DOI:
10.1039/D2MD00320A
(Research Article)
RSC Med. Chem., 2023,
14, 433-443
Multi-drug loaded eugenol-based nanoemulsions for enhanced anti-mycobacterial activity†
Received
12th September 2022
, Accepted 22nd December 2022
First published on 19th January 2023
1. Introduction
Tuberculosis (TB) is still a significant global health issue even after the invention of various medications. Human TB is the second leading infectious killer of a single infectious agent, Mycobacterium tuberculosis, accounting for eight million new cases and 1.5 million deaths in the year 2020 as per the recent survey recorded by the WHO.1 According to 2021 figures, tuberculosis is the second leading cause of death in the category of infectious diseases after corona. This communicable disease is disseminated by contaminated aerosols that affect macrophages in the lungs, causing pulmonary tuberculosis, but it can also affect other organs, causing extrapulmonary tuberculosis.2,3 As a result, the development of contemporary antibacterial medications becomes a foregone conclusion to eradicate TB. This leads to the invention of a vast range of anti-TB medications ranging from first-line drugs like rifampicin (R), isoniazid (I), pyrazinamide (Z), and ethambutol (E) to the most recent next-generation drugs like bedaquiline.4,5 However, the bacteria's innate capacity to fend off antibacterial medications over time resulted in the re-emergence of more serious TB.6 Therefore, the need to find ways to minimize the growth of such multi-resistive bacteria is of paramount importance. Along with drug resistance, the cost and the adverse effect of these drugs on human health have also made their long-term administration difficult.7,8 This has brought a decline in the success rate of the current chemotherapy along with the realization of the potential of repurposing older drugs rather than the discovery of newer drugs.9,10 Several methods to combat tuberculosis have been reported, which included multiple drug therapies such as directly observed treatment short-course (DOTS) one of which is the intake of isoniazid (I) in combination with rifampicin (R), pyrazinamide (Z), and ethambutol (E) in a fixed dose as a combination therapy focusing on curing TB patients. However, some exclusive strains of TB such as MDR-TBs and XDR-TBs are ineffectively treated with moderate antibacterial medications, and TB transmission is becoming increasingly uncontrollable. Espinal M et al. through their work have provided evidence on the effect of a 1st line drug-based DOTS regimen in the reduction of MDR TB transmission.11 Thus, it is urged that we find other modes of medication to control the transmission and destruction of the TB bacterium. For better administration of drugs into the human body, the manner of delivery of drugs is also important. Several drug delivery systems have been invented including liposomes, proliposomes, microspheres, gels, prodrugs, cyclodextrin assisted systems, and nanoparticle assisted systems.12 Nanoparticle assisted systems can be coated nanoparticles, pegylated nanoparticles, solid lipid nanoparticles (SLN), and nanogels. Nonetheless, sometimes the toxicity and cell viability of the system matter during drug loading as well as its release into the targeted cell. Nano-emulsions are another kind and emerging drug delivery system because of their peculiar features like high solubility of the drug concerned, nontoxicity, biocompatibility, and formation of monodisperse stable emulsion droplets to meet the FDA (Food and Drug Administration) guidelines.13,14 Such nano-emulsions are composed of a hydrophilic phase and a hydrophobic phase where one is dispersed through the other, which normally involves either the dispersion of oil into the water or water droplet dispersion through oil to completely form a monodisperse system.15–17 The involvement of natural oils and water prevents the chances of being toxic; also, both the lipophilic and hydrophilic nature of the water–oil system makes it biocompatible in itself.18 Generally natural sources are considered to be potential antibiotic agents.19,20 Natural sources also include essential oils which are known for their chemical diversity and are widely used for their anti-inflammatory, anti-microbial and even anti-cancer action.21,22 The anti-mycobacterial activities of very few essential oils are explored as of now.23–25 Besides their improved bioavailability due to the increased interfacial area of individual nanosized droplets, the site-specific release of incorporated drugs into the alveolar region of human beings,26 the drug delivery of hydrophobic drugs, even the combination of drugs into the human body, etc. are also plus points in choosing nano-emulsions.27 Multiple reports have been published suggesting the use of a nanoparticle-assisted drug delivery system against TB by demonstrating the efficacy of drug delivery through various administration routes.28 Muttil et al. exemplified the benefits of inhaled drug delivery to conquer TB in one of their studies.29 A study of a range of medications encapsulated in poly(DL-lactide-co-glycolide) (PLG) for tuberculosis, including ATD, I, R, and Z, as well as optimized oral and inhalable delivery methods, concluded that aerosol systems are not as bioavailable as injectable systems.30 A surface-modified 4.0 G PAMAM dendrimer has been studied by Ahmed et al. as a potential carrier system loaded with rifampicin to treat tuberculosis, where rifampicin was encapsulated into dendrimers using a simple dissolution–solvent evaporation process.31 Chen et al. disclosed carbon nanotube-mediated isoniazid release in which the cytotoxicity was managed and reduced by the addition of chitosan.32 Nonetheless, toxicity studies should have been implemented for the betterment of designing drug delivery systems and this becomes a dragging element in the incorporation of such drug delivery systems. The additional functionalization with cell viable molecules also complexifies their synthesis and administration. The conventional nontoxicity of nano-emulsions can be utilized to extend their use in more areas including drug delivery.33
Herein, we intended to emphasize on the reformulation of 1st line anti-tuberculosis drugs such as rifampicin, isoniazid, pyrazinamide and ethambutol by preparing a stable nano-emulsion system of different eugenol based essential oils using central composite design (CCD), thus improving its ability to inhibit the action of MDR strains of Mycobacterium tuberculosis and also exhibit excellent biocompatibility and nontoxicity. We also studied the drug release kinetics of this combinational nano based drug delivery system for the controlled and targeted release of the aforementioned drugs.
2. Experimental methods
2.1. Chemicals and microorganisms
Rifampicin, ethambutol, pyrazinamide, and isoniazid were purchased from HiMedia Laboratories, Mumbai. Eugenol, cinnamon leaf essential oil, and clove essential oil, as well as the non-ionic surfactants Tween 20 (TW20) and Tween 80 (TW80) were supplied by Sigma-Aldrich Chemicals Private Limited, Bengaluru. Middlebrook medium was the culture medium used for culturing Mycobacterium tuberculosis and it was handled by a microbiologist at the National TB Centre, Chennai. All these chemicals were used without further purification as they were of analytical grade. All the studies were carried out with ultrapure water condensed from a CascadaTM BIO-water purifier (Pall India Pvt. Ltd, India) with a resistance of 18.3 M cm−1.
Solubility studies: since rifampicin is the only hydrophobic of the four drugs, its solubility in the selected essential oils and surfactants was determined using the equilibration method34 after dilution with a specific solvent, and the final concentration was determined using a UV-vis spectrometer (Eppendorf, Chennai).
3. Preparation of nano-emulsions
3.1. Formulation and optimization of stable emulsions
The formulation of oil-in-water nano-emulsions was performed in two steps. The first step entailed creating a pseudo ternary phase diagram using the water titration method in different combinations of selected essential oils, such as cinnamon oil, clove oil, and eugenol, as well as surfactants, such as Tween 20 and Tween 80. To produce the best nano-emulsion composition, the surfactant was added in various quantities and combined with a predetermined amount of oil to form a total of 5 ml, and the stirring was continued at a speed of 300 rpm until the mixture became homogeneous. These formulations demonstrated no phase separation during the 12-hour incubation period, indicating the development of a stable nano-emulsion. To finalize the formulation of nano-emulsions created from these essential oils, a highly complex computational method known as the response surface methodology (RSM) was used to refer to the multiple combinations of oil and surfactant percentages of the most stable emulsions from the pseudo ternary phase diagrams. A three-factor matrix named central composite design (CCD) was the design technique preferred to optimize the formulations. All the studies were carried out by considering the mean droplet size (nm) and polydispersity index (PI) of the nano-emulsions as the dependent factors whereas the concentration of the inner oil phase (%), the concentration of surfactant (%), and ultra-sonication time (minutes) were the independent factors. The best fit model for each dependent factor was statistically analyzed.35,36
3.2. Stability studies of nano-emulsions
The kinetic stability of the prepared nano-emulsions was studied by both centrifugation and temperature studies. At first, these nano-emulsions were subjected to three cycles of temperature studies including heating at 45 °C and cooling at 4 °C and then at room temperature for a continuous period of 2 days each. After the temperature studies these emulsions were centrifuged at 1500 × g for 30 min to confirm their physical stability.37
3.3. Drug loaded nano-emulsions
The optimized stable nano-emulsions of each essential oil were taken for the preparation of drug loaded nano-emulsions. Primarily, 1 mg ml−1 of the hydrophobic drug rifampicin was dissolved in the oil phase and 1 mg ml−1 each of the hydrophilic drugs ethambutol, pyrazinamide and isoniazid was dissolved in the water phase. These prepared oil phase and water phase were then used to prepare the nano-emulsions as mentioned above.
4. Characterization of the optimized nano-emulsion
4.1. Determination of the mean size of droplets and poly dispersity index
The dynamic light scattering (DLS) technique was utilized for measuring the average size of droplets (nm) and the polydispersity index (PDI) of the optimized nano-emulsion. Data were analysed using an SZ-100 Horiba, a Japanese nanoparticle analyzer with double laser (10 mV) scattering light at 173° at 25 ± 1 °C. Data analysis was performed using the software developed by the manufacturer.
4.2. Determination of zeta potential (ζ potential)
The ζ potential (mV) of the nano-emulsions determines the electrophoretic mobility of the particles within the samples. The samples were collected in a clear zeta cell measured using special software in the SZ-100 Horiba nanoparticle analyzer.
4.3. Physicochemical properties
The study of other important physicochemical properties of these optimized nano-emulsions includes the determination of pH using a calibrated pH meter (Systronics, India), the determination of conductivity using a calibrated conductivity meter (Elico, India), and the determination of viscosity using a spindle viscometer (Brookfield, USA) with spindle sized SC4-18.38
4.4. Transmission electron microscopy analysis
Transmission electron microscopy (TEM) imaging was performed using a Tecnai F20 microscope from FEI at an accelerating voltage of 200 kV. One drop of the nano-emulsion was placed on a copper grid and then vacuum dried. This analysis was conducted to determine the morphology of the optimized formulation.
4.5. FT-IR analysis
Fourier transform infrared spectroscopy was used to carry out the drug excipient compatibility studies of single nano-emulsions and drug loaded nano-emulsion systems. The samples were measured and analysed using an FT/IR-6800 FTIR spectrophotometer (Jasco, India) within 600–4000 cm−1.
4.6. Drug release kinetic studies
The drug release kinetics of 1st line anti-Tb drugs in simulated body fluids were determined using a Franz diffusion apparatus purchased from PermeGear, Inc., USA. As we intend to focus on the nasal delivery of these drug loaded nanoemulsions, simulated lung fluids such as simulated Gamble's solution (SGS) and artificial lysosomal fluid (ALF) along with simulated gastric fluid (SGF) were chosen as the simulated body fluids and were prepared according to the US Pharmacopeia.39 The Franz diffusion chamber consisted of a 5 ml recipient compartment to which the simulated body fluid would be added and a donor compartment to which 500 μl of the drug loaded nanoemulsion would be added. These compartments were separated by a layer of cellulose nitrate dialysis membrane. The temperature in the recipient compartment was maintained at 37 °C to mimic the normal body temperature using a temperature-controlled water circulator purchased from Lab Companion (RW0525G, JeioTech., Ltd., South Korea) and stirred at 150 rpm. Samples were withdrawn from the recipient arm every 15 minutes for the first hour and then at an interval of an hour for 10 hours. The total volume of the recipient arm was maintained by adding fresh body fluid. The concentration of the drugs was estimated using a UV-vis spectrometer by measuring the respective absorbances at 252 nm for rifampicin, 260 nm for isoniazid, and 266 nm for pyrazinamide. Further statistical studies and mathematical modeling were carried out using Microsoft Excel (2019 version, Microsoft Corporation, USA).
4.7. Anti-mycobacterial studies
REMA was preferred for the confirmation of the antimycobacterial activity of the optimized nano-emulsions and both unloaded and drug loaded were studied against hospital strains of Mycobacterial tuberculosis provided by the National TB Centre, Chennai. Two-fold dilutions of the nano-emulsion system and drug loaded system (1 mg ml−1) were freshly prepared and added to a 96 well microtiter plate. The final volume of each well was brought to 100 μl with Middlebrook 7H9 broth supplemented with ODAC supplements. The bacteria were then inoculated into these plates and incubated at 37 °C for 7 days.40 30μl of 0.01% freshly prepared resazurin dye was added to each well containing the sample and mycobacterial culture and kept for overnight incubation. The lowest concentration which prevented a colour change from blue to pink was selected as the MIC value for the sample.
4.8. Statistical analysis
All the results were evaluated in triplicate to erase any chance of error. The calculations and construction of graphs were carried out using Microsoft Excel (2019 version, Microsoft Corporation, USA).
5. Results and discussion
5.1. Preparation of stable nano-emulsions
Essential oils specifically eugenol, clove oil and cinnamon oil were chosen over edible oils or vegetable oils due to their well-known antimicrobial activity41,42 and also their specific ability to solubilize a lipophilic drug like rifampicin. Selecting an appropriate surfactant is very important in the process of making the emulsion as it is an inevitable factor that validates the stability of the resulting emulsion. Based on their hydrophilic–lipophilic balance (HLB) values different surfactants can be considered when preparing both micro- and nano-emulsions. Moreover, for an ideal oil-in-water nano-emulsion, surfactants with an HLB value ranging from 12 to 16 are suitable. Therefore, TW20 and TW80 with HLB values of 16.7 and 15, respectively, were finalized for the preparation of clove oil, cinnamon oil, and eugenol nano-emulsion systems. On plotting the pseudo ternary phase diagram, the region which showed the clearest formulations was the lowest percentage of surfactant with the best oil percentage from a wide range. Keeping the above diagram as a guide, the ranges for the oil content (2–6%), surfactant concentration (10–30%), and ultra-sonication time (1–10 minutes) were selected for executing the central composite design. After the completion of the emulsion formulation, the optimization was done several times by considering both dependent and independent variables for each separate oil (Table 1). The dependent variables such as the mean size of the droplets (nm) and PDI were experimentally determined and added to the software to optimize the formulation computationally. The ANOVA test performed within the RSM notably demonstrates values with lower p values and hence fit properly into the quadratic model as shown in the summarized experimental data in Table 2. In this case, A, B, AB, AC, BC, A2, B2, and C2 are significant model terms and were calculated using eqn (1)–(3) for each oil where R1 and R2 correspond to the mean droplet size in nm and the polydispersity index of the emulsions, respectively. The fit statistics tabulated in Table 2 provided for each oil based on the variables by the software also clearly suggest the desirability of the experimental design. The higher coefficient of variance (CV%) in the fit statistics establishes the acceptability of the experimental model, whilst a suitable precision greater than 4 denotes an acceptable signal-to-noise ratio rendering the design experimentally feasible. From Fig. 1, it can be observed that with an increase in the concentration of oil and surfactant there is a decrease in mean droplet size. Finally, we have narrowed down to one final concentration of optimized value for all the three essential oil-based nano-emulsions: 4.5% of oil concentration, 22% of surfactant concentration and an ultra-sonication time of 8 minutes at an intensity of 40% with a pulse of 30 seconds every 2 minutes by maintaining a temperature of about 4 °C during the preparation of nano-emulsions. When these emulsion systems were exposed to stability tests, it was discovered that there was no notable change in the first three months, but by the sixth month, the droplets had shrunk in size, indicating that the nano-emulsions' stability had been compromised.
Table 1 RSM chart of different factors and responses for each oil-based nano emulsion
Run |
Factor A oil (%) |
Factor B surfactant (%) |
Factor C Ultrasonication (minutes) |
Clove oil |
Eugenol |
Cinnamon oil |
Response 1 droplet size (nm) |
Response 2 PDI |
Response 1 droplet size (nm) |
Response 2 PDI |
Response 1 droplet size (nm) |
Response 2 PDI |
1 |
−1.000 |
1.000 |
−1.000 |
0 |
0 |
14.7 |
0.239 |
7.2 |
0.255 |
2 |
−1.682 |
0.000 |
0.000 |
7.7 |
0.322 |
12.8 |
0.171 |
8.4 |
0.158 |
3 |
0.000 |
0.000 |
−1.682 |
11.4 |
0.171 |
22.4 |
0.316 |
10.1 |
0.188 |
4 |
0.000 |
0.000 |
0.000 |
11.5 |
0.193 |
20.9 |
0.33 |
10.3 |
0.186 |
5 |
0.000 |
0.000 |
0.000 |
11.3 |
0.179 |
20.8 |
0.362 |
10.6 |
0.145 |
6 |
−1.000 |
−1.000 |
1.000 |
10.3 |
0.217 |
0 |
0 |
10 |
0.144 |
7 |
0.000 |
0.000 |
1.682 |
11.3 |
0.253 |
23 |
0.346 |
10.7 |
0.208 |
8 |
0.000 |
0.000 |
0.000 |
11.3 |
0.15 |
21 |
0.3 |
10.6 |
0.145 |
9 |
−1.000 |
−1.000 |
−1.000 |
10.4 |
0.224 |
0 |
0 |
9.5 |
0.158 |
10 |
0.000 |
0.000 |
0.000 |
11.3 |
0.15 |
20.9 |
0.308 |
10.6 |
0.181 |
11 |
0.000 |
1.682 |
0.000 |
9.2 |
0.159 |
14.1 |
0.263 |
8.3 |
0.184 |
12 |
0.000 |
0.000 |
0.000 |
11.4 |
0.202 |
21.3 |
0.272 |
10.6 |
0.164 |
13 |
1.000 |
1.000 |
−1.000 |
12 |
0.206 |
21.8 |
0.353 |
0 |
0 |
14 |
−1.000 |
1.000 |
1.000 |
0 |
0 |
14.9 |
0.261 |
8.3 |
0.152 |
15 |
1.000 |
1.000 |
1.000 |
12.1 |
0.2 |
22.2 |
0.344 |
11.1 |
0.191 |
16 |
0.000 |
0.000 |
0.000 |
11.2 |
0.194 |
21 |
0.293 |
10.6 |
0.205 |
Table 2 ANOVA and fit statistics of each nanoemulsion
Source |
Sum of squares |
df |
Mean square |
F value |
P value |
Fit statistics |
Remarks |
R
1
|
R
2
|
Clove |
471.90 |
9 |
52.43 |
11.98 |
0.0003 |
C.V.%: 27.46 |
C.V.%: 54.91 |
Significant |
Adequate precision: 9.4722 |
Adequate precision: 6.8608 |
Eugenol |
350.02 |
9 |
38.89 |
13.10 |
0.0002 |
C.V.%: 33.27 |
C.V.%: 31.56 |
Significant |
Adequate precision: 8.7053 |
Adequate precision: 8.8194 |
Cinnamon |
358.01 |
9 |
38.9 |
9.09 |
0.0009 |
C.V.%: 23.44 |
C.V.%: 28.28 |
Significant |
Adequate precision: 10.71 |
Adequate precision: 9.5138 |
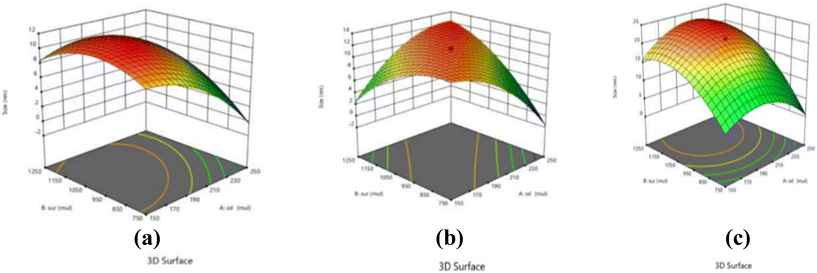 |
| Fig. 1 3-D response plots showing the interaction effect for particle size, it being dependent on oil (%), surfactant (%), ultrasonication time (minutes), of the (a) cinnamon oil nano emulsions, (b) clove oil nano emulsions and (c) eugenol nano emulsions. | |
Eqn (1): cinnamon
| R1 = +10.57 + 2.78A + 1.54B + 1.00C + 1.89AB + 1.19AC + 1.46BC + 2.30A2 + 2.32B2 + 0.1065C2 | (1) |
R2 = +0.1739 + 0.0574A + 0.0443B + 0.0079C + 0.0107AB + 0.0385AC + 0.0128BC + 0.0350A2 + 0.0304 + B2 + 0.0070C2 |
Eqn (2): clove
| R1 = +11.36 + 0.6993A + 1.38B + 0.0123C + 5.60AB + 0.0250AC + 0.0250BC + 2.80A2 + 2.53B2 + 0.1445C2 | (2) |
R2 = +0.141 + 0.0422A + 0.0170B + 0.0091C + 0.1059AB + 0.0001AC + 0.0001BC |
Eqn (3): eugenol
| R1 = 21.0579 + 0.521859 × A + 7.1256 × B + 0.117822 × C + 1.8 × AB + 0.025 × AC + 0.075 × BC + 5.64305 × A2 + 5.41324 × B2 + 0.119868 × C2 | (3) |
R2 = 0.311649 + 0.00663305 × A + 0.120036 × B + 0.0046463 × C + 0.024625 × AB + 0.003875 × AC′′ + 0.001625 × BC + 0.0849993 × A2 + 0.0687359 × B2 + 0.00179804 × C2 |
5.2. Characterization of the optimized nano-emulsions
The formation of nano-emulsions was tested with DLS to calculate the average size of emulsion particles where the oil droplets were covered with a water environment. The mean droplet sizes of the nano-emulsions formed for cinnamon oil, clove oil, and eugenol were within the range of 10 nm to 20 nm whereas the drug-loaded nano-emulsions were found to have double the size of the free nano-emulsions which is because of incorporation of multiple drugs into the nano emulsion systems (Fig. 2) with a spherical morphology (Fig. 3). The polydispersity index (PDI) of all the formed nano-emulsions was found to have values lesser than 0.5 which indicates the homogeneity of the particles within the emulsion, that is, a lesser PDI value indicates a high rate of homogeneity and vice versa.43 The status of stability and the chances of accumulation are determined by measuring the surface charge of the nano-emulsion. Strong surface charges cause mutual repulsion and provide good stability, allowing the emulsion particles to maintain their size and shape. The charges were measured using the ζ potential and it was observed that the ζ potential values of all the emulsions were low, negative and within the range indicating the presence of a non-ionic emulsifier used for the preparation that wraps around the particle where it can give some interactive forces between the oil and water and eliminate chances of agglomeration by serving as a barrier. pH, conductivity, and viscosity studies are a few other factors that describe the stability of the emulsion in detail, as shown in Table 3. It can be correlated that the surface charge of most of the oil-in-water combinations was negative due to the presence of more negative functional groups like –OH, –C
O, and –COOH groups, so that the pH becomes acidic due to the abundance of acidic moieties. The involvement of drug moieties reduces the negative surface charge because of the interaction of drug molecules with oil or water. This further confirms the complete dissolution of drugs into the system. The presence of charges makes the electron flow transparent and facilitates electron conductivity so that all the combinations exhibit conductivity as it involves charges, and conductivity becomes less during the incorporation of drug molecule as they resist the flow of electrons. The FT-IR spectra of bulk oil, nano-emulsions, and drug-loaded nano-emulsions were analysed to find if any chemical change happened in the bulk oil or if any specific interaction occurred in the bulk oil in the formulation of the nano-emulsion and when loaded with drugs. From the spectra in Fig. 4, it can be observed that there is no evident change in the spectra peaks apart from the peak at 3369 cm−1 which showed improved H–OH interactions in the case of nano-emulsions, both drug loaded and non-drug loaded. This can be explained by the presence of high-water content in the formulation of nano-emulsions. Thus, we can conclude that the oil phase is as chemically stable as it is in the bulk oil even after the formulation of nano-emulsions.
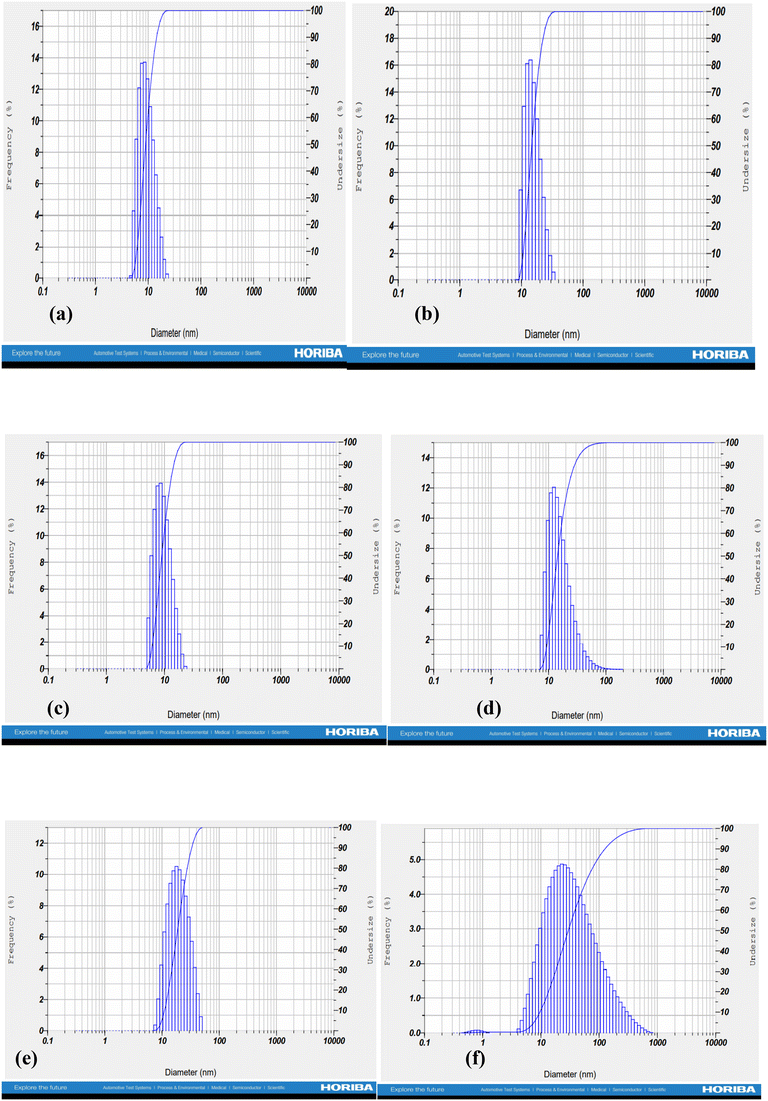 |
| Fig. 2 Droplet size and poly dispersity index of the optimized (a) and (b) clove oil nano emulsions, (c) and (d) cinnamon nano emulsions, and € and (f) eugenol nano emulsions. | |
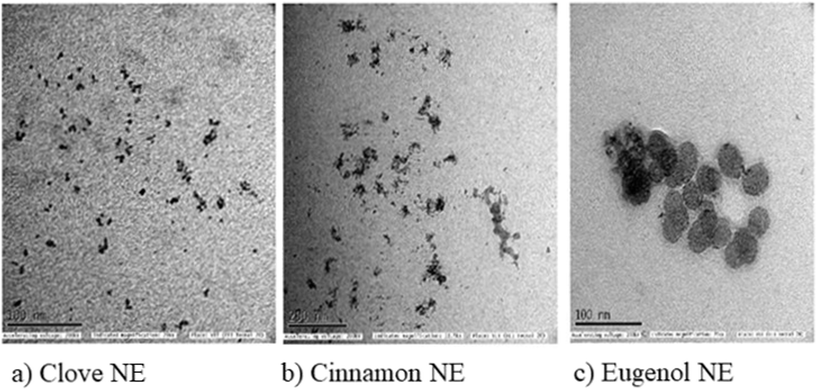 |
| Fig. 3 TEM analysis: (a) clove nanoemulsions; (b) cinnamon nanoemulsions; (c) eugenol nanoemulsions. | |
Table 3 Physicochemical properties of the optimized nano emulsions
Sample |
Mean droplet size (nm) |
pH |
Zeta potential (mV) |
Conductivity (μS cm−1) |
Viscosity (cP) |
CLNE |
10.8 ± 1.9 |
3.86 ± 2 |
−13.0 ± 0.52 |
0.145 |
20.38 |
CNNE |
10.8 ± 0.2 |
4.36 ± 0.5 |
−12.2 ± 0.34 |
0.114 |
28.29 |
ENE |
19.3 ± 2.5 |
4.83 ± 0.2 |
−17.1 ± 0.10 |
0.134 |
291.4 |
CLDLNE |
21.4 ± 2.1 |
4.31 ± 2 |
−5.3 ± 0.23 |
0.103 |
28.88 |
CNDLNE |
21.1 ± 1.6 |
4.15 ± 1.1 |
−3.6 ± 0.61 |
0.104 |
26.1 |
EDLE |
37.7 ± 0.4 |
4.9 ± 0.3 |
−10.3 ± 0.31 |
0.168 |
255.9 |
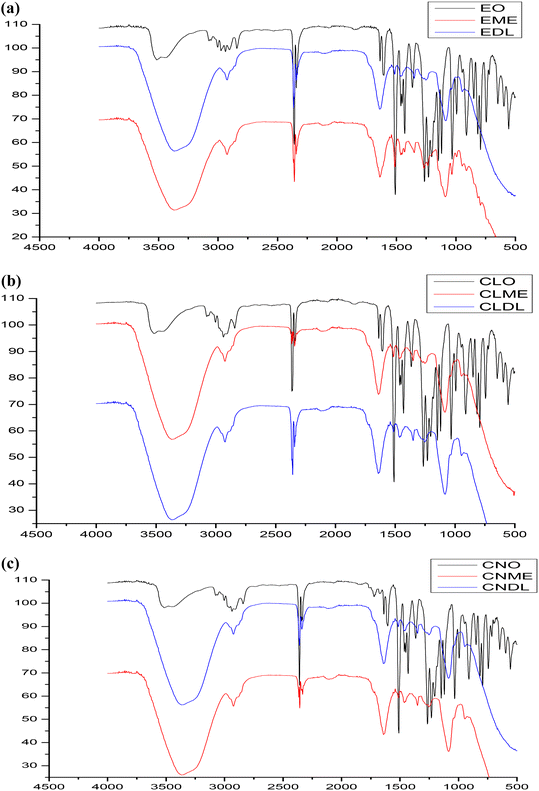 |
| Fig. 4 FTIR spectra: (a) cinnamon oil-based systems; (b) clove oil-based systems; (c) eugenol-based systems. | |
5.3. Drug release kinetic studies
From statistical analysis in general, we can comprehend (Fig. 5) that all the three eugenol based nanoemulsion systems successfully aided in controlled and sustainable release of 1st line anti-tubercular drugs over a period of 10 hours into the simulated body fluids. From a mathematical point of view, the release of each drug in different simulated body fluids followed mostly the Hixson–Crowell model (HO) which depends on the size of particles with respect to the cube root of the volume, the first order model (FO) which depends on the rate of release with respect to the drug concentration, and the zero order model (ZO) which depends on the drug release with respect to time, which are tabulated in Tables S1–S3.†
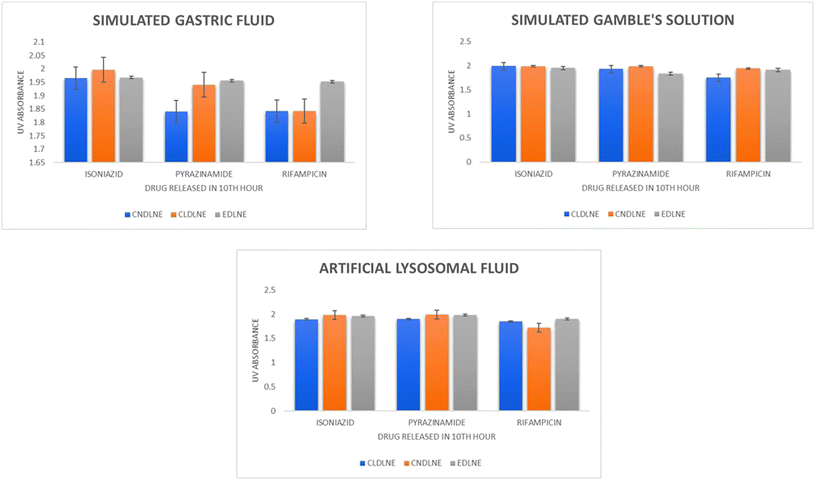 |
| Fig. 5 Release kinetics profile of 1st line anti-tubercular drugs in simulated gastric fluid, simulated Gamble's solution, and artificial lysosomal fluid. | |
5.4. Antimycobacterial studies
The antimycobacterial activities of the essential oil based nano-emulsion and drug loaded nano-emulsion systems in this study were observed to have better results when compared to the drug alone, as shown in Table 4. It is observed that all the three essential oil based multiple drug loaded nanoemulsions completely inhibited the growth of the bacterium in 1/1024 dilution, whereas the cinnamon and eugenol unloaded nanoemulsions showed their maximum activities only at 1/512 dilution.
Table 4 Minimum inhibitory concentration (MIC) values for each oil based microemulsion and drug loaded microemulsion and each separate drug
Compound name |
1/2 |
1/4 |
1/8 |
1/16 |
1/32 |
1/64 |
1/128 |
1/256 |
1/512 |
1/1024 |
MIC |
Concentration in mg ml−1 |
0.5 |
0.25 |
0.12 |
0.06 |
0.03 |
0.015 |
0.0075 |
0.00375 |
0.0018 |
0.0009 |
|
Cinnamon oil single Nano emulsion |
N/N |
N/N |
N/N |
N/N |
N/N |
N/N |
N/N |
N/N |
N/N |
P/P |
1/1024 dilution or 0.0009 mg ml−1 |
Cinnamon oil drug loaded NE |
N/N |
N/N |
N/N |
N/N |
N/N |
N/N |
N/N |
N/N |
N/N |
N/N |
<1/1024 dilution or < 0.0009 mg ml−1 |
Cinnamon oil NE + RIF |
N/N |
N/N |
N/N |
N/N |
N/N |
N/N |
N/N |
N/N |
N/N |
N/N |
<1/1024 dilution or <0.0009 mg ml−1 |
Cinnamon oil NE + INH |
N/N |
N/N |
N/N |
N/N |
N/N |
N/N |
N/N |
N/N |
N/N |
N/N |
<1/1024 dilution or <0.0009 mg ml−1 |
Cinnamon oil NE + EMB |
N/N |
N/N |
N/N |
N/N |
N/N |
N/N |
N/N |
N/N |
N/N |
N/N |
<1/1024 dilution or <0.0009 mg ml−1 |
Cinnamon oil NE + PYZ |
N/N |
N/N |
N/N |
N/N |
N/N |
N/N |
N/N |
N/N |
N/N |
N/N |
<1/1024 dilution or <0.0009 mg ml−1 |
Clove oil single Nano emulsion |
N/N |
N/N |
N/N |
N/N |
N/N |
N/N |
N/N |
N/N |
N/N |
N/N |
<1/1024 dilution or<0.0009 mg ml−1 |
Clove oil drug loaded NE |
N/N |
N/N |
N/N |
N/N |
N/N |
N/N |
N/N |
N/N |
N/N |
N/N |
<1/1024 dilution or <0.0009 mg ml−1 |
Clove oil NE + RIF |
N/N |
N/N |
N/N |
N/N |
N/N |
N/N |
N/N |
N/N |
N/N |
N/N |
<1/1024 dilution or <0.0009 mg ml−1 |
Clove oil NE + INH |
N/N |
N/N |
N/N |
N/N |
N/N |
N/N |
N/N |
N/N |
N/N |
N/N |
<1/1024 dilution or <0.0009 mg ml−1 |
Clove oil NE + EMB |
N/N |
N/N |
N/N |
N/N |
N/N |
N/N |
N/N |
N/N |
N/N |
N/N |
<1/1024 dilution or <0.0009 mg ml−1 |
Clove oil NE + PYZ |
N/N |
N/N |
N/N |
N/N |
N/N |
N/N |
N/N |
N/N |
N/N |
N/N |
<1/1024 dilution or <0.0009 mg ml−1 |
Eugenol oil single Nano emulsion |
N/N |
N/N |
N/N |
N/N |
N/N |
N/N |
N/N |
N/N |
N/N |
P/P |
1/1024 dilution or 0.0009 mg ml−1 |
Eugenol oil drug loaded NE |
N/N |
N/N |
N/N |
N/N |
N/N |
N/N |
N/N |
N/N |
N/N |
N/N |
<1/1024 dilution or <0.0009 mg ml−1 |
Eugenol oil NE + RIF |
N/N |
N/N |
N/N |
N/N |
N/N |
N/N |
N/N |
N/N |
N/N |
N/N |
<1/1024 dilution or <0.0009 mg ml−1 |
Eugenol oil NE + INH |
N/N |
N/N |
N/N |
N/N |
N/N |
N/N |
N/N |
N/N |
N/N |
P/P |
1/1024 dilution or 0.0009 mg ml−1 |
Eugenol oil NE + EMB |
N/N |
N/N |
N/N |
N/N |
N/N |
N/N |
N/N |
N/N |
N/N |
P/P |
1/1024 dilution or 0.0009 mg ml−1 |
Eugenol oil NE + PYZ |
N/N |
N/N |
N/N |
N/N |
N/N |
N/N |
N/N |
N/N |
N/N |
P/P |
1/1024 dilution or <0.0009 mg ml−1 |
6. Conclusion
The oil-in-water nano emulsion system we have developed has been proved to be substantially more stable through several characterization studies. Combinational delivery of both hydrophobic and hydrophilic 1st line anti-TB drugs in a single nanoemulsion system with a diameter of less than 40 nm provides an exceptional chance of site-specific release of drugs. From the above studies, we can conclude that combining 1st line tuberculosis drugs with eugenol based essential oils in the form of nano-emulsions has enhanced their drug potency as an anti-mycobacterium agent. From the MIC values, it is highly proved that the eugenol based multiple drug loaded nano-emulsions showed more promising results than the free nanoemulsions and the single drug loaded nanoemulsions. From the drug release kinetic studies, we showed beyond doubt that these formulations aided in the extended release of the 1st line anti-tubercular drugs till 10 hours and we can also say that by choosing essential oils we can rule out the toxicity effects of these systems. Further studies using in vitro and in vivo models can assure the efficiency and desirability of this treatment method on MDR-TB infected patients.
Conflicts of interest
None of the authors have had any chance for conflict of interest during this research study.
Acknowledgements
Funding: this research did not receive any specific funding from funding agencies in public, commercial, or not-for-profit sectors. We would like to thank the Vellore Institute of Technology, Vellore campus for providing all the facilities and instruments required to carry out the research work.
References
-
W. H. Organization, Global tuberculosis report 2021, Licence: CC BY-NC-SA 3.0 IGO, Cataloguing-in-Publication, World Health Organization, Geneva, 2021 Search PubMed.
- K. Sakamoto, Vet. Pathol., 2012, 49, 423–439 CrossRef CAS PubMed.
- Q. Chai, Y. Zhang and C. H. Liu, Front. Cell. Infect. Microbiol., 2018, 8, 1–15 CrossRef PubMed.
- Y. L. Janin, Bioorg. Med. Chem., 2007, 15, 2479–2513 CrossRef CAS PubMed.
- S. Chakraborty and K. Y. Rhee, Cold Spring Harbor Perspect. Med., 2015, 5, 1–11 CAS.
- K. J. Seung, S. Keshavjee and M. L. Rich, Cold Spring Harbor Perspect. Med., 2015, 5(9), a017863 CrossRef PubMed.
- J. Cao, Y. Mi, C. Shi, Y. Bian, C. Huang, Z. Ye, L. Liu and L. Miao, Biochem. Biophys. Res. Commun., 2018, 497, 485–491 CrossRef CAS PubMed.
- A. Prasanna and V. Niranjan, Bioinformation, 2019, 15, 261–268 CrossRef PubMed.
- N. Padayatchi, A. Daftary, N. Naidu, K. Naidoo and M. Pai, BMJ Glob. Health, 2019, 4, 1–6 Search PubMed.
- P. Brouqui, F. Quenard and M. Drancourt, Int. J. Antimicrob. Agents, 2017, 49, 554–557 CrossRef CAS PubMed.
- M. A. Espinal and C. Dye, Lancet, 2005, 365(9466), 1206–1209 CrossRef PubMed.
- G. Tiwari, R. Tiwari, S. Bannerjee, L. Bhati, S. Pandey, P. Pandey and B. Sriwastawa, Int. J. Pharm. Invest., 2012, 2, 2 CrossRef PubMed.
- R. J. Wilson, Y. Li, G. Yang and C. X. Zhao, Particuology, 2022, 64, 85–97 CrossRef CAS.
- J. Jerobin, R. S. Sureshkumar, C. H. Anjali, A. Mukherjee and N. Chandrasekaran, Carbohydr. Polym., 2012, 90(4), 1750–1756 CrossRef CAS PubMed.
- J. S. Franklyne, P. M. Gopinath, A. Mukherjee and N. Chandrasekaran, Curr. Opin. Colloid Interface Sci., 2021, 54, 101458 CrossRef CAS PubMed.
- Y. Ma, D. Liu, D. Wang, Y. Wang, Q. Fu, J. K. Fallon, X. Yang, Z. He and F. Liu, Mol. Pharmaceutics, 2014, 11, 2623–2630 CrossRef CAS PubMed.
- E. Sieniawska, R. Sawicki, M. Swatko-Ossor, A. Napiorkowska, A. Przekora, G. Ginalska and E. Augustynowicz-Kopec, Molecules, 2018, 23, 176 CrossRef PubMed.
- M. Kumar, R. S. Bishnoi, A. K. Shukla and C. P. Jain, Prev. Nutr. Food Sci., 2019, 24, 225–234 CrossRef CAS PubMed.
- G. Niu and W. Li, Trends Biochem. Sci., 2019, 44, 961–972 CrossRef CAS PubMed.
- G. Martelli and D. Giacomini, Eur. J. Med. Chem., 2018, 158, 91–105 CrossRef CAS PubMed.
- A. Man, L. Santacroce, R. Jacob, A. Mare and L. Man, Pathogens, 2019, 8, 1–11 CrossRef PubMed.
- K. Wí Nska, W. M. Aczka, J. Łyczko, M. Grabarczyk, A. Czubaszek and A. Szumny, Molecules, 2019, 24(11), 2130 CrossRef PubMed.
- V. P. Baldin, R. B. de Lima Scodro, M. A. Lopes-Ortiz, A. L. de Almeida, Z. C. Gazim, L. Ferarrese and V. dos Santos Faiões, Phytomedicine, 2018, 47, 34–39 CrossRef CAS PubMed.
- V. Jayapal, C. K. Vidya Raj, M. Muthaiah, V. K. Chadha, U. Brammacharry, S. Selvaraj and J. M. Easow, Indian J. Tuberc., 2021, 68(4), 470–473 CrossRef PubMed.
- N. E. Sandoval-Montemayor, A. García, E. Elizondo-Treviño, E. Garza-González, L. Alvarez, M. Del and R. Camacho-Corona, Molecules, 2012, 17(9), 11173–11184 CrossRef CAS PubMed.
- N. R. Labiris and M. B. Dolovich, Br. J. Clin. Pharmacol., 2003, 56, 588 CrossRef CAS PubMed.
- R. K. Harwansh, R. Deshmukh and M. A. Rahman, J. Drug Delivery Sci. Technol., 2019, 51, 224–233 CrossRef CAS.
- S. Gelperina, K. Kisich, M. D. Iseman and L. Heifets, Am. J. Respir. Crit. Care Med., 2005, 172, 1487–1490 CrossRef PubMed.
- P. Muttil, C. Wang and A. J. Hickey, Pharm. Res., 2009, 26, 2401–2416 CrossRef CAS PubMed.
- R. Pandey and G. Khuller, Curr. Drug Delivery, 2005, 1, 195–201 CrossRef PubMed.
- R. Ahmed, M. Aucamp, N. Ebrahim and H. Samsodien, J. Drug Delivery Sci. Technol., 2021, 66, 102773 CrossRef CAS.
- G. Chen, Y. Wu, D. Yu, R. Li, W. Luo, G. Ma and C. Zhang, J. Biomater. Appl., 2019, 33, 989–996 CrossRef CAS PubMed.
- H. H. Tayeb and F. Sainsbury, Nanomedicine, 2018, 13, 2507–2525 CrossRef CAS PubMed.
- A. Ebenazer, J. S. Franklyne, A. Mukherjee and N. Chandrasekaran, Int. J. Appl. Pharm., 2018, 10, 72–81 CrossRef CAS.
- P. Mishra, M. K. Samuel, R. Reddy, B. K. Tyagi, A. Mukherjee and N. Chandrasekaran, Environ. Sci. Pollut. Res., 2018, 25, 2211–2230 CrossRef CAS PubMed.
- P. Pongsumpun, S. Iwamoto and U. Siripatrawan, Ultrason. Sonochem., 2020, 60, 104604 CrossRef CAS PubMed.
- J. S. Franklyne, L. Andrew Ebenazer, A. Mukherjee and C. Natarajan, Appl. Nanosci., 2019, 9, 1405–1415 CrossRef CAS.
- W. S. Samiun, S. E. Ashari, N. Salim and S. Ahmad, Int. J. Nanomed., 2020, 15, 1585–1594 CrossRef CAS PubMed.
- M. R. C. Marques, R. Loebenberg and M. Almukainzi, Dissolution Technol., 2011, 18, 15–28 CrossRef CAS.
- J. C. Palomino, A. Martin, M. Camacho, H. Guerra, J. Swings and F. Portaels, Antimicrob. Agents Chemother., 2002, 46, 2720–2722 CrossRef CAS PubMed.
- A. L. de Almeida, K. R. Caleffi-Ferracioli, R. B. de Scodro, V. P. Baldin, D. C. Montaholi, L. F. Spricigo, S. S. Nakamura-Vasconcelos, L. A. Hegeto, E. G. Sampiron, G. F. Costacurta, D. A. dos Yamazaki, G. de F. Gauze, V. L. Siqueira and R. F. Cardoso, Future Microbiol., 2019, 14, 331–344 CrossRef CAS PubMed.
- A. O. Sergio, C. V. Fabiola, N. M. Guadalupe, R. C. Blanca and H. O. León, Adv. Biol. Chem., 2013, 3, 480–484 CrossRef CAS.
- T. Tadros, P. Izquierdo, J. Esquena and C. Solans, Adv. Colloid Interface Sci., 2004, 108–109, 303–318 CrossRef CAS PubMed.
|
This journal is © The Royal Society of Chemistry 2023 |