DOI:
10.1039/D3MA00469D
(Paper)
Mater. Adv., 2023,
4, 5149-5159
Effects of hydrocarbon substituents on highly fluorescent bis(4-phenylphenyl)pyridylmethyl radical derivatives†
Received
26th July 2023
, Accepted 21st September 2023
First published on 26th September 2023
Abstract
Eight new stable luminescent radicals are reported. We previously reported that the photoluminescence quantum yields (PLQYs) of diphenylpyridylmethyl radicals is dramatically increased by the addition of mesityl (2,4,6-trimethylphenyl) groups. By changing the mesityl groups to other hydrocarbon-substituted phenyl groups, the issue of the fluoresence efficiency and photostability of these radicals could be clarified. Contrary to our expectations, changing 2,4,6-trimethyl to 2,4,6-triisopropyl did not produce a positive effect, other than some improvement in purification. Nevertheless, a PLQY of more than 60% was a significant value. The addition of methyl substituents at the meta-positions drastically quenched the fluorescence in dichloromethane, while maintaining bright fluorescence in chloroform. Removing one of the ortho-methyl groups somewhat decreased the fluorescence efficiency but greatly improved the photostability. By using a phenyl substituent instead of a methyl group, more stabilization under photoirradiation could be achieved.
Introduction
Recently, stable luminescent radicals have attracted much attention for application in highly efficient OLEDs1 utilizing their emitting D1 (doublet lowest excited state) to D0 (doublet ground state) transition. They are also actively studied as emitting materials closely related to electron spin2 and magnetism.3
Leaving aside the new types of luminescent radicals that are on the rise,4 polyhalogenated triarylmethyl radicals have established a high degree of stability and a variety of derivative types.5 The most basic stable luminescent triarylmethyl radicals are triphenylmethyl radicals such as tris(2,4,6-trichlorophenyl)methyl radical (TTM, Scheme 1)6 and perchlorotriphenylmethyl radical (PTM),7 and the second group that is similarly easy to obtain and handle is diphenylpyridylmethyl radicals such as (3,5-dichloro-4-pyridyl)bis(2,4,6-trichlorophenyl)methyl radical (PyBTM)8 and (3,5-difluoro-4-pyridyl)bis(2,4,6-trichlorophenyl)methyl radical (F2PyBTM).9 PyBTM and F2PyBTM are superior to TTM in terms of their stability under light irradition8,9 and unique in their ability to form metal complexes.10 However, diphenylpyridyl radicals had been less explored than triphenylmethyl radicals for making highly fluorescent derivatives in organic solvent11 until new reporting, in 2022, on an F2PyBTM NHC-Gold(I) complex12 with 36% photoluminescence quantum yield (PLQY) in dichloromethane and para-mesitylated F2PyBTM (Mes2F2PyBTM)13 with 69% chloroform.
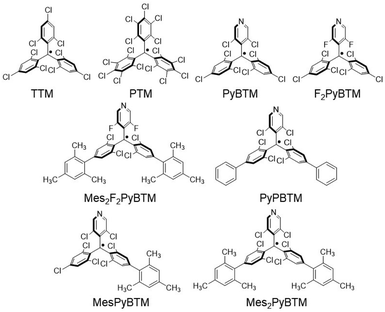 |
| Scheme 1 Structures of TTM and PyBTM derivatives. | |
In addition to the idea of substituting the para-positions to the radical centre of the PyBTM by two phenyl groups (PyPBTM),14ortho-methyl groups on the phenyl groups play an important role in enhancing fluorescence.13 One mesityl group works as an electron-donor in mesitylated PyBTM derivatives and composes a donor–acceptor system with a relatively easily reducible radical centre. The ortho-methyl groups obstruct π-conjugation between the mesityl and diphenylpyridylmethyl in the D0 ground state, and suppress the structural relaxation in the D1 excited state.
Here, we prepared several new Mes2F2PyBTM and Mes2PyBTM analogues with modified hydrocarbon substituents on the terminal phenyl groups. Detailed effects of these substituents on PLQY and photostability were elucidated.
Results and discussion
First, we attempted to synthesize derivatives with bulkier ortho-substituents, expecting higher fluorescence efficiency. Instead of 2,4,6-trimethylphenylboronic acid, we used 2,4,6-triisopropylphenylboronic acid and carried out the Suzuki–Miyaura coupling reaction with a micellar catalysis.14 Despite the bulkiness of the ortho-substituents, the reaction proceeded smoothly, thus producing 2,4,6-triisopropylphenylated αH-PyBTM (precursor of PyBTM) and αH-F2PyBTM (precursor of F2PyBTM). In general, the reaction of αH-PyBTM yields fewer byproducts than that of αH-F2PyBTM. Thanks to the isopropyl groups, we could isolate αH-TippF2PyBTM (3H), although we could not isolate αH-MesF2PyBTM in our previous report. After deprotonation and oxidation, stable luminescent radicals, TippPyBTM (1), Tipp2PyBTM (2), TippF2PyBTM (3), and Tipp2F2PyBTM (4) were obtained (Scheme 2 and Fig. S1 for ESR spectra, ESI†).
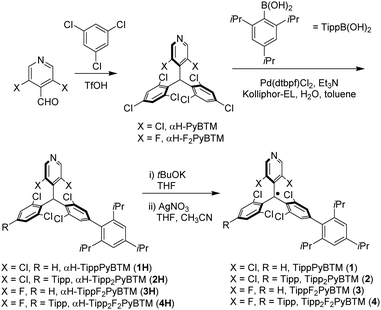 |
| Scheme 2 Synthesis of 1–4. | |
Absorption and emission spectra in dichloromethane of 1, 2, and 4 were very similar to their corresponding mesityl radicals (Fig. 1).13 The lowest energy absorption changes little from PyBTM and F2PyBTM because the triisopropylphenyl groups are poorly conjugated with the radical moieties at the ground state. In order to estimate structures using DFT, we adopted the UB3LYP level of theory with 6-31G(d, p) basis sets, since they closely reproduced the experimental absorption and emission spectra from previous studies. The solvent effect of dichloromethane was considered by using a polarizable continuum model (PCM).15 In the DFT optimized structure of 1, 2, and 4, the triisopropylphenyl groups are nearly perpendicular to the bonded dichorophenyl group, similarly to Mes2F2PyBTM (Table 1, Table S1 (ESI†), Scheme 3, ϕ4 and ϕ5).
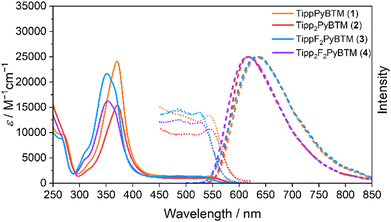 |
| Fig. 1 Absorption (solid line) and emission (broken line, λex = 450 nm) spectra of TippPyBTM (1, orange), Tipp2PyBTM (2, red), TippF2PyBTM (3, light blue), and Tipp2F2PyBTM (4, purple) in dichloromethane. Enlarged portions of the absorption spectra (10 fold) are shown from λ = 450 to 620 nm (dotted lines). | |
Table 1 Calculated torsion angles of the aryl rings of radicals in dichloromethane. Angles outside of brackets are in the D0 state optimized using UB3LYP/6-31G(d, p). Angles inside the brackets are in the D1 state optimized using TD-UB3LYP/6-31G(d,p)
|
1
|
2
|
3
|
4
|
φ
1: Torsion angle of pyridyl ring. φ2 and φ3: torsion angles of dichlorophenyl rings. ϕ4 and ϕ5: dihedral angles between dichlorophenyl groups and 2,4,6-triisopropylphenyl groups. |
φ
1
|
48° [36°] |
48° [37°] |
32° [23°] |
32° [23°] |
φ
2
|
49° [52°] |
49° [52°] |
52° [54°] |
52° [53°] |
φ
3
|
49° [47°] |
49° [48°] |
52° [48°] |
52° [49°] |
ϕ
4
|
90° [55°] |
88° [55°] |
89° [54°] |
89° [54°] |
ϕ
5
|
|
88° [87°] |
|
89° [89°] |
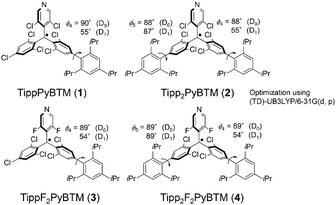 |
| Scheme 3 Dihedral angles between dichlorophenyl groups and 2,4,6-triisopropylphenyl groups. | |
On the other hand, the emission maxima of radicals were to some extent redshifted from PyBTM and F2PyBTM because one of the triisopropylphenyl groups is conjugated by a certain amount with the bonded dichorophenyl group. The TD-DFT optimized dihedral angles between the triisopropylphenyl ring and dichlorophenyl ring (ϕ4) were ca. 54–55°, whereas the dihedral angles between the mesityl ring and dichlorophenyl ring were ca. 49–54°. These changes can be explained by the electronic structures, similarly to the cases of mesityl groups. The lowest excitation of these radicals can generally be regarded as the β-spin electron transition from the β-HOMO to the β-LUMO by TD-DFT calculations, where the spin of an unpaired electron of the radical is defined as α-spin. Since the β-HOMOs of 1, 2, 3, and 4 are mainly distributed on one of the triisopropylphenyl groups (Fig. 2), these triisopropylphenyl groups become cationic at the lowest excited state (D1) by intramolecular electron transfer. The cationic triisopropylphenyl ring seeks conjugation between the bonded dichlorophenyl ring and stabilizes its energy by delocalizing the plus charge.
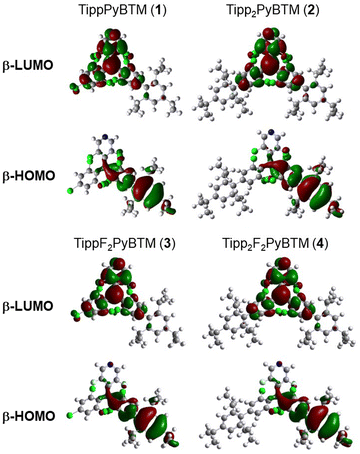 |
| Fig. 2 TD-DFT calculated frontier orbitals of 1, 2, 3, and 4 at the D1 optimized structure calculated using UB3LYP/6-31G(d,p). | |
The PLQYs (Φf) of 1, 2, and 4 were inferior to those of the corresponding mesityl radicals in both dichloromethane and chloroform (Table 2 and Table S2, ESI†), while 4 in particular was still highly emissive. The PLQYs of the radicals are simply determined by the competition of the rate of fluorescence (kf) and the rate of the non-radiative decay from the D1 to D0. These parameters can be calculated from measured PLQYs and fluorescence lifetimes. The kf values of TippPyBTM (1), Tipp2PyBTM (2), and Tipp2F2PyBTM (4) were lower than those of MesPyBTM, Mes2PyBTM, and Mes2F2PyBTM, respectively. This effect can be explained by the lower conjugation between the triisopropylphenyl and dichlorophenyl groups, which probably delayed the transition from D1 to D0. Actually, the TD-DFT calculated oscillator strengths of the D0-D1 transitions at the D1 optimized structures of 1, 2, and 4 were slightly smaller than those of the corresponding mesityl radicals. The knr values show that the isopropyl groups also do not contribute to decreasing the internal conversion caused by vibronic coupling, and they were rather counterproductive in 1 and 2.
Table 2 PLQYs and photophysical parameters of radicals in dichloromethane
|
λ
em (nm) |
Φ
f (%) |
τ/ns |
k
f/107 s−1 |
k
nr/107 s−1 |
Photophysical parameters of MesPyBTM, Mes2PyBTM, and Mes2F2PyBTM are cited from ref. 13. All Φf values were obtained by absolute PLQY measurement. |
MesPyBTM |
645 |
30 |
26 |
1.2 |
2.7 |
1
|
637 |
22 |
22 |
1.0 |
3.5 |
Mes2PyBTM |
628 |
47 |
38 |
1.2 |
1.4 |
2
|
618 |
39 |
37 |
1.1 |
1.6 |
3
|
632 |
46 |
32 |
1.4 |
1.7 |
Mes2F2PyBTM |
623 |
66 |
44 |
1.5 |
0.8 |
4
|
617 |
62 |
47 |
1.3 |
0.8 |
Previously, it was reported that aryl substitution of PyBTM further improved the photostability of the radicals.14 However, the photostabilities of MesPyBTM, Mes2PyBTM, and Mes2F2PyBTM were very near to that of PyBTM. Perpendicular mesityl groups do not help to improve photostability. Similarly, 1, 2, 3 and 4 were not more photostable than PyBTM (Fig. S2a and Table S3, ESI†), although they are still much more photostable than TTM.8 Perpendicular triisopropylphenyl groups also do not help to improve photostability.
In order to clarify the role of m-positions on the phenyl groups, we next prepared F2PyBTM derivatives with 2,3,4,5,6-pentamethylphenyl groups. Ph*F2PyBTM (5) and Ph*2F2PyBTM (6) were synthesized using 2,3,4,5,6-pentamethylphenylboronic acid (Scheme 4). The steric effect of the ortho-methyl groups was not expected to change, but the inductive effect of m-methyl groups became a matter of interest.
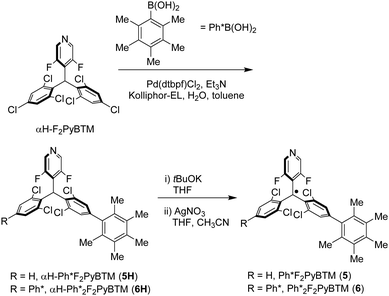 |
| Scheme 4 Synthesis of 5 and 6. | |
Absorption spectra of these radicals were very similar to that of Mes2F2PyBTM (Fig. 3). The pentamethylphenyl group was not conjugated with the radical, similarly to the mesityl and triisopropylphenyl groups. The DFT optimized dihedral angles between the pentamethyl group and the dichlorophenyl group were nearly 90° (Table 3 and Scheme 5). Surprisingly, the fluorescence of 5 and 6 was quite weak (<2%) in dichloromethane. The TD-DFT calculation shows that their first excited state D1 is almost completely composed of the excitation of the β-spin electron, which is similar to other PyBTM derivatives. The main transition, the β-HOMO–LUMO transition, can be regarded as intramolecular electron transfer from β-HOMO on the added phenyl group to the β-LUMO on the radical moiety. The β-HOMOs were to some extent delocalized on the dichlorophenyl group in the mesityl and triisopropylphenyl radicals; however, the β-HOMOs of pentamethylphenyl radicals are almost localized on the pentamethyl phenyl groups (Fig. 4), and the dihedral angles between the pentaphenyl and dichlorophenyl groups are still nearly 90° at the D1 state (Table 3). This means that plus and minus charges at the D1 state of 5 and 6 are more separated. The luminescence of such radicals tends to be quenched in polar solvents.11,16
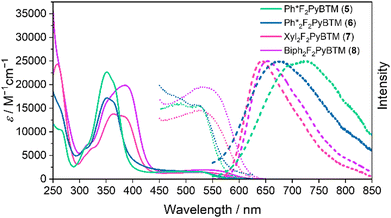 |
| Fig. 3 Absorption (solid line) and emission (broken line, λex = 450 nm) spectra of Ph*F2PyBTM (5, pale green), Ph*2F2PyBTM (6, dark green), Xyl2F2PyBTM (7, pink), and Biph2F2PyBTM (8, pale purple) in dichloromethane. Enlarged portions of the absorption spectra (10 fold) are shown from λ = 450 to 650 nm (dotted lines). | |
Table 3 Calculated torsion angles of the aryl rings of radicals in dichloromethane. Angles outside of brackets are in the D0 state optimized using UB3LYP/6-31G(d, p). Angles inside the brackets are in the D1 state optimized using TD-UB3LYP/6-31G(d,p)
|
5
|
6
|
7
|
8
|
φ
1: Torsion angle of pyridyl ring. φ2 and φ3: torsion angles of dichlorophenyl rings. ϕ4 and ϕ5: dihedral angles between dichlorophenyl groups and (alkyl)phenyl groups. ϕ6 and ϕ7: dihedral angles between o-phenylene groups and phenyl groups. |
φ
1
|
32° [25°] |
32° [24°] |
32° [27°] |
32° [25°] |
φ
2
|
52° [50°] |
52° [52°] |
52° [52°] |
52° [50°] |
φ
3
|
53° [51°] |
52° [49°] |
52° [46°] |
52° [48°] |
ϕ
4
|
88° [89°] |
88° [88°] |
48° [34°] |
48° [39°] |
ϕ
5
|
|
88° [89°] |
51° [50°] |
50° [49°] |
ϕ
6
|
|
|
|
52° [39°] |
ϕ
7
|
|
|
|
52° [51°] |
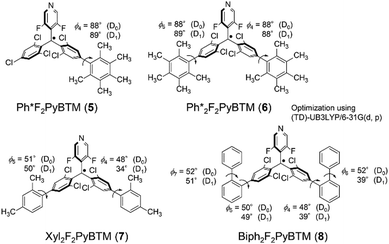 |
| Scheme 5 Dihedral angles between benzene rings. | |
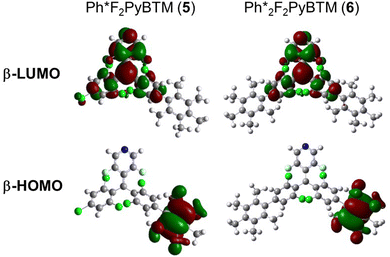 |
| Fig. 4 TD-DFT calculated frontier orbitals of 5 and 6 at the D1 optimized structure calculated using UB3LYP/6-31G(d,p). | |
The table of photophysical parameters of Ph*F2PyBTM (5) and Ph*2F2PyBTM (6) show that they have quite large non-radiative decay rates in dichloromethane in addition to small rates of fluorescence. Luminescence quenching in polar organic solvents have been reported in the donor–acceptor system using TTM as an acceptor. Even chloroform (relative permittivity εr = 4.8) can largely decrease the PLQY of the carbazole-TTM system.11ab In the cases of Ph*F2PyBTM (5) and Ph*2F2PyBTM (6), dichloromethane (εr = 9.1) quenches the fluorescence, while chloroform maintains high-efficiency fluorescence (30% and 47%, respectively, Table S2, ESI†). We propose that the minimum polarity of a solvent that can quench the fluorescence is a measure of the degree of polarization at the intramolecular charge transfer excited state in the D–A radical systems.
So far, we have considered PyPBTM derivatives with alkyl groups at double ortho-positions. What about the effect of an alkyl group at a single ortho-position, and what about the effect of an aryl group at a single ortho-position? Using 2,4,-dimethylphenylboronic acid and 2-biphenylboronic acid, we prepared Xyl2F2PyBTM (7) and Biph2F2PyBTM (8), respectively (Scheme 6).
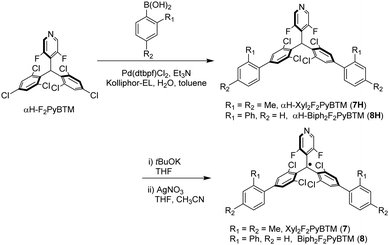 |
| Scheme 6 Synthesis of 7 and 8. | |
Absorption spectra of 7 and 8 show that the lowest energy absorptions of these radicals are slightly redshifted (Fig. 3). This means that the xylyl and biphenyl groups can be conjugated to the radical moieties due to less steric groups at the ortho-positions. The DFT-optimized dihedral angles between the dichlorophenyl ring and xylyl or biphenyl were 48–51° at the ground state (Table 3 and Scheme 5). The emission peaks of these radicals were more greatly bathochromically shifted, since the conjugation between dichlorophenyl and xylyl or biphenyl was strengthened. The TD-DFT-optimized dihedral angles were 34° in 7 and 39° in 8 on one side Fig. 5.
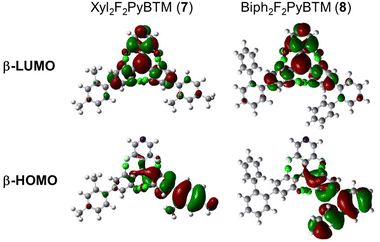 |
| Fig. 5 TD-DFT calculated frontier orbitals of 7 and 8 at the D1 optimized structure calculated using UB3LYP/6-31G(d,p). | |
Xyl2F2PyBTM (7) showed bright fluorescence in solutions, and its PLQY was 40% in dichloromethane. The rate of fluorescence (kf) of 7 was similar to that of Mes2F2PyBTM (Tables 2 and 4). The difference in PLQYs between 7 and Mes2F2PyBTM derives from the difference in the rate of non-radiative decay (knr), which is seen in the shorter fluorescence lifetime (26 ns) of 7 compared to the fluorescence lifetime (44 ns) of Mes2F2PyBTM. It was shown that even a single ortho-methyl group has a considerable effect in suppressing the non-radiative decay, although it did not reach that of double ortho-methyl groups. The PLQY of 15% for 8 was a bit larger than that of PyPBTM, although it showed similar redshifted emission (Table 4). The fluorescence lifetime of 8 was slightly longer than those of F2PyBTM and PyPBTM, and the rate of fluorescence of 8 was faster than that of PyPBTM.
Table 4 PLQYs and photophysical parameters of radicals in dichloromethane
|
λ
em (nm) |
Φ
f (%) |
τ/ns |
k
f/107 s−1 |
k
nr/107 s−1 |
Cited from ref. 8.
Cited from ref. 9.
Cited from ref. 13. All Φf values were obtained by absolute PLQY measurement.
|
PyBTMa |
585 |
2 |
6.4 |
0.3 |
14 |
F2PyBTMb |
566 |
4 |
12.5 |
0.3 |
7.7 |
PyPBTMc |
654 |
9.5 |
12 |
0.8 |
7.5 |
5
|
725 |
0.5 |
0.7 |
0.7 |
142 |
6
|
670 |
1.3 |
2.4 |
0.5 |
41 |
7
|
642 |
40 |
26 |
1.5 |
2.3 |
8
|
653 |
15 |
15 |
1.0 |
5.7 |
The photostabilities of Ph*F2PyBTM (5) and Ph*2F2PyBTM (6) in chloroform were clearly improved over that of PyBTM (Fig. S2b and Table S4, ESI†). We speculate that photostability almost entirely depends on the stability of the intramolecular charge transfer excited state. The two additional methyl groups of the pentamethylphenyl group compared to the mesityl group are thought to stabilize the positive charge on the terminal phenyl groups through electronic or steric effects.
Xyl2F2PyBTM (7) had larger photostability than F2PyBTM and Mes2F2PyBTM in dichloromethane (Fig. 6). The lack of one ortho-methyl group can be interpreted as having less protection by methyl groups, but the conjugation between the dichlorophenyl and xylyl groups had a larger stabilization effect. Photostabilization by conjugation between benzene rings is more pronounced in 8. Conjugation of the phenylene ring with both dichlorophenyl and terminal ortho-phenyl rings (Fig. 5) resulted in stabilization of about 100 times that of PyBTM under 370 nm UV irradiation (Table S3, ESI†). These effects are consistent with the previous reports of photostabilization of TTM using carbazole donors and phenyl rings.17
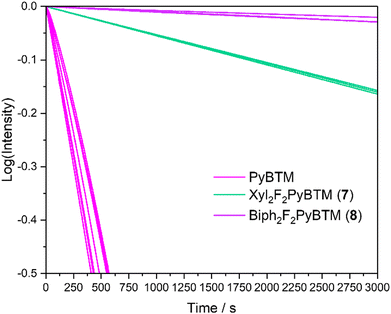 |
| Fig. 6 Plots showing the emission decay of 7 and 8 in dichloromethane under continuous excitation with light at λex = 370 nm ± 10 nm. | |
Conclusions
We synthesized eight novel photostable radicals and clarified the role of hydrocarbon substituents on the terminal phenyl groups. Isopropy groups at the 2-, (4-,) and 6-positions had the effect of sterically disturbing the conjugation between the dichlorophenyl group and the terminal phenyl group, which had a positive role in the enhancement of fluorescence in the previous case of methyl groups. However, an excessive steric effect was found to slightly detract from the PLQYs. Methyl groups at the 2-, 3-, 4-, 5-, and 6-positions had the effect of strengthening the electron-donor character of the phenyl group. The charge-separated excited state was largely quenched in dichloromethane, whereas a smaller amount of quenching occured in less polar chloroform. Single substitution of the ortho-position (2-position) of the terminal phenyl group had certain effects on enhancing the fluorescence compared to no substitution at the ortho-position. The effect of the ortho-methyl group is indicated to be superior to that of the ortho-phenyl group. Compared to the ineffectiveness of the set of 2,4,6-methyl or 2,4,6-triisopropyl groups in the enhancement of photostability, methyl groups at the 3,5-positions or a blank (hydrogen substituent) of the 6-position had a pronounced effect on stabilizing the excited state, thus enhancing the photostability. These findings will be useful in the development of a donor-PyBTM or donor-F2PyBTM system with high PLQY, high photostability, and other useful functions.
Experimental
Materials and methods
The starting materials, αH-PyBTM and αH-F2PyBTM was prepared according to the previous report.8,9 Commercially available compounds were used as received without further purification. The emulsion of toluene in aqueous 2 wt% Kolliphor EL (K-EL) was prepared by mixing a 2 wt% aqueous dispersion of K-EL (1.8 g of K-EL in 88.2 mL of deionized water) with 10 mL of toluene until a stable, milky dispersion was obtained. The emulsion and triethylamine were deoxygenated bubbling argon before use. Preparative recycling gel permeation chromatography was performed with a recycling preparative HPLC, LaboACE LC-5060, Japan Analytical Industry Co., Ltd. 1H (400 MHz) and 13C NMR (100 MHz) spectra were recorded on a JEOL JNM-ECS 400 spectrometer using CDCl3. The residual solvent signals (1H NMR: δ 7.26, 13C NMR: δ 77.16) were used as the internal standards. Gaussian 16 software was used for the DFT and TD-DFT calculations. ESR spectra were recorded with a JEOL JES-FR30EX spectrometer with X-band microwave. Sample solutions were charged in a 2.5 mmϕ sample tube. Magnetic field was calibrated with the Mn2+/MgO standard. Mass spectrometry was performed with a JEOL-JMS-S3000 (MALDI-Spiral-TOF MS) mass spectrometer with DCTB (20 mg/mL in CHCl3) as a matrix and TFANa (1 mg mL−1 in THF) as a cationization agent. Absorption and emission spectra were monitored on Hitachi U-4150 spectrophotometer and Hitachi F-7100 fluorescence spectrophotometer, respectively. Photostability under 370 nm light were recorded with a JASCO FP-8600KS spectrofluorometer. Absolute luminescence quantum yields were measured using Hamamatsu Photonics Quantaurus QY. Photoluminescence decay curves were measured using a measurement system with a picosecond diode laser with the emission wavelength of 375 nm (Advanced Laser Diode Systems PIL037X) as light source, a single grating spectrometer (Andor Kymera193i-B1), and a photon counting detector (MPD SPD-050-CTE) operated with a time-correlated single photon counting (TCSPC) technique. Elemental analysis was conducted at NAIST (1, 2, 3, 5, 8) and Center for Organic Elemental Microanalysis, Graduate School of Pharmaceutical Sciences, Kyoto University (4, 6, 7).
Synthesis of αH-TippPyBTM (1H) and αH-Tipp2PyBTM (2H)
In a Schlenk tube αH-PyBTM (521 mg, 1.00 mmol), 2,4,6-triisopropylphenylboronic acid (744 mg, 3.00 mmol), Pd(dtbpf)Cl2 (65.2 mg, 0.100 mmol), were put under an argon atmosphere. Degassed K-EL 2 wt%: water
:
toluene (9
:
1 v/v) emulsion (3.5 mL) was added, and the mixture was heated at 70 °C. Degassed triethylamine (1.2 mL, 8.6 mmol) was finally added, and the reaction mixture was stirred at 70 °C overnight. The reaction mixture was cooled down to room temperature, added chloroform, and filtered on a pad of silica and sodium sulfate. The solvent was evaporated and purified by silica gel column chromatography (CHCl3
:
hexane = 1
:
1). The yellow oil (806 mg) was separated by GPC (CHCl3) to obtain pure 1H (240 mg, 0.348 mmol, 35%) and 2H (113 mg, 0.132 mmol, 13%).
1H (1
:
1 mixture of two conformers).
1H NMR (400 MHz, CDCl3, ppm): δ 8.52 (s, 0.5H), 8.50 (s, 0.5H), 8.39 (s, 0.5H), 8.38 (s, 0.5H), 7.44 (d, J = 2.1 Hz, 0.5H), 7.40 (d, J = 2.2 Hz, 0.5H), 7.31 (d, J = 2.2 Hz, 0.5H), 7.26 (br, 0.5H), 7.22 (d, J = 1.5 Hz, 0.5H), 7.21 (d, J = 1.6 Hz, 0.5H), 7.10 (d, J = 1.5 Hz, 0.5H), 7.07 (d, J = 1.6 Hz, 0.5H), 7.05 (s, 2H), 6.83 (s, 0.5H), 6.83 (s, 0.5H), 2.93 (sep, J = 6.9 Hz, 1H), 2.66–2.54 (m, 2H), 1.29 (d, J = 6.9, Hz, 6H), 1.15–1.04 (m, 12H).
13C NMR (100 MHz, CDCl3, ppm): δ 149.7, 149.5, 149.1, 147.9, 147.8, 146.3, 146.2, 144.2, 144.1, 142.9, 142.8, 138.1, 138.0, 137.4, 137.4, 137.0, 137.0, 136.4, 134.6, 134.5, 134.2, 134.1, 133.9, 133.7, 133.7, 133.6, 133.3, 132.1, 131.9, 131.8, 131.6, 130.5, 130.1, 130.0, 129.9, 128.8, 128.5, 120.9, 120.8, 50.0, 34.5, 30.6, 30.5, 24.3, 24.3, 24.2, 24.0.
HRMS (MALDI-TOF MS positive mode) m/z: [MH]+ calcd for C33H31Cl7N+ 686.02707; found 686.02764.
2H
.
1H NMR (400 MHz, CDCl3, ppm): δ 8.53 (s, 1H), 8.40 (s, 1H), 7.27 (d, J = 1.7 Hz, 1H), 7.23 (d, J = 1.7 Hz, 1H), 7.13 (d, J = 1.7 Hz, 1H), 7.08 (d, J = 1.7 Hz, 1H), 7.06 (br, 4H), 6.95 (s, 1H), 2.94 (sep, J = 6.9 Hz, 2H), 2.72–2.57 (m, 4H), 1.30 (dd, J = 6.9, 1.4 Hz, 12H), 1.16–1.07 (m, 24H).
13C NMR (100 MHz, CDCl3, ppm): δ 149.6, 149.1, 147.9, 146.5, 146.3, 146.3, 144.9, 142.6, 137.1, 137.0, 136.7, 136.3, 134.7, 134.1, 134.0, 133.9, 132.8, 132.2, 132.0, 131.7, 130.0, 130.0, 120.9, 50.3, 30.6, 30.6, 30.5, 30.4, 24.5, 24.3, 24.3, 24.3, 24.3, 24.1, 24.1.
HRMS (MALDI-TOF MS positive mode) m/z: [MH]+ calcd for C48H54Cl6N+ 854.23819; found 854.23855.
Synthesis of αH-TippF2PyBTM (3H) and αH-Tipp2F2PyBTM (4H)
In a Schlenk tube αH-F2PyBTM (489 mg, 1.00 mmol), 2,4,6-triisopropylphenylboronic acid (746 mg, 3.01 mmol), Pd(dtbpf)Cl2 (52.3 mg, 0.0802 mmol), were put under an argon atmosphere. Degassed K-EL 2 wt%: water
:
toluene (9
:
1 v/v) emulsion (3.5 mL) was added, and the mixture was heated at 80 °C. Degassed triethylamine (0.85 mL, 6.1 mmol) was finally added, and the reaction mixture was stirred at 80 °C overnight. The reaction mixture was cooled down to room temperature, added dichloromethane, and filtered on a Celite pad. The solvent was evaporated and purified by silica gel column chromatography (CHCl3
:
hexane = 1
:
1). The mixture was separated by GPC (CHCl3) to obtain crude 3H (291 mg, 44%) and pure 4H (128 mg, 0.195 mmol, 20%). Recrystallization of crude 3H from dichloromethane-methanol twice gave pure 3H (69.8 mg, 0.106 mmol, 11%).
3H
.
1H NMR (400 MHz, CDCl3, ppm): δ 8.36 (s, 1H), 8.24 (s, 1H), 7.36 (s, 2H), 7.15 (s, 2H), 7.04 (s, 2H), 6.76 (s, 1H), 2.92 (sep, J = 6.9 Hz, 1H), 2.57 (sep, J = 6.8 Hz, 4H), 1.28 (d, J = 6.9 Hz, 6H), 1.11 (d, J = 6.8 Hz, 6H), 1.10 (d, J = 6.8 Hz, 6H).
13C NMR (100 MHz, CDCl3, ppm): δ 149.1, 146.3, 142.8, 137.2, 136.1, 135.4, 134.2, 133.8, 133.0, 131.4, 131.0, 129.6, 120.8, 42.0, 34.5, 30.5, 24.3, 24.2, 24.1.
HRMS(MALDI-TOF MS positive mode) m/z: [MH]+ calcd for C33H31Cl5NF2+ 654.08617; found 654.08662.
4H
.
1H NMR (400 MHz, CDCl3, ppm): δ 8.38 (s, 1H), 8.25 (s, 1H), 7.17 (s, 4H), 7.05 (s, 4H), 6.88 (s, 1H), 2.93 (sep, J = 6.9 Hz, 2H), 2.62 (sep, J = 6.8 Hz, 4H), 1.30 (d, J = 6.9 Hz, 12H), 1.13 (d, J = 6.8 Hz, 12H), 1.12 (d, J = 6.8 Hz, 12H).
13C NMR (100 MHz, CDCl3, ppm): δ 149.1, 146.4, 142.5, 136.1, 135.3, 135.1, 134.0, 133.8, 133.6, 132.1, 131.0, 125.5, 125.4, 125.2, 120.9, 42.4, 42.3, 34.5, 30.5, 24.4, 24.3, 24.2.
HRMS (MALDI-TOF MS positive mode) m/z: [MH]+ calcd for C48H54Cl4NF2+ 822.29729; found 822.29764.
Synthesis of αH-Ph*PyBTM (5H) and αH-Ph*2F2PyBTM (6H)
In a Schlenk tube αH-F2PyBTM (330 mg, 0.675 mmol), 2,3,4,5,6-pentamethylphenylboronic acid (414 mg, 2.16 mmol), Pd(dtbpf)Cl2 (43 mg, 0.066 mmol), were put under an argon atmosphere. Degassed K-EL 2 wt%: water
:
toluene (9
:
1 v/v) emulsion (2.4 mL) was added, and the mixture was heated at 70 °C. Degassed triethylamine (0.56 mL, 4.0 mmol) was finally added, and the reaction mixture was stirred at 70 °C overnight. The reaction mixture was cooled down to room temperature, added chloroform, and filtered on a Celite pad. The solvent was evaporated and purified by silica gel column chromatography (CHCl3
:
hexane = 1
:
1). The mixture (538 mg) was separated by GPC (CHCl3) to obtain crude 5H (224 mg, 55%) and 6H (91 mg, 19%). Recrystallization from dichloromethane-methanol gave 5H (142 mg, 24%) and 6H (35 mg, 0.049 mmol, 7.3%).
5H
.
1H NMR (400 MHz, CDCl3, ppm): δ 8.35 (s, 1H), 8.23 (s, 1H), 7.35 (s, 2H), 7.08 (s, 2H), 6.74 (s, 1H), 2.29 (s, 3H), 2.24 (s, 6H), 1.95 (s, 6H).
13C NMR (100 MHz, CDCl3, ppm): δ 145.0, 137.2, 136.6, 136.5, 135.4, 135.1, 134.1, 133.6, 132.8, 131.3, 131.3, 130.8, 129.5, 124.8, 124.7, 124.6, 42.1, 42.0, 18.5, 17.0, 16.7.
HRMS (MALDI-TOF MS positive mode) m/z: [MH]+ calcd for C29H23Cl5NF2+ 598.02357; found 598.02323.
6H
.
1H NMR (400 MHz, CDCl3, ppm): δ 8.37 (s, 1H), 8.25 (s, 1H), 7.10 (s, 4H), 6.86 (s, 1H), 2.29 (s, 6H), 2.25 (s, 12H), 1.97 (s, 12H).
13C NMR (100 MHz, CDCl3, ppm): δ 144.7, 136.7, 136.5, 135.4, 135.3, 135.1, 133.7, 133.5, 132.8, 132.0, 131.4, 131.0, 130.7, 125.2, 42.3, 18.6, 17.0, 16.7.
HRMS(MALDI-TOF MS positive mode) m/z: [MH]+ calcd for C40H38Cl4NF2+ 710.17209; found 710.17249.
Synthesis of αH-Xyl2F2PyBTM (7H)
In a Schlenk tube αH-F2PyBTM (244 mg, 0.500 mmol), 2,4-dimethylphenylboronic acid (225 mg, 1.50 mmol), Pd(dtbpf)Cl2 (32.6 mg, 0.0500 mmol), were put under an argon atmosphere. Degassed K-EL 2 wt%: water
:
toluene (9
:
1 v/v) emulsion (1.8 mL) was added, and the mixture was heated at 70 °C. Degassed triethylamine (0.45 mL, 3.2 mmol) was finally added, and the reaction mixture was stirred at 70 °C overnight. The reaction mixture was cooled down to room temperature, added chloroform, and filtered on a Celite pad. The solvent was evaporated and purified by silica gel column chromatography (CHCl3
:
hexane = 1
:
1). The mixture was separated by GPC (CHCl3) to obtain crude 7H and monoadduct. (58 mg, ca. 20%). Recrystallization from dichloromethane-methanol gave 7H (25.0 mg, 0.040 mmol, 8.0%).
1H NMR (400 MHz, CDCl3, ppm): δ 8.36 (s, 1H), 8.24 (s, 1H), 7.27 (s, 4H), 7.12 (d, 2H. J = 7.8 Hz), 7.09 (s, 2H), 7.06 (d, 2H. J = 7.8 Hz), 6.84 (s, 1H), 2.36 (s, 6H), 2.26 (s, 6H).
13C NMR (100 MHz, CDCl3, ppm): δ 143.4, 138.2, 136.2, 135.8, 135.1, 132.1, 131.5, 130.3, 129.5, 126.9, 42.2, 21.2, 20.4.
HRMS (MALDI-TOF MS positive mode) m/z: [MH]+ calcd for C34H26Cl4NF2+ 626.07819; found 626.07819.
Synthesis of αH-Biph2F2PyBTM (8H)
In a Schlenk tube αH-F2PyBTM (245 mg, 0.502 mmol), 2-biphenylboronic acid (396 mg, 2.00 mmol), Pd(dtbpf)Cl2 (33 mg, 0.051 mmol), were put under an argon atmosphere. Degassed K-EL 2 wt%: water
:
toluene (9
:
1 v/v) emulsion (1.8 mL) was added, and the mixture was heated at 70 °C. Degassed triethylamine (0.42 mL, 3.0 mmol) was finally added, and the reaction mixture was stirred at 70 °C overnight. The reaction mixture was cooled down to room temperature, added chloroform, and filtered on a Celite pad. The solvent was evaporated and purified by silica gel column chromatography (CHCl3
:
hexane = 1
:
1). The mixture was separated by GPC (CHCl3) to obtain crude 8H (195 mg, ca. 54%). Recrystallization from dichloromethane-methanol three times gave pure 8H (177 mg, 0.245 mmol, 49%).
1H NMR (400 MHz, CDCl3, ppm): δ 8.30 (s, 1H), 8.19 (s, 1H), 7.46–7.43 (m, 8H), 7.25–7.23 (m, 6H), 7.12–7.10 (m, 4H), 7.04 (s, 4H), 6.63 (s, 1H).
13C NMR (100 MHz, CDCl3, ppm): δ 142.9, 140.9, 140.4, 137.4, 136.0, 135.2, 134.9, 133.6, 133.4, 131.8, 130.8, 130.7, 130.0, 128.7, 128.2, 127.8, 127.2, 125.0, 42.0.
HRMS (MALDI-TOF MS positive mode) m/z: [MH]+ calcd for C42H26Cl4NF2+ 722.07819; found 722.07761.
Synthesis of TippPyBTM (1)
Under an argon atmosphere, αH-TippPyBTM (1H, 108.1 mg, 0.157 mmol) was dissolved in dry THF (∼3 mL), and tBuOK in THF (1 M solution, 0.3 mL, 1.9 eq.) was added. The reaction mixture was stirred overnight in the dark. Silver nitrate (87.5 mg, 0.515 mmol) in acetonitrile (1.6 mL) was added and stirred for 2.5 h. The reaction mixture was added chloroform, filtered on a Celite pad, evaporated and purified by flash chromatography on silica gel (CHCl3
:
hexane = 1
:
1) and dried in vacuo to afford 1 (96.6 mg, 0.140 mmol, 89%) as a red solid.
Elemental analysis calcd for C33H29Cl7N: C 57.63, H 4.25, N 2.04, found: C 57.98, H 4.18, N 2.11.
HRMS (MALDI-TOF MS negative mode) m/z: [M]− calcd for C33H29Cl7N− 684.01142; found 684.01141.
Synthesis of Tipp2PyBTM (2)
Under an argon atmosphere, αH-Tipp2PyBTM (2H, 60.0 mg, 0.0700 mmol) was dissolved in dry THF (3 mL), and tBuOK in THF (1 M solution, 0.15 mL, 2.1 eq.) was added. The reaction mixture was stirred overnight in the dark. Silver nitrate (40.2 mg, 0.237 mmol) in acetonitrile (∼0.7 mL) was added and stirred for 2.25 h. The reaction mixture was filtered on a Celite pad, and the solvent was evaporated. The crude product was purified by silica gel column chromatography (CHCl3
:
hexane = 1
:
1) and dried in vacuo to afford 2 (53.7 mg, 0.0628 mmol, 90%) as a red solid.
Elemental analysis calcd for C48H52Cl6N: C 67.38, H 6.13, N 1.64, found: C 67.61, H 6.12, N 1.51.
HRMS (MALDI-TOF MS negative mode) m/z: [M]− calcd for C48H52Cl6N− 852.22364; found 852.22402.
Synthesis of TippF2PyBTM (3)
Under an argon atmosphere, αH-TippPyBTM (3H, 44 mg, 0.067 mmol) was dissolved in dry THF (2 mL), and tBuOK in THF (1 M solution, 0.10 mL, 1.5 eq.) was added. The reaction mixture was stirred overnight in the dark. Silver nitrate (29.9 mg, 0.176 mmol) in acetonitrile (0.6 mL) was added and stirred for 2.2 h. The reaction mixture was filtered on a Celite pad, and the solvent was evaporated. The crude product was purified by silica gel column chromatography (CHCl3
:
hexane = 1
:
1) and dried in vacuo to afford 3 (44 mg, 0.067 mmol, quant.) as a red solid.
Elemental analysis calcd for C33H29Cl5F2N: C 60.53, H 4.46, N 2.14, found: C 60.64, H 4.36, N 2.00.
HRMS (MALDI-TOF MS negative mode) m/z: [M]− calcd for C33H29Cl5NF2− 652.07162; found 652.07115.
Synthesis of Tipp2F2PyBTM (4)
Under an argon atmosphere, αH-Tipp2F2PyBTM (4H, 49.2 mg, 0.0597 mmol) was dissolved in dry THF (3 mL), and tBuOK in THF (1 M solution, 0.12 mL, 2.0 eq.) was added. The reaction mixture was stirred overnight in the dark. Silver nitrate (49.6 mg, 0.292 mmol) in acetonitrile (0.8 mL) was added and stirred for 2.25 h. The mixture was added chloroform and filtered on a Celite pad. The solvent was evaporated and the reaction mixture was purified by silica gel column chromatography (CHCl3
:
hexane = 1
:
2) and dried in vacuo to afford 4 (46.0 mg, 0.0559 mmol, 93%) as a red solid.
Elemental analysis calcd for C48H52Cl4F2N: C 70.07, H 6.37, N 1.70, found: C 70.30, H 6.55, N 1.64.
HRMS (MALDI-TOF MS negative mode) m/z: [M]− calcd for C48H52Cl4NF2− 820.28274; found 820.28223.
Synthesis of Ph*F2PyBTM (5)
Under an argon atmosphere, αH-Ph*F2PyBTM (5H, 46.2 mg, 0.0770 mmol) was dissolved in dry THF (3 mL), and tBuOK in THF (1 M solution, 0.1 mL, 1.3 eq.) was added. The reaction mixture was stirred overnight in the dark. Silver nitrate (40.7 mg, 0.240 mmol) in acetonitrile (0.6 mL) was added and stirred for 2.5 h. The mixture was added chloroform and filtered on a Celite pad. The solvent was evaporated and the reaction mixture was purified by silica gel column chromatography (CHCl3
:
hexane = 1
:
1) and dried in vacuo to afford 5 (38.8 mg, 0.0648 mmol, 84%) as a red solid.
Elemental analysis calcd for C29H21Cl5F2N: C 58.18, H 3.54, N 2.34, found: C 58.03, H 3.25, N 2.30.
HRMS (MALDI-TOF MS negative mode) m/z: [M]− calcd for C29H21Cl5NF2− 596.00902; found 596.00916.
Synthesis of Ph*2F2PyBTM (6)
Under an argon atmosphere, αH-Ph*2F2PyBTM (6H, 24.3 mg, 0.0342 mmol) was dissolved in dry THF (3 mL), and tBuOK in THF (1 M solution, 0.1 mL, 2.9 eq.) was added. The reaction mixture was stirred overnight in the dark. Silver nitrate (34.8 mg, 0.205 mmol) in acetonitrile (0.5 mL) was added and stirred for 2.5 h. The mixture was added chloroform and filtered on a Celite pad. The solvent was evaporated and the reaction mixture was purified by silica gel column chromatography (CHCl3
:
hexane = 1
:
1) and dried in vacuo to afford 4 (20.5 mg, 0.0289 mmol, 84%) as a red solid.
Elemental analysis calcd for C40H36Cl4F2N: C 67.62, H 5.11, N 1.97, found: C 67.25, H 5.25, N 2.00.
HRMS (MALDI-TOF MS negative mode) m/z: [M]− calcd for C40H36Cl4NF2− 708.15754; found 708.15708.
Synthesis of Xyl2F2PyBTM (7)
Under an argon atmosphere, αH-Xyl2F2PyBTM (7H, 20.9 mg, 0.0333 mmol) was dissolved in dry THF (3 mL), and tBuOK in THF (1 M solution, 0.1 mL, 3.0 eq.) was added. The reaction mixture was stirred overnight in the dark. Silver nitrate (39.9 mg, 0.234 mmol) in acetonitrile (0.6 mL) was added and stirred for 2.5 h. The mixture was added chloroform and filtered on a Celite pad. The solvent was evaporated and the reaction mixture was purified by silica gel column chromatography (CHCl3
:
hexane = 1
:
1) and dried in vacuo to afford 5 (20 mg, 0.032 mmol, 96%) as a red solid.
Elemental analysis calcd for C34H24Cl4F2N + 0.2CHCl3: C 63.17, H 3.75, N 2.15, found: C 63.03, H 3.86, N 2.13.
HRMS (MALDI-TOF MS negative mode) m/z: [M]− calcd for C34H24Cl4NF2− 624.06364; found 624.06312.
Synthesis of Biph2F2PyBTM (8)
Under an argon atmosphere, αH-BiPh2F2PyBTM (8H, 72.4 mg, 0.100 mmol) was dissolved in dry THF (3 mL), and tBuOK in THF (1 M solution, 0.2 mL, 2.0 eq.) was added. The reaction mixture was stirred overnight in the dark. Silver nitrate (61.8 mg, 0.364 mmol) in acetonitrile (0.9 mL) was added and stirred for 2.5 h. The mixture was added chloroform and filtered on a Celite pad. The solvent was evaporated and the reaction mixture was purified by silica gel column chromatography (CHCl3
:
hexane = 1
:
1) and dried in vacuo to afford 6 (69.6 mg, 0.0963 mmol, 96%) as a red solid.
Elemental analysis calcd for C42H24Cl4F2N: C 69.83, H 3.35, N 1.94, found: C 69.71, H 3.30, N 1.81.
HRMS (MALDI-TOF MS negative mode) m/z: [M]− calcd for C42H24Cl4NF2− 720.06364; found 720.06349.
Author contributions
Y. H. conceived the project. Y. H., R. K., A. B. and K. Y. prepared the compounds. Y. H. conducted the DFT calculations. Y. H. and R. M. did the photophysical measurements. Y. H. wrote original draft, and R. M., T. K., and K. U. reviewed and edited.
Conflicts of interest
There are no conflicts to declare.
Acknowledgements
We thank Prof. Takehiro Kawauchi, Ryukoku University for absolute luminescence quantum yield measurements. We acknowledge support by Ms Yoshiko Nishikawa and Ms Mieko Yamagaki for HRMS (MALDI-TOF MS) and Mr Fumio Asanoma for elemental analysis conducted in NAIST. This work was partly supported by the ARIM Program of the Ministry of Education, Culture, Sports, Science and Technology (MEXT), Japan (JPMXP1222NR0016, JPMXP1223NR0017 and JPMXP1222MS0011). This research was supported by 2022 Ryukoku University Science and Technology Fund, JSPS KAKENHI Grant Number JP20H02759 in Scientific Research (B), JP20K15304 in Early-Career-Scientists and Japan Science and Technology Agency, CREST program Grant Number JPMJCR17N2.
Notes and references
-
(a) Q. Peng, A. Obolda, M. Zhang and F. Li, Organic Light-Emitting Diodes Using a Neutral π Radical as Emitter: The Emission from a Doublet, Angew. Chem., Int. Ed., 2015, 54, 7091–7095 CrossRef CAS PubMed;
(b) X. Ai, E. W. Evans, S. Dong, A. J. Gillett, H. Guo, Y. Chen, T. J. H. Hele, R. H. Friend and F. Li, Efficient radical-based light-emitting diodes with doublet emission, Nature, 2018, 563, 536–540 CrossRef CAS PubMed;
(c) H. Guo, Q. Peng, X.-K. Chen, Q. Gu, S. Dong, E. W. Evans, A. J. Gillett, X. Ai, M. Zhang, D. Credgington, V. Coropceanu, R. H. Friend, J.-L. Brédas and F. Li, High stability and luminescence efficiency in donor–acceptor neutral radicals not following the Aufbau principle, Nat. Mater., 2019, 18, 977–984 CrossRef CAS PubMed;
(d) A. Abdurahman, T. J. H. Hele, Q. Gu, J. Zhang, Q. Peng, M. Zhang, R. H. Friend, F. Li and E. W. Evans, Understanding the luminescent nature of organic radicals for efficient doublet emitters and pure-red light-emitting diodes, Nat. Mater., 2020, 19, 1224–1229 CrossRef CAS PubMed;
(e) F. Li, A. J. Gillett, Q. Gu, J. Ding, Z. Chen, T. J. H. Hele, W. K. Myers, R. H. Friend and E. W. Evans, Singlet and triplet to doublet energy transfer: improving organic light-emitting diodes with radicals, Nat. Commun., 2022, 13, 2744 CrossRef CAS PubMed.
-
(a) A. Ghirri, C. Bonizzoni, F. Troiani, N. Buccheri, L. Beverina, A. Cassinese and M. Affronte, Coherently coupling distinct spin ensembles through a high-Tc superconducting resonator, Phys. Rev. A, 2016, 93, 063855 CrossRef;
(b) Y. Hattori, S. Kimura, T. Kusamoto, H. Maeda and H. Nishihara, Cation-responsive turn-on fluorescence and absence of heavy atom effects of pyridyl-substituted triarylmethyl radicals, Chem. Commun., 2018, 54, 615–618 RSC;
(c) C.-H. Liu, E. Hamzehpoor, Y. Sakai-Otsuka, T. Jadhav and D. F. Perepichka, A Pure-Red Doublet Emission with 90% Quantum Yield: Stable, Colorless, Iodinated Triphenylmethane Solid, Angew. Chem., Int. Ed., 2020, 59, 23030–23034 CrossRef CAS PubMed;
(d) Z. Zhou, C. Qiao, J. Yao, Y. Yan and Y. S. Zhao, Exciton funneling amplified photoluminescence anisotropy in organic radical-doped microcrystals, J. Mater. Chem. C, 2022, 10, 2551–2555 RSC.
-
(a) S. Kimura, T. Kusamoto, S. Kimura, K. Kato, Y. Teki and H. Nishihara, Magnetoluminescence in a Photostable, Brightly Luminescent Organic Radical in a Rigid Environment, Angew. Chem., Int. Ed., 2018, 57, 12711–12715 CrossRef CAS PubMed;
(b) K. Kato, S. Kimura, T. Kusamoto, H. Nishihara and Y. Teki, Luminescent Radical-Excimer: Excited-State Dynamics of Luminescent Radicals in Doped Host Crystals, Angew. Chem., Int. Ed., 2019, 58, 2606–2611 CrossRef CAS PubMed;
(c) S. Kimura, S. Kimura, K. Kato, Y. Teki, H. Nishihara and T. Kusamoto, A ground-state-dominated magnetic field effect on the luminescence of stable organic radicals, Chem. Sci., 2021, 12, 2025–2029 RSC;
(d) S. Kimura, R. Matsuoka, S. Kimura, H. Nishihara and T. Kusamoto, Radical-Based Coordination Polymers as a Platform for Magnetoluminescence, J. Am. Chem. Soc., 2021, 143, 5610–5615 CrossRef CAS PubMed;
(e) R. Matsuoka, S. Kimura, T. Miura, T. Ikoma and T. Kusamoto, Single-Molecule Magnetoluminescence from a Spatially Confined Persistent Diradical Emitter, J. Am. Chem. Soc., 2023, 145, 13615–13622 CrossRef CAS PubMed.
-
(a) Y. Beldjoudi, M. A. Nascimento, Y. J. Cho, H. Yu, H. Aziz, D. Tonouchi, K. Eguchi, M. M. Matsushita, K. Awaga, I. Osorio-Roman, C. P. Constantinides and J. M. Rawson, Multifunctional Dithiadiazolyl Radicals: Fluorescence, Electroluminescence, and Photoconducting Behavior in Pyren-1′-yl-dithiadiazolyl, J. Am. Chem. Soc., 2018, 140, 6260–6270 CrossRef CAS PubMed;
(b) M. Ito, S. Shirai, Y. Xie, T. Kushida, N. Ando, H. Soutome, K. J. Fujimoto, T. Yanai, K. Tabata, Y. Miyata, H. Kita and S. Yamaguchi, Fluorescent Organic π-Radicals Stabilized with Boron: Featuring a SOMO–LUMO Electronic Transition, Angew. Chem., Int. Ed., 2022, e202201965 CAS;
(c) X. Li, Y.-L. Wang, C. Chem, Y.-Y. Ren and Y.-F. Han, A platform for blue-luminescent carbon-centered radicals, Nat. Commun., 2022, 13, 5367 CrossRef CAS PubMed.
-
(a) P. Murto and H. Bronstein, Electro-optical π-radicals: design advances, applications and future perspectives, J. Mater. Chem. C, 2022, 10, 7368–7403 RSC;
(b) R. Matsuoka, A. Mizuno, T. Mibu and T. Kusamoto, Luminescence of doublet molecular systems, Coord. Chem. Rev., 2022, 467, 214646 CrossRef.
- O. Armet, J. Veciana, C. Rovira, J. Riera, J. Casteñer, E. Molins, J. Rius, C. Miravitlles, S. Olivella and J. Brichfeus, Inert carbon free radicals. 8. Polychlorotriphenylmethyl radicals: synthesis, structure, and spin-density distribution, J. Phys. Chem., 1987, 91, 5608–5616 CrossRef CAS.
- M. Ballester and G. de la Fuente, Synthesis and isolation of a perchlorotriphenylcarbanion salt, Tetrahedron Lett., 1970, 11, 4509–4510 CrossRef.
- Y. Hattori, T. Kusamoto and H. Nishihara, Luminescence, Stability, and Proton Response of an Open-Shell (3,5-Dichloro-4-pyridyl)bis(2,4,6-trichlorophenyl)methyl Radical, Angew. Chem., Int. Ed., 2014, 53, 11845–11848 CrossRef CAS PubMed.
- Y. Hattori, T. Kusamoto and H. Nishihara, Highly photostable luminescent open-shell (3,5-dihalo-4-pyridyl)bis(2,4,6-trichlorophenyl)methyl radicals: significant effects of halogen atoms on their photophysical and photochemical properties, RSC Adv., 2015, 5, 64802–64805 RSC.
-
(a) Y. Hattori, T. Kusamoto and H. Nishihara, Enhanced Luminescent Properties of an Open-Shell (3,5-Dichloro-4-pyridyl)bis(2,4,6-trichlorophenyl)methyl Radical by Coordination to Gold, Angew. Chem., Int. Ed., 2015, 54, 3731–3734 CrossRef CAS PubMed;
(b) T. Kusamoto, Y. Hattori, A. Tanushi and H. Nishihara, Intramolecular Ferromagnetic Radical–CuII Coupling in a CuII Complex Ligated with Pyridyl-Substituted Triarylmethyl Radicals, Inorg. Chem., 2015, 54, 4186–4188 CrossRef CAS PubMed;
(c) Y. Hattori, T. Kusamoto, T. Sato and H. Nishihara, Synergistic luminescence enhancement of a pyridyl-substituted triarylmethyl radical based on fluorine substitution and coordination to gold, Chem. Commun., 2016, 52, 13393–13396 RSC.
-
(a) V. Gamero, D. Velasco, S. Latorre, F. López-Calahorra, E. Brillas and L. Juliá, [4-(N-Carbazolyl)-2,6-dichlorophenyl]bis(2,4,6-trichlorophenyl)methyl radical an efficient red light-emitting paramagnetic molecule, Tetrahedron Lett., 2006, 47, 2305–2309 CrossRef CAS;
(b) D. Velasco, S. Castellanos, M. López, F. López-Calahorra, E. Brillas and L. Juliá, Red Organic Light-Emitting Radical Adducts of Carbazole and Tris(2,4,6-trichlorotriphenyl)methyl Radical That Exhibit High Thermal Stability and Electrochemical Amphotericity, J. Org. Chem., 2007, 72, 7523–7532 CrossRef CAS PubMed;
(c) S. Castellanos, D. Velasco, F. López-Calahorra, E. Brillas and L. Juliá, Taking Advantage of the Radical Character of Tris(2,4,6-trichlorophenyl)methyl To Synthesize New Paramagnetic Glassy Molecular Materials, J. Org. Chem., 2008, 73, 3759–3767 CrossRef CAS PubMed;
(d) S. Dong, W. Xu, H. Guo, W. Yan, M. Zhang and F. Li, Effects of substituents on luminescent efficiency of stable triaryl methyl radicals, Phys. Chem. Chem. Phys., 2018, 20, 18657–18662 RSC;
(e) A. Heckmann, S. Dümmler, J. Pauli, M. Margraf, J. Köhler, D. Stich, C. Lambert, I. Fischer and U. Resch-Genger, Highly Fluorescent Open-Shell NIR Dyes: The Time-Dependence of Back Electron Transfer in Triarylamine-Perchlorotriphenylmethyl Radicals, J. Phys. Chem. C, 2009, 113, 20958–20966 CrossRef CAS;
(f) Y. Zhao, A. Abdurahman, Y. Zhang, P. Zhang, M. Zhang and F. Li, Highly Efficient Multifunctional Luminescent Radicals, CCS Chem., 2022, 4, 722–731 CrossRef CAS;
(g) R. Xiaotian, W. Ota, T. Sato, M. Furukori, Y. Nakayama, T. Hosokai, E. Hisamura, K. Nakamura, K. Matsuda, K. Nakano, A. P. Monkman and K. Albrecht, Carbazole-Dendronized Luminescent Radicals, Angew. Chem., Int. Ed., 2023, e202302550 CAS.
- Y. Hattori, R. Kitajima, R. Matsuoka, T. Kusamoto, H. Nishihara and K. Uchida, Amplification of luminescence of stable radicals by coordination to NHC–gold(I) complex, Chem. Commun., 2022, 58, 2560–2563 RSC.
- Y. Hattori, R. Kitajima, W. Ota, R. Matsuoka, T. Kusamoto, T. Sato and K. Uchida, The simplest structure of a stable radical showing high fluorescence efficiency in solution: benzene donors with triarylmethyl radicals, Chem. Sci., 2022, 13, 13418–13425 RSC.
- S. Mattiello, F. Corsini, S. Mecca, M. Sassi, R. Ruffo, G. Mattioli, Y. Hattori, T. Kusamoto, G. Griffini and L. Beverina, First demonstration of the use of open-shell derivatives as organic luminophores for transparent luminescent solar concentrators, Mater. Adv., 2021, 2, 7369–7378 RSC.
-
(a) G. Scalmani and M. J. Frisch, Continuous surface charge polarizable continuum models of solvation. I. General formalism, J. Chem. Phys., 2010, 132, 114110 CrossRef PubMed;
(b) R. Improta, V. Barone, G. Scalmani and M. J. Frisch, A state-specific polarizable continuum model time dependent density functional theory method for excited state calculations in solution, J. Chem. Phys., 2006, 125, 054103 CrossRef PubMed.
-
(a) M. López, D. Velasco, F. López-Calahorra and L. Juliá, Light-emitting persistent radicals for efficient sensor devices of solvent polarity, Tetrahedron Lett., 2008, 49, 5196–5199 CrossRef;
(b) L. Fajarí, R. Papoular, M. Reig, E. Brillas, J. L. Jorda, O. Vallcorba, J. Rius, D. Velasco and L. Juliá, Charge Transfer States in Stable Neutral and Oxidized Radical Adducts from Carbazole Derivatives, J. Org. Chem., 2014, 79, 1771–1777 CrossRef PubMed;
(c) Y. Hattori, E. Michail, A. Schmiedel, M. Moos, M. Holzapfel, I. Krummenacher, H. Braunschweig, U. Meller, J. Pflaum and C. Lambert, Luminescent Mono-, Di-, and Triradicals: Bridging Polychlorinated Triarylmethyl Radicals by Triarylamines and Triarylboranes, Chem. – Eur. J., 2019, 25, 15463–15471 CrossRef CAS PubMed;
(d) S. Mattiello, Y. Hattori, R. Kitajima, R. Matsuoka, T. Kusamoto, K. Uchida and L. Beverina, Enhancement of fluorescence and photostability of luminescent radicals by quadruple addition of phenyl groups, J. Mater. Chem. C, 2022, 10, 15028–15034 RSC.
- K. Matsuda, R. Xiaotian, K. Nakamura, M. Furukori, T. Hosokai, K. Anraku, K. Nakao and K. Albrecht, Photostability of luminescent tris(2,4,6-trichlorophenyl)methyl radical enhanced by terminal modification of carbazole donor, Chem. Commun., 2022, 58, 13443–13446 RSC.
|
This journal is © The Royal Society of Chemistry 2023 |