xiao, C. Xiao and F. Zhao, Front. Environ. Sci. Eng., 2020, 14, 1–11 CrossRef
.
- U. Schröder, Phys. Chem. Chem. Phys., 2007, 9, 2619–2629 RSC
.
- D. J. Richardson, J. N. Butt, J. K. Fredrickson, J. M. Zachara, L. Shi, M. J. Edwards, G. White, N. Baiden, A. J. Gates, S. J. Marritt and T. A. Clarke, Mol. Microbiol., 2012, 85, 201–212 CrossRef CAS PubMed
.
- J. S. McLean, P. D. Majors, C. L. Reardon, C. L. Bilskis, S. B. Reed, M. F. Romine and J. K. Fredrickson, J. Microbiol. Methods, 2008, 74, 47–56 CrossRef CAS PubMed
.
- J. A. Gralnick, H. Vali, D. P. Lies and D. K. Newman, Proc. Natl. Acad. Sci. U. S. A., 2006, 103, 4669–4674 CrossRef CAS PubMed
.
- G. J. Newton, S. Mori, R. Nakamura, K. Hashimoto and K. Watanabe, Appl. Environ. Microbiol., 2009, 75, 7674–7681 CrossRef CAS PubMed
.
- I. L. Gunsolus, M. N. Hang, N. V Hudson-Smith, J. T. Buchman, J. W. Bennett, D. Conroy, S. E. Mason, R. J. Hamers and C. L. Haynes, Environ. Sci.: Nano, 2017, 4, 636–646 RSC
.
- D. Ghosal, M. V. Omelchenko, E. K. Gaidamakova, V. Y. Matrosova, A. Vasilenko, A. Venkateswaran, M. Zhai, H. M. Kostandarithes, H. Brim, K. S. Makarova, L. P. Wackett, J. K. Fredrickson and M. J. Daly, FEMS Microbiol. Rev., 2005, 29, 361–375 CAS
.
- J. Qin, L. Qian, J. Zhang, Y. Zheng, J. Shi, J. Shen and C. Ou, Chemosphere, 2021, 263, 128048–128057 CrossRef CAS PubMed
.
- Y. Yin, C. Liu, G. Zhao and Y. Chen, J. Hazard. Mater., 2022, 440, 165187–165211 Search PubMed
.
- J. Hu, I. M. C. Lo and G. Chen, Sep. Purif. Technol., 2007, 56, 249–256 CrossRef CAS
.
- H. Cheng, Z. Jing, L. Yang, A. Lu, G. Ren and J. Liu, Geochim. Cosmochim. Acta, 2021, 305, 19–32 CrossRef CAS
.
- M. G. Fortune and W. B. Mellon, Ind. Eng. Chem., Anal. Ed., 1938, 10, 60–64 CrossRef
.
- A. Sanchez-Hachair and A. Hofmann, C. R. Chim., 2018, 21, 890–896 CrossRef CAS
.
- P. F. Urone, Anal. Chem., 1955, 27, 1354–1355 CrossRef CAS
.
- M. A. Al-ghouti and D. A. Da, J. Hazard. Mater., 2020, 393, 122383–122404 CrossRef CAS PubMed
.
- L. Xu, H. Chen, M. Canales and L. Ciric, J. Microbiol. Methods, 2019, 164, 105670–105676 CrossRef CAS PubMed
.
- N. Wurzler, J. David, R. Wagner, M. Dimper, D. Lützenkirchen-hecht and O. Ozcan, Corros. Sci., 2020, 174, 108855–108862 CrossRef CAS
.
- E. D. Kees, C. E. Levar, S. P. Miller, D. R. Bond, J. A. Gralnick and A. M. Dean, Commun. Biol., 2021, 4, 1–9 CrossRef PubMed
.
- C. Bankier, Y. Cheong, S. Mahalingam, M. Edirisinghe, G. Ren, E. Cloutman-Green and L. Ciric, PLoS One, 2018, 13, 1–13 CrossRef PubMed
.
|
This journal is © The Royal Society of Chemistry 2023 |