DOI:
10.1039/D3MA00615H
(Paper)
Mater. Adv., 2023,
4, 5160-5174
Fabrication of highly efficient encapsulated SnO2@alginate beads as regenerative nanosorbents for anionic dye pollutants removal from aqueous solution†
Received
29th August 2023
, Accepted 12th September 2023
First published on 13th September 2023
Abstract
Nano-adsorbents are emerging as potential candidates for the treatment of toxic organic pollutants. In this context, spherical SnO2 nanoparticles (NPs) are synthesized through a facile hydrothermal method without the usage of toxic chemical precursors, which is confirmed through PXRD, TEM, FTIR, and Raman spectroscopy. SEM imaging was used to confirm the spherical morphology of the NPs. These NPs when applied as nano-adsorbents demonstrate the ability to remove anionic dyes from aqueous solution. In particular, 0.1 g/100 mL SnO2 NPs exhibit 95.96% Congo red (CR) removal efficiency from its aqueous solution within an exposure time of 1 min. Primary driving forces of adsorption can be attributed to electrostatic attraction and hydrogen bonding. Raman spectra confirmed the presence of oxygen vacancies which can be a major factor for their high adsorption efficiency. The adsorption process for CR dye fitted felicitously with pseudo-second order kinetics indicating chemisorption and the Langmuir model indicating CR monolayer formation on the nano-adsorbent. The maximum adsorption capacity is estimated to be 961.5 mg g−1. Finally, the possibility of metal leaching has been addressed through encapsulation of SnO2 NPs within sodium alginate crosslinked by CaCl2, which also exhibited nearly 75% adsorption efficiency after an exposure time of 5 min, with successful regeneration. In addition to adsorption capability toward CR dye, SnO2@SA also exhibited moderate adsorption efficiencies towards eosin yellow, orange G, and methyl orange dyes. This study demonstrates SnO2 NPs as a potential nano-adsorbent for treatment of toxic effluents through a sustainable, facile and economical method.
1. Introduction
On an industrial basis, each year, approximately 7 × 105 tons of wide ranges of synthetic dyes and pigments are used.1 The printing and textile industry releases industrial wastewater after primary treatment, which does not efficiently remove the dyes, thereby resulting in the contamination of water bodies.2,3 The release of untreated effluents containing a high concentration of dyes into the aquatic environment endangers both the ecological environment and human health. Due to their toxicity, mutagenicity, and carcinogenicity, azo dyes like Direct Blue15, Congo Red, and Direct Red 2 are recognized as hazardous to human health.4,5 Particularly, Congo red (CR) dye, which is used extensively in the textile sector, has a strong affinity for cellulose fibers.6 Numerous methods have been investigated to reduce dye pollution in wastewater, each with various benefits and drawbacks. Some of these methods include biodegradation,7 photodegradation,8 membrane separation,9 and adsorption.2,10 Adsorption is acknowledged as a viable method due to its simplicity, affordability, and sustainability. Micro-pollutants (such as dyes) can be removed from industrial effluents using the technique of adsorption. Adsorbents used in this technique should be easy to use and store, have good mechanical strength, possess the right pore structure and surface chemical composition, have significant capacity for adsorbing the adsorbate, and be highly recyclable and re-usable for economic reasons.10
Various adsorbents related to the environmental remediation of dyes in textile effluents have been explored in a wide range of publications.2,10–14 However, SnO2 nanoparticles (NPs) have attracted significant interest in multiple industries because of their intriguing physicochemical features.15 SnO2 is an n-type semiconducting oxide having a wide band gap and it possesses high resistance to photocorrosion.16 SnO2 NPs can be prepared through various routes such as sol–gel,17 hydrothermal,18 microemulsion,19 and the solvothermal method.20 Among the available methods, the hydrothermal technique has emerged as a highly versatile and conventional choice, garnering popularity in industrial applications owing to its straightforward methodology, high yield, cost-effectiveness and suitability for moderate-temperature processes.21 Moreover, this method is well-recognized for its ability to produce NPs with desired size and shape, well-crystalized products, and NPs exhibiting high crystallinity.22 SnO2 has been investigated as a potential material with numerous unique surface features, such as luminescence and photocatalytic activity, over the past ten years.15,23 Due to its high chemical stability and unique physico-chemical properties, it has been utilized in various applications such as sensors,24 photocatalytic degradation,15 thermoluminescence25 and many others.
Table 1 presents the various studies carried out for removal and degradation of CR dye using SnO2 based nanomaterials via two common routes, namely adsorption and photocatalytic degradation. Adsorbents are typically utilized as a fine powder, and the difficulty of separating the adsorbent from the effluent may result in sorbent loss. Besides this, metal leaching can also pose as an inevitable subsequence of using the powder form of adsorbents. Encapsulating nanomaterials in alginate beads is a clever solution to this challenge. Alginate is a polymer derived from brown seaweed. It has substantial advantages, including accessibility, relatively inexpensiveness, non-toxicity, biocompatibility, and biodegradability.32 Nanomaterial-alginate beads have garnered attention due to the combined properties and advantages of their constituents.6,33–38
Table 1 Investigations related to SnO2 based nanomaterials for mitigation of CR dye via two facile methods (adsorption and photocatalytic degradation)
Nanomaterial |
Synthesis |
Removal technique |
Removal efficiency (%) |
Contact time (min) |
Ref. |
SnO2 |
Sol–gel method |
Adsorption |
84.41 |
60 |
17
|
Fe2O3–SnO2 nanorods |
Wet-chemical method |
Adsorption |
99.9 |
30 |
26
|
SnO2 |
Simarouba glauca leaf extract |
Photocatalytic degradation |
80 |
150 |
27
|
ZnO/SnO2 |
Electrospun method |
Photocatalytic degradation |
78.29 |
60 |
28
|
SnO2 |
Precipitation and calcination |
Photocatalytic degradation |
100 |
97 |
29
|
SnO2/chitosan |
Chemical precipitation |
Photocatalytic degradation |
95 |
60 |
30
|
SnO2 nanosheets |
Hydrothermal method |
Photocatalytic degradation |
96 |
55 |
31
|
In this study, the efficiency of hydrothermally produced SnO2 NPs for CR dye removal from its aqueous solution is meticulously evaluated. Additionally, the encapsulation of SnO2 NPs in sodium alginate (SA) beads, their use as nano-adsorbents, as well as their capacity to demonstrate regenerative cycles have been explored. Three primary approaches were used to conduct this study: sustainability, viability, and cost-effectiveness. To completely assess their adsorption effectiveness, a range of operational parameters, including pH, contact time, the amount of adsorbent, and dye concentrations, have been studied. To ascertain the adsorption mechanisms, the adsorption isotherms and kinetics were also investigated. SnO2 encapsulated within SA (SnO2@SA) beads highlights the reduced likelihood of post-adsorption metal leaching while also illuminating the practical applicability of the synthesized NPs. In its entirety, the utilization of SnO2 NPs for dye removal and their encapsulation within alginate beads constitute a fascinating approach that provides numerous advantages over traditional techniques, including the ease of adsorbent separation from the treated wastewater, the adsorbent's increased stability and reusability, and the nanoparticles’ decreased toxicity. This research is intended to help establish an effective and sustainable process for treating dye wastewater, which could have major positive effects on the environment, water industry and economy.
2. Materials and method
2.1 Materials
The materials SnCl2·2H2O (tin(II) chloride dihydrate, 98–103% purity), CaCl2 (calcium dichloride, 98% purity), CH3OH (methanol having >99% purity), ethanol and acetone were purchased from Merck Chemicals. H2O2 (hydrogen peroxide, 30% w/v purity), Congo red, eosin yellow, methyl orange and orange G were purchased from Thermo Fisher Scientific. Sodium alginate was purchased from High Purity Laboratory Chemical Pvt. Ltd. All chemicals and solvents used in the experiments were of analytical grade.
2.2 Synthesis of nanoparticles
SnO2 was prepared through a facile hydrothermal reaction. In a clean and dry vessel, 40 mL methanolic solution of 0.11 M SnCl2·2H2O was used. To this, 10 mL of hydrogen peroxide was added. The solution was autoclaved at 150 °C for 15 hours. Thereafter, the sample was washed numerous times with distilled water and then with methanol for removal of any residual impurities. Finally, the sample was oven dried at 60 °C and ground in a mortar and pestle to obtain a white homogeneous SnO2 NP powder.
2.3 Synthesis of alginate beads
A 3% solution of sodium alginate was prepared which was continuously stirred for 2 hours to form a homogeneous viscous gel. 100 mL of 0.05 M CaCl2 solution was prepared in a separate beaker. This was followed by dropwise addition of alginate gel to the CaCl2 solution which led to the formation of light brown spherical beads.39,40 The beads were kept immersed in CaCl2 overnight, and then washed with distilled water several times. Finally, the beads were partially oven dried at 80 °C to remove excess water and then utilized for adsorption studies.
2.4 Encapsulation of SnO2 in alginate beads
First, 3% solution of sodium alginate was prepared. To the viscous gel, varying concentrations of SnO2 NPs (0.1 g, 0.2 g and 0.5 g) were added with continuous stirring for 2 hours to form a homogeneous viscous gel. This was followed by preparation of 0.05 M CaCl2 solution. The viscous gel was dropwise added to CaCl2 solution to form spherical white colored SnO2@SA beads. Further, these beads were kept overnight in CaCl2 solution for hardening, which was followed by washing with distilled water. Finally, they were partially oven dried at 80 °C to remove excess water for further usage in adsorption studies.
2.5 Adsorption of anionic dyes
All the batch experiments, for adsorption studies, were carried out at room temperature. First, tests were conducted in 100 mL glass beakers with varying dye concentrations (5–60 μM) using the powder form of SnO2 NPs. Similar batch experiments were carried out for investigating the efficiency of SnO2@SA beads in 5 μM aqueous solutions of anionic dyes. 5 mL aliquots of solution were collected at regular time intervals, and then centrifuged to remove the nano-adsorbent for further analysis on a UV-Visible spectrophotometer. For pH studies, 1 M HCl/NaOH solutions were used. For carrying out desorption studies, chemical regeneration was carried out using 15 mL of various eluents such as methanol, ethanol, acetone, and dil. HCl.
2.6 Instruments
The crystalline structure of the synthesized NPs was characterized by PXRD using a scan rate of 1° min−1 for 2θ range from 5° to 70° in a Rigaku Miniflex 600 (Japan) X-ray diffractometer (λ = 1.5406 Å). For determining the sample morphology and elemental composition, SEM images and EDX spectra were obtained from a SEM (JEOL) coupled with an EDX detector. TEM images were obtained from a Tecnai G2 20 S-TWIN TEM. FTIR and Raman spectra were recorded using a Shimadzu instrument and an EnSpectr+R532 spectrometer (λ = 532 nm), respectively. Sn detection was carried out on Agilent ICP-MS 7900. UV-Visible spectra were recorded using a UV-Visible spectrophotometer (PG Instruments, India).
3. Results and discussion
3.1 X-ray diffraction
The crystallographic studies of SnO2 NPs have been confirmed by PXRD. Fig. 1(a) depicts the obtained XRD pattern. NPs are confirmed to have a rutile structure having tetragonal phase with space group P42/mnm. The concluded data are in agreement with JCPDS file no. 41-1445. In addition to this, the diffraction peaks have been indexed to the characteristic planes (110), (101), (200), (210), (211), (002), and (310). The highest relative intensity peak was determined for (101) and (210) planes. The average crystallite NP size has been determined to be approximately 3–5 nm using the Debye–Scherrer equation. No characteristic peak was additionally observed, depicting the purity of the synthesized NPs. Thus, it can be concluded that rutile phase SnO2 NPs are formed having broad peaks which indicates smaller particle size. Furthermore, Fig. 1(b) depicts that all the characteristic peaks for SnO2 NPs are observed in the obtained XRD pattern for SnO2@SA beads. Besides this, a similar pattern is observed for SnO2@SA beads before and after adsorption as shown in ESI† (Fig. S3). The lowered intensities and the broadness of the peaks in the SnO2@SA beads can be attributed to dispersion of SnO2 NPs within the alginate polymer matrix.41 No additional peak confirms the purity of the beads. Thus, the crystallinity and purity of SnO2 NPs continue to be well-maintained after their successful encapsulation within SA beads. Similar observations have been reported by other studies based on encapsulation of nanoparticles within alginate beads.42,43
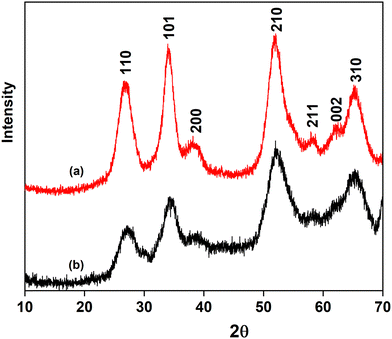 |
| Fig. 1 XRD spectra of (a) SnO2 NPs in a powder form and (b) SnO2 NPs encapsulated within SA beads. | |
3.2 Optical Study
The structural properties of pure SnO2 NPs were investigated using FTIR spectroscopic analysis. As shown in Fig. 2(a), the absorbance bands at 3348 cm−1 and 1621 cm−1 can be assigned to the hydroxyl group which may have resulted from the adsorbed water molecules on the surface of SnO2 NPs.44,45 The absorbance bands at 3348 cm−1 and the strong peak at 1621 cm−1 depict the stretching and bending mode of the OH groups, respectively.45 Below the fingerprint region of the IR spectra, the characteristic features of the SnO2 lattice can be highlighted by the presence of absorbance bands at 470 cm−1 which represent O–Sn–O stretching vibrations and the shoulder peaks at 639 cm−1, collectively marking the stretching vibrations of Sn–O bonds.16
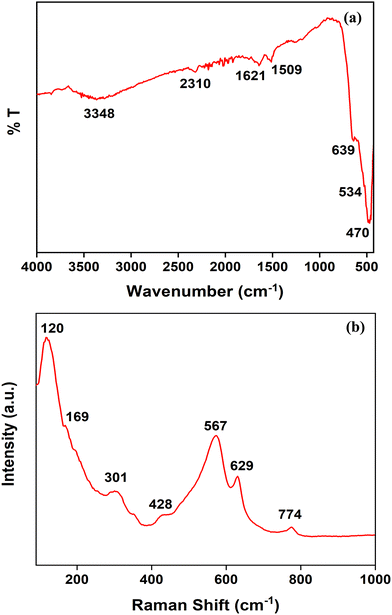 |
| Fig. 2 (a) FTIR spectra and (b) Raman spectra of SnO2 NPs. | |
The analysis of SnO2 NPs through Raman spectral data has been shown in Fig. 2(b). The spectrum of the synthesized SnO2 shows bands at 120 cm−1, 169 cm−1, 301 cm−1, 428 cm−1, 567 cm−1, 629 cm−1 and 774 cm−1. The band found at 629 cm−1 represents the SnO2 lattice structure, which is corroborated by the shoulder at 428 cm−1 reflecting the Sn–O–Sn linkage bending vibrations.23 Bridging and in-plane oxygen vacancies can be attributed to the bands at 567 cm−1 and 774 cm−1, respectively.16 Typically, a surface with oxygen vacancies exhibits high electron density, low adsorption energy, and rapid electron transfer.46,47 The oxygen vacancies may aid in creating additional new active binding sites for adsorption.48 Thus, the presence of oxygen vacancies on the synthesized nano-adsorbent can also be largely responsible for its high efficiency. Various similar studies have reported the presence of oxygen vacancies on metal oxides which lead to enhanced adsorption efficiency.48–50
3.3 Morphological study
The surface morphologies of SnO2 NPs and SnO2@SA beads were studied by scanning electron microscopy (SEM). Fig. 3(a) shows typical SEM images of the synthesized SnO2 NPs. The morphologies clearly depict the formation of various sizes of spherical NPs (ESI,† Fig. S4a). Additionally, TEM images shown in Fig. 4(a) and (b) indicate the crystalline nature of NPs with a lattice fringe spacing of 3.36 Å which can be indexed to the (110) plane of SnO2 NPs. It was observed that many lattice fringe spacings were within the approximate range of ≈3.34–3.63 Å. Also, elemental mapping of SnO2 NPs for confirmation of elemental composition analysis has been obtained which confirmed SnO2 NPs. The presence of each element has been highlighted, where the green color denotes the distribution of tin (Sn) and red denotes the distribution of oxygen (O) (Fig. 4(c) and (d)).
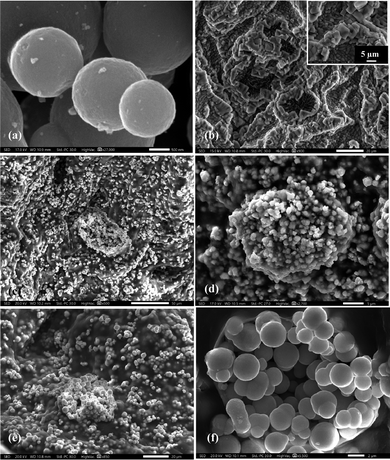 |
| Fig. 3 SEM images of (a) SnO2 NPs and (b) SA beads crosslinked by CaCl2 at 20 μm, with the inset representing the image taken at 5 μm; panels (c), (d), (e) and (f) show 0.5 g SnO2 encapsulated within SA beads at different magnification. | |
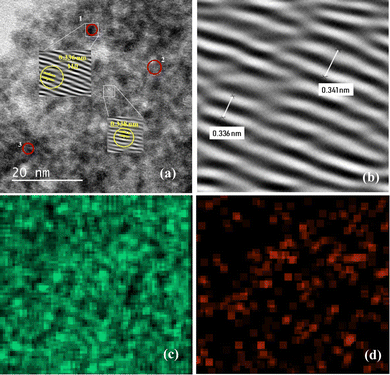 |
| Fig. 4 (a) Enlarged view of TEM images of SnO2 NPs showing various lattice fringes marked in a red circle; (b) magnified view of red circle 1 from image (a); and panels (c) and (d) show the elemental mapping of SnO2 NPs with Sn and O in green and red colors, respectively. | |
The morphological study of SnO2@SA beads has also been carried out through SEM imaging. Fig. 3(b), with a higher magnification image in the inset, shows the surface morphology of completely dried pure SA beads, which clearly indicates long crosslinked chains. Upon encapsulation of 0.5 g SnO2 within the SA beads, the surface morphology drastically changed as depicted in Fig. 3(c)–(f) which demonstrates that the spherically shaped SnO2 NPs have been successfully entrapped within SA beads. Additionally, the SEM images for the encapsulation of 0.2 g SnO2 within SA beads have been illustrated in ESI† (Fig. S4b–d) which clearly depict that at lower concentration (0.2 g SnO2@SA beads, Fig. S4b–d, ESI†) the NPs are sparsely spread and entrapped, while at high concentration (0.5 g SnO2@SA beads, Fig. 3(c)–(f)) the NPs are densely entrapped within SA beads.
4. Adsorption of anionic dyes
SnO2 NPs were utilized as nano-adsorbents to remove four different anionic dyes (Congo red, eosin yellow, methyl orange, and orange G) from their aqueous solutions. In Fig. 5, the removal efficiency of SnO2 NPs is depicted, revealing nearly complete removal (99.96%) of Congo red within 5 min of contact time. Moreover, the removal efficiency for eosin yellow after 30 min of contact time reaches approximately 82%. Additionally, 5.22% and 2.46% removal efficiencies are observed for orange G and methyl orange, respectively.
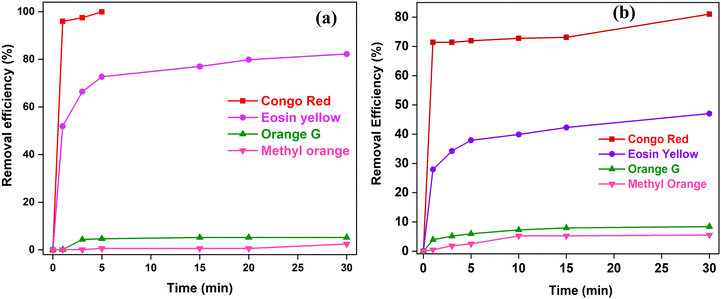 |
| Fig. 5 Adsorption of four anionic dyes at a concentration of 5 μM using (a) 0.1 g/100 mL of SnO2 NPs and (b) 0.3 g SnO2@SA beads. | |
Adsorption studies were also conducted to evaluate the versatility of SnO2@SA beads. The results, presented in Fig. 5(b), illustrate the adsorption capabilities of 0.3 g SnO2@SA beads for eosin yellow, orange G, and methyl orange, in addition to Congo red. After a contact time of 30 min, the adsorption efficiencies were found to be 81%, 47.05%, 8.39%, and 5.53% for Congo red, eosin yellow, orange G, and methyl orange, respectively. This clearly demonstrates that SnO2@SA beads possess the potential to effectively remove various anionic dyes, making them versatile candidates for wastewater treatment and environmental applications. The remarkable adsorption efficiency for Congo red and eosin yellow may also indicate the potential selectivity of the adsorbent for dyes with different molecular structure and charge densities. Besides, the moderate removal efficiencies for orange G and methyl orange indicate that SnO2@SA beads can be further fine-tuned for specific dye removal applications. Based on the highest efficiency observed for Congo red dye, further investigations were carried out using Congo red to examine the influence of various operational parameters, as well as to study adsorption kinetics and isotherm.
5. Adsorption efficiency of SnO2 nanoparticles
Various dye concentrations have been studied. The calibration curves and linear fit have been shown in ESI† (Fig. S5). The efficiency of adsorption by SnO2 NPs is shown in Fig. 6(a). Within the first minute, fast adsorption of the dye occurs, resulting in more than 90% adsorption of the dye (Fig. S6a, ESI†). This high rate of adsorption may be credited to the large surface area of SnO2 NPs, as confirmed through PXRD and SEM results. The removal efficiency (η) has been calculated using eqn (S1) discussed in the ESI† (S1).17,51 The results revealed that nearly complete adsorption of organic pollutant CR has been successfully carried out within 5 min for the organic azo dye without the application of external factors such as cocatalyst, sacrificial agent, heat and light.
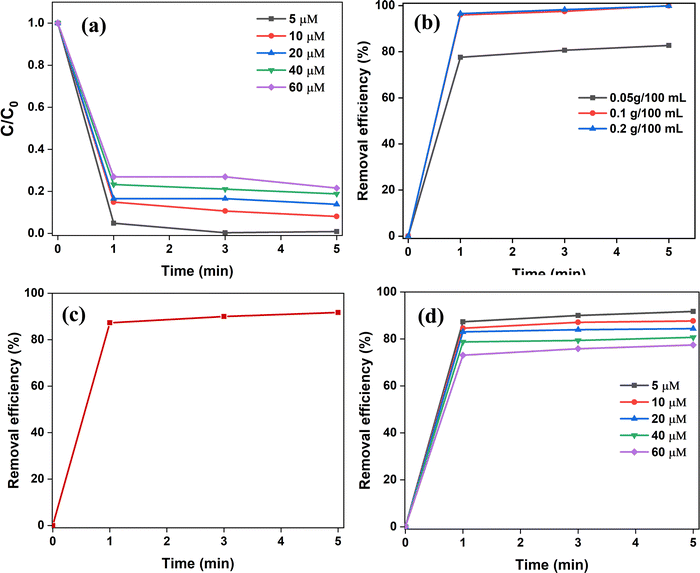 |
| Fig. 6 (a) Adsorption efficiencies of spherical SnO2 NPs as observed for various dye concentrations; (b) effect of adsorbent dosage on the removal efficiency for 5 μM CR at pH = 5; (c) effect of contact time upon the removal efficiency for 5 μM CR dye; and (d) effect of dye concentration on the removal efficiency for CR by 0.1 g/100 mL of SnO2 NPs. | |
In the adsorption of organic dyes, a major role is played by the surface chemistry of the adsorbents. The favorable enhanced adsorption of CR dye by the synthesized NPs is to a great degree due to the electrostatic interaction between the cationic center of SnO2 and the electron rich center of the CR dye.52 Another major factor that can influence adsorption efficiency is the formation of oxygen vacancies. The presence of oxygen vacancies on the SnO2 nano-adsorbent has been confirmed by Raman spectra, which are responsible for generating vacant active binding sites.16,48,50 Also, additional capture of CR moieties may occur through the formation of hydrogen bonds.53 This process is supported further by the fact that other reported positively charged adsorbents had significant adsorption capacity towards anionic dyes due to oxygen vacancies, electrostatic interactions and hydrogen bonding.2,10,26,37,54,55
5.1 Effect of adsorbent dose
At room temperature, the effect of adsorbent dosage has been investigated for 5 μM CR dye at different time intervals (0–5 min). The results are shown in Fig. 6(b), which indicates that increasing the adsorbent dose from 0.05 to 0.2 g/100 mL increases the percentage of adsorption for the entire contact time. This can be the consequence of increased surface area which further allows increased binding at active sites. The presence of additional adsorption active sites can also be responsible for the rise in adsorption effectiveness with the adsorbent dose.56 However, at 5 min, the removal efficiencies of 0.1 and 0.2 g SnO2 NPs are 99.96% and 99.83%, respectively. Since it does not indicate a significant difference, further investigations were carried out at 0.1 g/100 mL.
5.2 Effect of pH
pH is a significant parameter since it affects the adsorbent's surface charge, degree of ionization, and adsorbate speciation. Fig. 7 shows the influence of initial CR pH on the adsorption efficiency of SnO2. The adsorbent dosage was kept fixed at 0.1 g/100 mL. At a varying pH range from 3 to 11, the predicted strong pH influence has been demonstrated for two dye concentrations (5 and 10 μM). At an initial pH of 5, maximum removal efficiency (∼92%) for CR on SnO2 was observed (Fig. 7(a)). However, at a pH lower than 5, as the acidic nature increased, its removal efficiency vastly declined to a much lower level. This can be due to the face-to-face arrangement of CR monomers into anionic dimers, which further allows them to partially self-associate and reduce their hydrophobic contact with water.12 Thus, at highly acidic pH ranges (pH < 5) CR dye may undergo tautomerism and aggregation, thereby hindering adsorption efficiency.12 At pH > 5, the increase in pH demonstrated a decline in the removal efficiency which can be due to interionic repulsions between similarly charged particles and the increased OH− concentrations which compete for adsorption.57,58 Therefore, the adsorption investigations were studied at an optimum pH = 5.
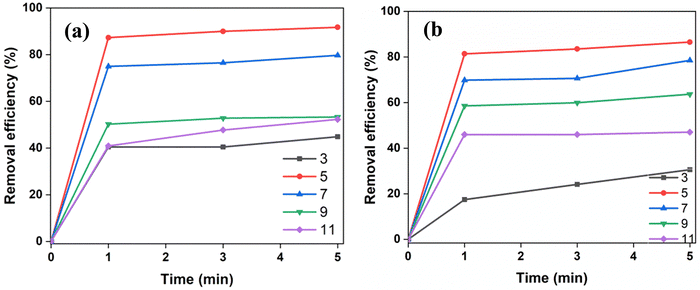 |
| Fig. 7 Effect of pH on removal efficiency for two dye concentrations: (a) 5 μM and (b) 10 μM. | |
5.3 Effect of contact time
As illustrated in Fig. 6(c), the adsorption of CR on SnO2 NPs was examined as a function of contact time to find the equilibrium for maximum adsorption. The adsorption rapidly increases within the 1st minute attaining more than 80% adsorption, after which it subsequently slows down as sorption equilibrium is attained after 5 min. Thus, it has been adopted as the optimum equilibrium time for the adsorption of CR dye on SnO2 NP's surface at a CR concentration of 5 μM, pH = 5, and adsorbent dose of 0.1 g/100 mL. The rapid adsorption of CR within the first minute of contact depicts the large number of free active binding sites that are conveniently available for CR molecules.17,51
5.4 Effect of dye concentration
In adsorption, it is necessary to overcome the mass transfer resistance of the dye between the aqueous and solid phases.59 This suggests that the initial concentration of the dye and its interaction with the adsorbent are crucial factors. In this context, the effect of initial dye concentration was studied for a range of 5–60 μM using a constant adsorbent dosage of 0.1 g/100 mL. Fig. 6(d) demonstrates that the increase of concentration from 5 to 60 μM resulted in a decreased percentage of dye removal efficiency. This is often related to the decrease in the number of vacant binding sites at high dye concentration, thereby lowering the adsorption driving force.60
6. Adsorption kinetics
The adsorption kinetics were determined at varying CR concentrations of 5–60 μM and at a fixed adsorbent dosage of 0.1 g/100 mL and pH = 5. For determining the appropriate model to describe the adsorption mechanism, the pseudo-first-order (PFO), pseudo-second-order (PSO) and intra-particle diffusion (IPD) models were applied (Fig. 8). The equations used for PFO, PSO and IPD have been discussed in the ESI† (S2).
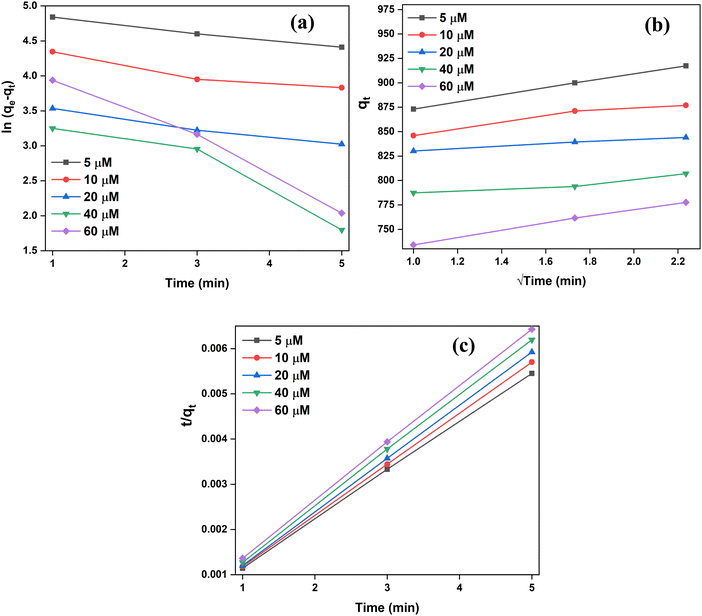 |
| Fig. 8 Adsorption kinetic models studied for varying dye concentrations (5–60 μM) and a constant adsorbent dose (0.1 g/100 mL): (a) pseudo-first-order, (b) intra-particle diffusion and (c) pseudo-second-order. | |
The most appropriately fit model has been determined from comparative analysis based on the obtained linear regression correlation coefficient (R2) values. The calculated kinetic parameters for each of the models have been summarized in Table 2. The results clearly depict that the PFO kinetics are not suitable for the present adsorption system due to the poor R2 values. Additionally, the large difference between the experimental and calculated values of qe demonstrated the poor fit of the PFO model. However, both PSO and IPD models demonstrated high R2 values. However, R2 values were more consistent and higher for PSO than for the IPD model. In addition to this, the calculated values of qe for the PSO model are very well in agreement with the experimental qe data. Secondly, the PSO kinetic model's curve-fitting plots demonstrated a straight line through the origin, validating it as the best fit model in the current system (Fig. 8(c)). This reflects that the adsorption mechanism involved in the adsorption of CR on SnO2 NPs is facilitated by the process of chemisorption. This implies that the rate determining step is the chemical sorption of CR on the adsorbent's surface which may be simultaneously accompanied by exchange of electrons between adsorbate and adsorbent.37
Table 2 Kinetic parameters obtained from CR adsorption on SnO2 NPs
Kinetic models |
5 μM |
10 μM |
20 μM |
40 μM |
60 μM |
q
e,exp (mg g−1) |
999.57 |
923.07 |
864.59 |
813.05 |
785.28 |
Pseudo-first-order |
R
2
|
0.9958 |
0.91193 |
0.99246 |
0.8948 |
0.98863 |
k
1 (min−1) |
0.1057 |
0.1285 |
0.12824 |
0.3638 |
0.4749 |
q
e,cal (mg g−1) |
138.37 |
83.76 |
38.09 |
42.52 |
87.35 |
|
Pseudo-second-order |
R
2
|
0.99991 |
1 |
0.99999 |
0.99988 |
0.9999 |
k
2 (g mg−1 min−1) |
0.0157 |
0.0246 |
0.0507 |
0.0283 |
0.0143 |
q
e,cal (mg g−1) |
929.925 |
884.95 |
847.45 |
813.00 |
793.65 |
|
Intra-particle diffusion |
R
2
|
0.99969 |
0.94341 |
0.99296 |
0.91478 |
0.99783 |
K
p (mg g−1 min−1/2) |
36.02 |
25.88 |
11.28 |
15.52 |
35.62 |
7. Adsorption isotherm studies
In order to understand the interactive model between the adsorbent and the adsorbate, it is critical to evaluate adsorption isotherms since they provide unique insight into the nature of interactions. In this context, the renowned Langmuir, Freundlich and Temkin models were studied. The equations have been discussed in the ESI† (S3).
Fig. 9(a)–(c) depicts the CR adsorption isotherms of SnO2 NPs for 5 μM CR at an adsorbent dosage of 0.1 g/100 mL having pH = 5. The R2 value for the Langmuir isotherm is high (0.99988), while those for Freundlich and Temkin are 0.91772 and 0.91787, respectively. The Langmuir isotherm model thus offered the most precise account of CR adsorption (Fig. 9(c)). Table S1 (ESI†) provides an overview of the three model's estimated parameters. The comparatively low R2 values of Freundlich and Temkin isotherm models, however, led to their rejection. This suggests that CR was adsorbed at predetermined homogeneous sites of the nano-adsorbent, resulting in the formation of a monolayer on the nano-adsorbent's surface. Similar results have been reported in various other recent investigations related to dye adsorption, indicating that PSO and Langmuir isotherms are most commonly reported.17,54,61–63 However, this does not eliminate the possibilities of adsorption kinetics and isotherms following other adsorption models.64–66
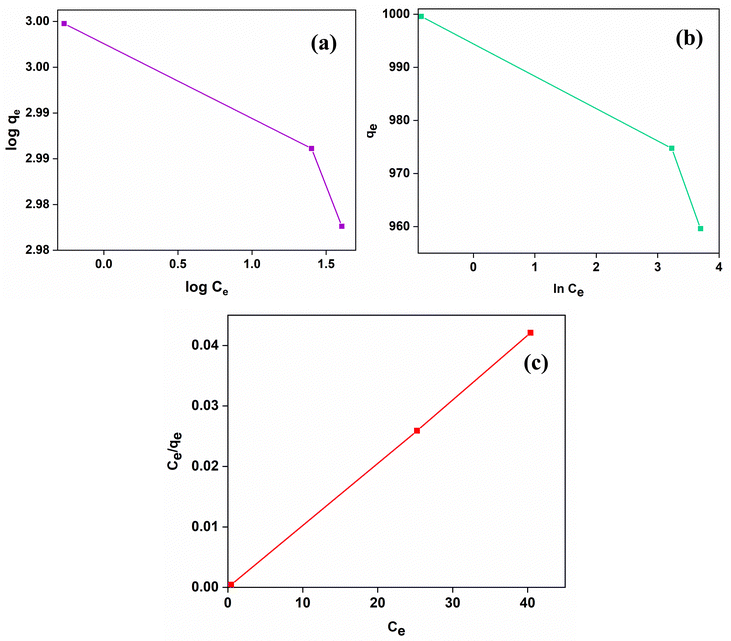 |
| Fig. 9 Adsorption isotherms for 5 μM CR adsorption by SnO2 at pH = 5 and room temperature. (a) Freundlich isotherm, (b) Temkin isotherm and (c) Langmuir isotherm. | |
The favorability of the adsorption isotherm was further determined by evaluating the dimensionless separation factor (RL), using eqn (S6) (ESI†).67,68 The adsorption process favorability is determined by 0 < RL < 1.10 It was determined that the value calculated for the adsorption of CR by the synthesized SnO2 NPs lies within the range of 0 to 1 (RL = 0.000113); thus the adsorption of CR on the adsorbent is determined to be favorable. Additionally, Table S1 (ESI†) compiles the information gathered from Freundlich isotherms, which clearly indicates that 1/n = 0.0083; hence the adsorption mechanism also fulfills the criteria for favorable reaction (0 < 1/n < 1).
8. Adsorption efficiency of encapsulated nanoparticles
Secondary pollutant generation due to metal leaching from the adsorbent and separation of the adsorbent from treated waters are two major challenges that need to be addressed for expanding the scope of pilot studies to practical implementation. To address these issues, SnO2 NPs were encapsulated within sodium alginate (SnO2@SA) beads. The adsorption efficiency of SnO2@SA beads was evaluated for varying concentrations of SnO2 (0.1 g, 0.2 g, and 0.5 g). However, to obtain a better comparative analysis, pure SA beads were first investigated for CR adsorption (Fig. 10(a)). For providing a clear perspective, the images of SnO2 NPs and the completely dried SnO2@SA beads are provided in the ESI† (Fig. S1). Additionally, Fig. S2 in the ESI† shows the images of the partially dried beads used for the adsorption studies. Fig. S1 (ESI†) illustrates the distinct color changes of the SnO2 NPs and dried SnO2@SA beads before and after adsorption. The white color of SnO2 NPs changes to a pale red color, while similar changes in color are also observed for the dried SnO2@SA beads. Thus, the adsorption of CR on both SnO2 NPs and SnO2@SA beads is quite evident through the color changes. Further analysis through UV-Visible spectroscopy has been carried out to clearly understand the comparative analysis of adsorption efficiency of SnO2@SA beads of three different concentrations.
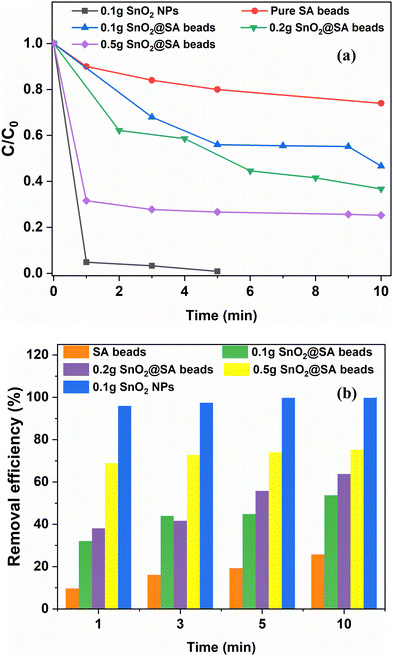 |
| Fig. 10 (a) Various compositions of SnO2@SA beads for CR dye adsorption, and (b) their comparative analysis through a bar diagram. | |
Pure SA beads demonstrated approximately 9% removal efficiency. Even after 10 min of exposure, the highest efficiency for pure SA beads is only about 25% (Fig. 10(a)). Upon encapsulating 0.1 g SnO2 within SA beads (0.1 g SnO2@SA beads), the efficiency increased to 32% within the first minute of contact. However, only 53% efficiency was attained after 10 min (Fig. S7, ESI†). Hence, 0.2 g and 0.5 g SnO2@SA beads were also investigated. Increment in efficiency (63.82%) is typically observed for 0.2 g SnO2@SA beads within 10 min (Fig. S8, ESI†), and nearly 75% efficiency is observed for 0.5 g SnO2@SA beads within 5 min (Fig. S6b and S9, ESI†). Also, Fig. 10(b) depicts the comparative analysis of adsorption efficiencies of SnO2 NPs and SnO2@SA beads of various compositions in the form of a bar diagram. This indicates that the encapsulated SnO2 NPs within SA beads can also show commendable efficiency when compared to SnO2 NPs.
8.1. Leaching analysis
To ascertain leachate (Sn) concentrations in treated water, ICP-MS was employed to detect inorganic Sn within the treated water after utilizing 0.5 g SnO2@SA beads, yielding a value of 7.729 ppb (parts per billion) or μg L−1. This indicates that the Sn concentration in the treated water accounted for approximately 0.00019% of initial Sn concentration present in the beads (S4 in ESI†).
Assessments carried out by the WHO reported inorganic Sn concentration within the range of 0.2–3 μg L−1 in seawater, <0.003 μg L−1 in rainwater, and 0.001–8 μg L−1 in polluted coastal waters. Furthermore, UK drinking water supplies revealed Sn concentration to be below 10 μg L−1, while an average concentration of 6 μg L−1 was reported in US municipal drinking water.69 The Joint FAO/WHO expert committee on Food Additives also reported that the maximum level of Sn in commodities such as canned food and beverages must not exceed 250 mg kg−1 and 150 mg kg−1, respectively.70 They also concluded that Sn concentration higher than 200 ppm can produce acute toxic effects in humans. Similarly, a study based in Perak, Malaysia, reported Sn concentration ranging between 1 and 7 μg L−1.71 More recently, the WHO released the 4th edition incorporating guidelines for drinking-water quality, which revealed that considering the minimal toxicity of reported Sn concentration, the establishment of permissible levels for Sn has been deemed unnecessary.72 Consequently, the direct comparison of measured Sn concentrations in treated water using SnO2@SA beads based on existing literature is not feasible. Nonetheless, it can be surmised that the detected Sn concentration identified in this work remains lower in comparison to the benchmarks reported in the existing literature. Thus, the pragmatic application of these beads as nano-adsorbents exhibits significant potential for treating dye wastewater. However, scaling up of these nanosorbents requires further in-depth exploration which will be performed to ensure complete mitigation of Sn leaching in treated water.
9. Regenerative studies
The stability of an adsorbent plays a crucial role when it undergoes repeated adsorption–desorption cycles. To establish the reusability, feasibility and cost-effectiveness of SnO2@SA beads were assessed through chemical regeneration using solvent desorption. This method of regeneration has substantial advantages as it has an environmentally benign nature, low-cost, and rapid regeneration rate. Fig. 11 presents comparative desorption efficiencies of acetone, methanol, ethanol and dil. HCl. Acetone and methanol exhibited the highest desorption efficiency, surpassing ethanol and dil. HCl significantly. However, considering the highly volatile nature of acetone, which may lead to incomplete desorption due to rapid evaporation, methanol was selected as the preferred eluent for further regeneration studies.
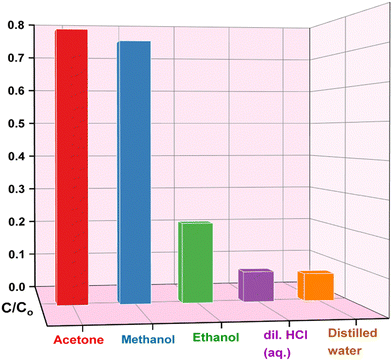 |
| Fig. 11 Bar diagram showing the concentration of desorbed Congo red in various eluents from the adsorbent. | |
SnO2@SA beads were used to perform three consecutive cycles using methanol as an eluent (Fig. S10, ESI†) and the results are shown in Fig. 12. The amount of CR adsorption gradually decreases as the number of cycles increases. The amount of adsorbed dye that was not recovered by desorption was likely present on the beads, which caused a gradual reduction in adsorption capacity in the following cycles.6 The lowered efficiency may be attributed to the unavailable or attenuated vacant adsorption sites during desorption.35 The CR adsorption capacity of 0.5 g SnO2@SA beads decreased from 75.3% to 18.9% after two cycles, while that of 0.1 g SnO2@SA beads decreased from 53.7% to 15.1% after two cycles (Fig. 12). As a result, it can be inferred that SnO2@SA beads are promising environmentally friendly nano-adsorbents for treatment of CR dye. However, more investigation is required to identify the ideal eluent to boost the efficacy of the reusability of SnO2@SA beads.
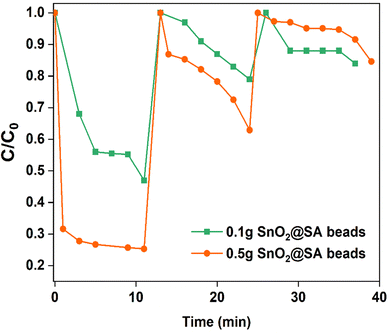 |
| Fig. 12 Regenerative studies of 0.5 g and 0.1 g SnO2@SA beads for 5 μM CR aqueous solution. | |
10. Comparison with other adsorbents
Various adsorbents such as activated carbon obtained from agricultural waste biomass,73 walnut shell74 and carboxymethyl cellulose,75 metal organic frameworks like UiO-6613 and even starch based hydrogels76 have been fabricated for the adsorption of CR and many other dyes such as methylene blue. The comparison of the presented work using SnO2 NPs with other adsorbents used for the treatment of CR dye is shown in Table 3. It clearly indicates that the maximum adsorption capacity of SnO2 NPs is quite high and even better than that of some of the reported studies. Abdelkader et al. reported 84.41% Congo red removal efficiency after 60 min of contact time using SnO2 NPs which were synthesized at 450 and 650 °C.17 In contrast, the current study achieved significantly higher efficiency, demonstrated regenerative abilities, and showcased versatility in adsorbing multiple anionic dyes by SnO2 NPs synthesized at a comparatively low temperature, even when encapsulated within sodium alginate beads. Therefore, these nano-adsorbents can be considered as of great potential for the treatment of anionic dyes. Moreover, the successful implementation of SnO2 NPs encapsulated within SA beads can provide immense control over the leaching of metal from the nano-adsorbent, thereby proving them to be a biocompatible, highly efficient and economical adsorbent.
Table 3 Comparison of various adsorbents used for removal of CR dye
Adsorbent |
Adsorbent dosage |
Adsorption capacity, qm (mg g−1) |
Isotherm |
Kinetic model |
Ref. |
Cd4(H4L)2(phen)2(H2O)4 |
3000 g mL−1 |
684 |
Langmuir |
Pseudo-second order |
77
|
Activated carbon from water hyacinth stem |
16 g L−1 |
14.367 |
Langmuir |
Pseudo-second order |
78
|
Cyanoguanidine-modified chitosan |
50 mg/50 mL |
666.67 |
Langmuir |
Pseudo-second order |
79
|
AlF-rGO |
0.1 g/25 mL |
178.57 |
Freundlich |
Pseudo-second order |
80
|
Vernonia amygdalina leaf powder |
5 g L−1 |
57.47 |
Langmuir |
Pseudo-second order |
81
|
Fe3O4/graphene oxide composite |
— |
769.2 |
Langmuir |
Pseudo-second order |
67
|
CoFe2−xGdxO4 |
— |
263.2 |
Langmuir |
Pseudo-second order |
82
|
Fe3O4@SiO2-COOH NPs |
0.5 g L−1 |
263.16 |
Langmuir |
Pseudo-second order |
51
|
SnO2 NPs |
1 g L−1 |
48.31 |
Langmuir |
Pseudo-second order |
17
|
SnO2 NPs |
0.1 g/100 mL |
961.54 |
Langmuir |
Pseudo-second order |
This work |
11. Conclusion
In this study, hydrothermally synthesized spherical SnO2 NPs were successfully prepared without any toxic reagents. PXRD, TEM, FTIR and Raman spectroscopy confirmed the formation of SnO2 NPs. SEM imaging confirmed the formation of spherical NPs and the encapsulation of SnO2 NPs in sodium alginate beads. Within 1 min, nearly 95% adsorption efficiency is observed for 5 μM CR dye by 0.1 g/100 mL of SnO2 NPs. Favorable adsorption is determined to be at pH = 5, while adsorption decreases at highly acidic and basic pH. The adsorption behavior can be best described by pseudo-second-order kinetics. The equilibrium data displayed the best fit of the Langmuir isotherm and the maximum adsorption capacity was determined to be 961.5 mg g−1. The driving forces can be attributed to oxygen vacancies, electrostatic force of attraction and hydrogen bonding, and the adsorption mechanism involved chemisorption and monolayer formation on adsorbent's surface. Finally, the encapsulated SnO2 NPs within sodium alginate beads were also capable of behaving as nano-adsorbents for CR dye removal, which also showed successful regeneration. Additionally, nearly 0.0019% of Sn concentration from the adsorbent material was detected in aqueous solution after treating dyes, which indicates significantly reduced metal leaching due to encapsulation of SnO2 in alginate beads. Moreover, the noteworthy adsorption capabilities of SnO2@SA beads extend beyond Congo red dye, as they also demonstrate adsorption of eosin yellow, orange G and methyl orange. In conclusion, SnO2@SA beads have high potential in dye wastewater treatment due to their ease of preparation, environmental friendliness, and cost-effectiveness. Nevertheless, efforts to improve the effectiveness of SnO2@SA beads through doping or the production of nanocomposites can be pursued in future studies.
Declaration of generative AI in scientific writing
Not applicable.
Conflicts of interest
The authors declare that they have no conflict of interest.
Acknowledgements
The author S. J. B is grateful to Jawaharlal Nehru University, New Delhi, for providing financial assistance. This research did not receive any specific grant from funding agencies in the public, commercial, or not-for-profit sectors.
References
- E. J. R. Almeida and C. R. Corso, Decolorization and removal of toxicity of textile azo dyes using fungal biomass pelletized, Int. J. Environ. Sci. Technol., 2019, 16, 1319–1328 CrossRef CAS.
- Y. Zheng, B. Cheng, J. Fan, J. Yu and W. Ho, Review on nickel-based adsorption materials for Congo red, J. Hazard. Mater., 2021, 403, 123559 CrossRef CAS PubMed.
- T. N. J. I. Edison, R. Atchudan, M. G. Sethuraman and Y. R. Lee, Reductive-degradation of carcinogenic azo dyes using Anacardium occidentale testa derived silver nanoparticles, J. Photochem. Photobiol., B, 2016, 162, 604–610 CrossRef CAS PubMed.
- R. G. Saratale, G. D. Saratale, J.-S. Chang and S. P. Govindwar, Bacterial decolorization and degradation of azo dyes: a review, J. Taiwan Inst. Chem. Eng., 2011, 42, 138–157 CrossRef CAS.
- S.-S. Yang, J.-H. Kang, T.-R. Xie, L. He, D.-F. Xing, N.-Q. Ren, S.-H. Ho and W.-M. Wu, Generation of high-efficient biochar for dye adsorption using frass of yellow mealworms (larvae of Tenebrio molitor Linnaeus) fed with wheat straw for insect biomass production, J. Cleaner Prod., 2019, 227, 33–47 CrossRef CAS.
- V. S. Munagapati and D.-S. Kim, Equilibrium isotherms, kinetics, and thermodynamics studies for congo red adsorption using calcium alginate beads impregnated with nano-goethite, Ecotoxicol. Environ. Saf., 2017, 141, 226–234 CrossRef CAS PubMed.
- R. K. Sonwani, G. Swain, B. S. Giri, R. S. Singh and B. N. Rai, Biodegradation of Congo red dye in a moving bed biofilm reactor: Performance evaluation and kinetic modeling, Bioresour. Technol., 2020, 302, 122811 CrossRef CAS PubMed.
- S. Chakraborty, J. J. Farida, R. Simon, S. Kasthuri and N. L. Mary, Averrhoe carrambola fruit extract assisted green synthesis of zno nanoparticles for the photodegradation of congo red dye, Surf. Interfaces, 2020, 19, 100488 CrossRef CAS.
- N. P. Khumalo, L. N. Nthunya, E. De Canck, S. Derese, A. R. Verliefde, A. T. Kuvarega, B. B. Mamba, S. D. Mhlanga and D. S. Dlamini, Congo red dye removal by direct membrane distillation using PVDF/PTFE membrane, Sep. Purif. Technol., 2019, 211, 578–586 CrossRef CAS.
- J. Liu, N. Wang, H. Zhang and J. Baeyens, Adsorption of Congo red dye on FexCo3−xO4 nanoparticles, J. Environ. Manage., 2019, 238, 473–483 CrossRef CAS PubMed.
- S. Yu, J. Wang and J. Cui, Preparation of a novel chitosan-based magnetic adsorbent CTS@ SnO2@Fe3O4 for effective treatment of dye wastewater, Int. J. Biol. Macromol., 2020, 156, 1474–1482 CrossRef CAS PubMed.
- Z. L. Yaneva and N. V. Georgieva, Insights into Congo Red Adsorption on Agro-Industrial Materials-Spectral, Equilibrium, Kinetic, Thermodynamic, Dynamic and Desorption Studies. A Review, Int. Rev. Chem. Eng., 2012, 4, 127–146 Search PubMed.
- F. Ahmadijokani, R. Mohammadkhani, S. Ahmadipouya, A. Shokrgozar, M. Rezakazemi, H. Molavi, T. M. Aminabhavi and M. Arjmand, Superior chemical stability of UiO-66 metal-organic frameworks (MOFs) for selective dye adsorption, Chem. Eng. J., 2020, 399, 125346 CrossRef CAS.
- Q. Du, J. Sun, Y. Li, X. Yang, X. Wang, Z. Wang and L. Xia, Highly enhanced adsorption of congo red onto graphene oxide/chitosan fibers by wet-chemical etching off silica nanoparticles, Chem. Eng. J., 2014, 245, 99–106 CrossRef CAS.
- Bhawna, S. Kumar, R. Sharma, A. Gupta, A. Tyagi, P. Singh, A. Kumar and V. Kumar, Recent insights into SnO2-based engineered nanoparticles for sustainable H2 generation and remediation of pesticides, New J. Chem., 2022, 46, 4014–4048 RSC.
- S. Kumar, Bhawna, S. K. Yadav, A. Gupta, R. Kumar, J. Ahmed, M. Chaudhary, Suhas and V. Kumar, B-doped
SnO2 nanoparticles: a new insight into the photocatalytic hydrogen generation by water splitting and degradation of dyes, Environ. Sci. Pollut. Res., 2022, 29, 47448–47461 CrossRef CAS PubMed.
- E. Abdelkader, L. Nadjia and V. Rose-Noëlle, Adsorption of Congo red azo dye on nanosized SnO2 derived from sol–gel method, Int. J. Ind. Chem., 2016, 7, 53–70 CrossRef CAS.
- H.-C. Chiu and C.-S. Yeh, Hydrothermal synthesis of SnO2 nanoparticles and their gas-sensing of alcohol, J. Phys. Chem. C, 2007, 111, 7256–7259 CrossRef CAS.
- G. X. Wang, Y. Chen, L. Yang, J. Yao, S. Needham, H. K. Liu and J. H. Ahn, Synthesis of nanocrystalline transition metal and oxides for lithium storage, J. Power Sources, 2005, 146, 487–491 CrossRef CAS.
- N. Talebian and H. S. H. Zavvare, Enhanced bactericidal action of SnO2 nanostructures having different morphologies under visible light: influence of surfactant, J. Photochem. Photobiol., B, 2014, 130, 132–139 CrossRef CAS PubMed.
- G. Korotcenkov, Nanomaterials, 2020, 10, 1392 CrossRef CAS PubMed.
- P. G. Jamkhande, N. W. Ghule, A. H. Bamer and M. G. Kalaskar, Metal nanoparticles synthesis: An overview on methods of preparation, advantages and disadvantages, and applications, J. Drug Delivery Sci. Technol., 2019, 53, 101174 CrossRef CAS.
- V. Kumar, K. Yadav, A. Gupta and B. Dwivedi, Facile Synthesis of Ce – Doped SnO2 Nanoparticles: A Promising Photocatalyst for Hydrogen Evolution and Dyes Degradation, ChemistrySelect, 2019, 3722–3729 CrossRef.
- Y. Wang, X. Jiang and Y. Xia, A solution-phase, precursor route to polycrystalline SnO2 nanowires that can be used for gas sensing under ambient conditions, J. Am. Chem. Soc., 2003, 125, 16176–16177 CrossRef CAS PubMed.
- V. Kumar and R. Nagarajan, Thermoluminescence in heavily F-doped of SnO2 nanocrystals, Chem. Phys. Lett., 2012, 530, 98–101 CrossRef CAS.
- J. P. Dhal, B. G. Mishra and G. Hota, Fe2O3–SnO2 composite nanorods: Facile synthesis and sorption properties, J. Environ. Chem. Eng., 2014, 2, 2188–2198 CrossRef CAS.
- A. F. Beevi, G. Sreekala and B. Beena, Synthesis, characterization and photocatalytic activity of SnO2, ZnO nanoparticles against congo red: A comparative study, Mater. Today: Proc., 2021, 45, 4045–4051 CrossRef.
- X. Chen, F. Zhang, Q. Wang, X. Han, X. Li, J. Liu, H. Lin and F. Qu, The synthesis of ZnO/SnO2 porous nanofibers for dye adsorption and degradation, Dalton Trans., 2015, 44, 3034–3042 RSC.
- C. M. Ma, G. B. Hong and S. C. Lee, Catalysts, 2020, 10 CAS.
- M. Maruthupandy, T. Muneeswaran, G. Chackaravarthi, T. Vennila, M. Anand, W.-S. Cho and F. Quero, Synthesis of chitosan/SnO2 nanocomposites by chemical precipitation for enhanced visible light photocatalytic degradation efficiency of congo red and rhodamine-B dye molecules, J. Photochem. Photobiol., A, 2022, 430, 113972 CrossRef CAS.
- D. Zhao and X. Wu, Nanoparticles assembled SnO2 nanosheet photocatalysts for wastewater purification, Mater. Lett., 2018, 210, 354–357 CrossRef CAS.
- V. Rocher, A. Bee, J.-M. Siaugue and V. Cabuil, Dye removal from aqueous solution by magnetic alginate beads crosslinked with epichlorohydrin, J. Hazard. Mater., 2010, 178, 434–439 CrossRef CAS PubMed.
- M. A. El-Bindary, I. M. El-Deen and A. F. Shoair, Removal of anionic dye from aqueous solution using magnetic sodium alginate beads, J. Mater. Environ. Sci., 2019, 10, 604–617 CAS.
- E. Tao, D. Ma, S. Yang and X. Hao, Graphene oxide-montmorillonite/sodium alginate aerogel beads for selective adsorption of methylene blue in wastewater, J. Alloys Compd., 2020, 832, 154833 CrossRef CAS.
- X. Zhao, X. Wang and T. Lou, Preparation of fibrous chitosan/sodium alginate composite foams for the adsorption of cationic and anionic dyes, J. Hazard. Mater., 2021, 403, 124054 CrossRef CAS PubMed.
- H. Rezaei, M. Haghshenasfard and A. Moheb, Optimization of dye adsorption using Fe3O4 nanoparticles encapsulated with alginate beads by Taguchi method, Adsorpt. Sci. Technol., 2017, 35, 55–71 CrossRef CAS.
- Y. Feng, H. Wang, J. Xu, X. Du, X. Cheng, Z. Du and H. Wang, Fabrication of MXene/PEI functionalized sodium alginate aerogel and its excellent adsorption behavior for Cr(VI) and Congo Red from aqueous solution, J. Hazard. Mater., 2021, 416, 125777 CrossRef CAS PubMed.
- M. E. González-López, C. M. Laureano-Anzaldo, A. A. Pérez-Fonseca, C. Gómez and J. R. Robledo-Ortíz, Congo red adsorption with cellulose-graphene nanoplatelets beads by differential column batch reactor, J. Environ. Chem. Eng., 2021, 9, 105029 CrossRef.
- H. S. Hassan, M. F. Elkady, A. H. El-Shazly and H. S. Bamufleh, Formulation of synthesized zinc oxide nanopowder into hybrid beads for dye separation, J. Nanomater., 2014, 2014, 6 Search PubMed.
- S. Yadav, A. Asthana, A. K. Singh, R. Chakraborty, S. Sree Vidya, A. Singh and S. A. C. Carabineiro, Methionine-functionalized graphene oxide/sodium alginate bio-polymer nanocomposite hydrogel beads: synthesis, isotherm and kinetic studies for an adsorptive removal of fluoroquinolone antibiotics, Nanomaterials, 2021, 11, 568 CrossRef CAS PubMed.
- M. Amiri, M. Salavati-Niasari, A. Pardakhty, M. Ahmadi and A. Akbari, Caffeine: A novel green precursor for synthesis of magnetic CoFe2O4 nanoparticles and pH-sensitive magnetic alginate beads for drug delivery, Mater. Sci. Eng., C, 2017, 76, 1085–1093 CrossRef CAS PubMed.
- X. Yi, J. He, Y. Guo, Z. Han, M. Yang, J. Jin, J. Gu, M. Ou and X. Xu, Encapsulating Fe3O4 into calcium alginate coated chitosan hydrochloride hydrogel beads for removal of Cu(II) and U(VI) from aqueous solutions, Ecotoxicol. Environ. Saf., 2018, 147, 699–707 CrossRef CAS PubMed.
- S. Periyasamy, V. Gopalakannan and N. Viswanathan, Hydrothermal assisted magnetic nano-hydroxyapatite encapsulated alginate beads for efficient Cr(VI) uptake from water, J. Environ. Chem. Eng., 2018, 6, 1443–1454 CrossRef CAS.
- F. Gu, S. F. Wang, C. F. Song, M. K. Lü, Y. X. Qi, G. J. Zhou, D. Xu and D. R. Yuan, Synthesis and luminescence properties of SnO2 nanoparticles, Chem. Phys. Lett., 2003, 372, 451–454 CrossRef CAS.
- R. Scipioni, D. Gazzoli, F. Teocoli, O. Palumbo, A. Paolone, N. Ibris, S. Brutti and M. A. Navarra, Membranes, 2014, 4, 123–142 CrossRef PubMed.
- N. Zhang, E. P. Tsang, J. Chen, Z. Fang and D. Zhao, Critical role of oxygen vacancies in heterogeneous Fenton oxidation over ceria-based catalysts, J. Colloid Interface Sci., 2020, 558, 163–172 CrossRef PubMed.
- J. Liu, O. Margeat, W. Dachraoui, X. Liu, M. Fahlman and J. Ackermann, Gram-scale synthesis of ultrathin tungsten oxide nanowires and their aspect ratio-dependent photocatalytic activity, Adv. Funct. Mater., 2014, 24, 6029–6037 CrossRef CAS.
- N. Cao, X. Zhao, M. Gao, Z. Li, X. Ding, C. Li, K. Liu, X. Du, W. Li, J. Feng, Y. Ren and T. Wei, Superior selective adsorption of MgO with abundant oxygen vacancies to removal and recycle reactive dyes, Sep. Purif. Technol., 2021, 275, 119236 CrossRef CAS.
- Y.-F. Sun, J.-J. Li, F. Xie, Y. Wei and M. Yang, Ruthenium-loaded cerium dioxide nanocomposites with rich oxygen vacancies promoted the highly sensitive electrochemical detection of Hg(II), Sens. Actuators, B, 2020, 320, 128355 CrossRef CAS.
- P. Ren, X. Ren, J. Xu, H. Li, Y. Zheng, Y. Hong, Y. Lin, Y. Zhou, Y. Chen and W. Zhang, Excellent adsorption property and mechanism of oxygen vacancies-assisted hexagonal MoO3 nanosheets for methylene blue and rhodamine b dyes, Appl. Surf. Sci., 2022, 597, 153699 CrossRef CAS.
- M. Mohammadi Galangash, M. Mohaghegh Montazeri, A. Ghavidast and M. Shirzad-Siboni, Synthesis of carboxyl-functionalized magnetic nanoparticles for adsorption of malachite green from water: Kinetics and thermodynamics studies, J. Chin. Chem. Soc., 2018, 65, 940–950 CrossRef CAS.
- G. Sangami and N. Dharmaraj, UV-visible spectroscopic estimation of photodegradation of rhodamine-B dye using tin(IV) oxide nanoparticles, Spectrochim. Acta, Part A, 2012, 97, 847–852 CrossRef CAS PubMed.
- X. Liu, Z. Zhang, W. Shi, Y. Zhang, S. An and L. Zhang, Adsorbing properties of magnetic nanoparticles Mn-ferrites on removal of Congo red from aqueous solution, J. Dispersion Sci. Technol., 2015, 36, 462–470 CrossRef CAS.
- M. P. Rao, A. K. Akhila, J. J. Wu, A. M. Asiri and S. Anandan, Synthesis, characterization and adsorption properties of Cu2V2O7 nanoparticles, Solid State Sci., 2019, 92, 13–23 CrossRef CAS.
- T. Mahmood, U. Noreen, R. Ali, A. Ullah, A. Naeem and M. Aslam, Adsorptive removal of Congo red from aqueous phase using graphene–tin oxide composite as a novel adsorbent, Int. J. Environ. Sci. Technol., 2022, 19, 10275–10290 CrossRef CAS.
- J.-S. Cao, J.-X. Lin, F. Fang, M.-T. Zhang and Z.-R. Hu, A new absorbent by modifying walnut shell for the removal of anionic dye: kinetic and thermodynamic studies, Bioresour. Technol., 2014, 163, 199–205 CrossRef CAS PubMed.
- R. Sivaraj, C. Namasivayam and K. Kadirvelu, Orange peel as an adsorbent in the removal of acid violet 17 (acid dye) from aqueous solutions, Waste Manag., 2001, 21, 105–110 CrossRef CAS PubMed.
- S. Mishra, D. J. Prakash and G. Ramakrishna, Characterization and utilization of mahua oil cake–A new adsorbent for the removal of congored dye from aqueous phase, Electron. J. Environ., Agric. Food Chem., 2009, 8, 425–436 CAS.
- Y. Önal, C. Akmil-Başar and Ç. Sarıcı-Özdemir, Investigation kinetics mechanisms of adsorption malachite green onto activated carbon, J. Hazard. Mater., 2007, 146, 194–203 CrossRef PubMed.
- M. Bhaumik, T. Y. Leswifi, A. Maity, V. V. Srinivasu and M. S. Onyango, Removal of fluoride from aqueous solution by polypyrrole/Fe3O4 magnetic nanocomposite, J. Hazard. Mater., 2011, 186, 150–159 CrossRef CAS PubMed.
- Y. Tang, X. Zhang, X. Li, J. Bai, C. Yang, Y. Zhang, Z. Xu, X. Jin and Y. Jiang, Facile synthesis of magnetic ZnAl layered double hydroxides and efficient adsorption of malachite green and Congo red, Sep. Purif. Technol., 2023, 322, 124305 CrossRef CAS.
- N. Ahmad, F. Suryani Arsyad, I. Royani, P. Mega Syah Bahar Nur Siregar, T. Taher and A. Lesbani, High regeneration of ZnAl/NiAl-Magnetite humic acid for adsorption of Congo red from aqueous solution, Inorg. Chem. Commun., 2023, 150, 110517 CrossRef CAS.
- M. T. Moustafa, Preparation and characterization of low-cost adsorbents for the efficient removal of malachite green using response surface modeling and reusability studies, Sci. Rep., 2023, 13, 4493 CrossRef CAS PubMed.
- R. El yousfi, N. Achalhi, A. Mohamed, A. Benahmed and A. El Idrissi, Synthesis, characterization of multi-arm copolymers and linear blocks based on PEG and PCL: Effect of topology on dye adsorption, Mater. Today: Proc., 2023, 72, 3650–3661 CrossRef CAS.
- P. K. Rose, R. Kumar, R. Kumar, M. Kumar and P. Sharma, Congo red dye adsorption onto cationic amino-modified walnut shell: Characterization, RSM optimization, isotherms, kinetics, and mechanism studies, Groundw. Sustain. Dev., 2023, 21, 100931 CrossRef.
- V. Watwe, S. Kulkarni and P. Kulkarni, Development of dried
uncharred leaves of Ficus benjamina as a novel adsorbent for cationic dyes: Kinetics, isotherm, and batch optimization, Ind. Crops Prod., 2023, 195, 116449 CrossRef CAS.
- H. Jiang, Y. Cao, F. Zeng, Z. Xie and F. He, A Novel Fe3O4/Graphene Oxide Composite Prepared by Click Chemistry for High-Efficiency Removal of Congo Red from Water, J. Nanomater., 2021, 2021, 9716897 Search PubMed.
- I. Langmuir, The adsorption of gases on plane surfaces of glass, mica and platinum, J. Am. Chem. Soc., 1918, 40, 1361–1403 CrossRef CAS.
-
A. Boehncke, G. Koennecker, I. Mangelsdorf and A. Wibbertmann, Concise international chemical assessment document 6, 1999 Search PubMed.
- Codex Alimantarius, CXS 193-1995, 2019.
- N. Rahmanian, S. H. B. Ali, M. Homayoonfard, N. J. Ali, M. Rehan, Y. Sadef and A. S. Nizami, Analysis of Physiochemical Parameters to Evaluate the Drinking Water Quality in the State of Perak, Malaysia, J. Chem., 2015, 2015, 716125 Search PubMed.
- WHO, Water Guidelines – Chemical Facts, 2010.
- H. Xue, X. Wang, Q. Xu, F. Dhaouadi, L. Sellaoui, M. K. Seliem, A. Ben Lamine, H. Belmabrouk, A. Bajahzar, A. Bonilla-Petriciolet, Z. Li and Q. Li, Adsorption of methylene blue from aqueous solution on activated carbons and composite prepared from an agricultural waste biomass: A comparative study by experimental and advanced modeling analysis, Chem. Eng. J., 2022, 430, 132801 CrossRef CAS.
- Z. Li, H. Hanafy, L. Zhang, L. Sellaoui, M. Schadeck Netto, M. L. S. Oliveira, M. K. Seliem, G. Luiz Dotto, A. Bonilla-Petriciolet and Q. Li, Adsorption of congo red and methylene blue dyes on an ashitaba waste and a walnut shell-based activated carbon from aqueous solutions: Experiments, characterization and physical interpretations, Chem. Eng. J., 2020, 388, 124263 CrossRef CAS.
- H. Wang, Z. Li, S. Yahyaoui, H. Hanafy, M. K. Seliem, A. Bonilla-Petriciolet, G. Luiz Dotto, L. Sellaoui and Q. Li, Effective adsorption of dyes on an activated carbon prepared from carboxymethyl cellulose: Experiments, characterization and advanced modelling, Chem. Eng. J., 2021, 417, 128116 CrossRef CAS.
- L. Chen, Y. Zhu, Y. Cui, R. Dai, Z. Shan and H. Chen, Fabrication of starch-based high-performance adsorptive hydrogels using a novel effective pretreatment and adsorption for cationic methylene blue dye: Behavior and mechanism, Chem. Eng. J., 2021, 405, 126953 CrossRef CAS.
- J. Ai, H.-R. Tian, X. Min, Z.-C. Wang and Z.-M. Sun, A fast and highly selective Congo red adsorption material based on a cadmium-phosphonate network, Dalton Trans., 2020, 49, 3700–3705 RSC.
- A. Extross, A. Waknis, C. Tagad, V. V. Gedam and P. D. Pathak, Adsorption of congo red using carbon from leaves and stem of water hyacinth: equilibrium, kinetics, thermodynamic studies, Int. J. Environ. Sci. Technol., 2023, 20, 1607–1644 CrossRef CAS.
- N. F. Al-Harby, E. F. Albahly and N. A. Mohamed, Polymers, 2021, 13, 4446 CrossRef CAS PubMed.
- R. Azhdari, S. M. Mousavi, S. A. Hashemi, S. Bahrani and S. Ramakrishna, Decorated graphene with aluminum fumarate metal organic framework as a superior non-toxic agent for efficient removal of Congo Red dye from wastewater, J. Environ. Chem. Eng., 2019, 7, 103437 CrossRef CAS.
- D. Zewde and B. Geremew, Removal of Congo red using Vernonia amygdalina leaf powder: optimization, isotherms, kinetics, and thermodynamics studies, Environ. Pollut. Bioavailability, 2022, 34, 88–101 CrossRef CAS.
- X. Zhao, W. Wang, Y. Zhang, S. Wu, F. Li and J. P. Liu, Synthesis and characterization of gadolinium doped cobalt ferrite nanoparticles with enhanced adsorption capability for Congo Red, Chem. Eng. J., 2014, 250, 164–174 CrossRef CAS.
|
This journal is © The Royal Society of Chemistry 2023 |
Click here to see how this site uses Cookies. View our privacy policy here.