DOI:
10.1039/D3MA00260H
(Paper)
Mater. Adv., 2023,
4, 3628-3635
A semiconducting supramolecular novel Ni(II)-metallogel derived from 5-aminoisophthalic acid low molecular weight gelator: an efficient Schottky barrier diode application†
Received
22nd May 2023
, Accepted 16th July 2023
First published on 19th July 2023
Abstract
An outstanding approach for the development of a supramolecular metallogel with nickel(II) ion and 5-aminoisophthalic acid as a gelator (LMWG) in DMF medium has been accomplished at room temperature. Rheological studies of the supramolecular Ni(II)-metallogel established the mechanical compactness of the gel material. FESEM microstructural study and EDX elemental mapping showed flake-like morphological patterns and major chemical constituents of the Ni(II)-metallogel. The possible metallogel formation approach has been examined using FT-IR spectroscopic study. Moreover, the supramolecular Ni(II)-metallogel assemblies show electrical conductivity in metal–semiconductor (MS) junction electronic devices. The metallogel based thin film device shows an electrical conductivity of 1.53 × 10−5 S m−1. Semiconductor properties such as Schottky barrier diode nature of the synthesized Ni(II)-metallogel based devices were explored.
1. Introduction
Gels are often thought to consist of an elastic cross-linked network containing a trapped liquid.1 Gels are ubiquitous in daily life; notable examples are hair gels, toothpaste, and soft contact lenses. The field of materials science and its associated industrial applications are constantly being reinforced by molecular self-assembly, one of the main branches of supramolecular chemistry.2 The spontaneous self-assembly of molecules produces three-dimensional frameworks known as supramolecular gels.3 The gelators and solvent molecules, which are immobilised by the gelator molecules in the 3D soft gel scaffolds, are the two principal elements making up the supramolecular gel.4 The vial inversion test, which demonstrates that a vial of gel remains stable against gravity, provides the most direct primary evidence of gel formation.5 The organic and/or inorganic gelators trap various solvents, such as water (H2O),6 acetonitrile (H3C–C
N),7 ethanol (CH3CH2OH),8 methanol (CH3OH),9 dichloromethane (CH2Cl2),10 deuterated dichloromethane (CD2Cl2),11 1,2-dichlorobenzene (C6H4Cl2),12 acetone (CH3COCH3),13 carbon tetrachloride (CCl4),14 DMF ((CH3)2NC(O)H),15 tetrahydrofuran ((CH2)4O),16 dimethyl sulfoxide (C2H6OS),17 and toluene (C6H5CH3),18 to create three-dimensional gel structures.19 Polymers, such as polyester, poly(ethylene glycol), polyolefins, polycaprolactones, and polycarbonates, often serve as gelators to produce a variety of stable gel compositions.20 However, low molecular weight gelators (LMWGs), which have molecular weights under 3000, have a strong capacity in supramolecular gel formation through the immobilization of solvent molecules.21,22 Literature is full with various low molecular weight gelators, such as alkenes,23 amides,24 modified amino acids,25 urea,26 peptides,27 sugars,28 and dendrimers,29 which have wonderful gelation properties in the presence of different solvent molecules. Supramolecular gel formation is the output of intriguing non-covalent interactions, including hydrogen bonds,30 electrostatic interactions,31 hydrophobic32 and hydrophilic forces,33 van der Waals forces,30 and aryl-system-based interactions.34 Supramolecular gels have become a vital area in materials science owing to their numerous applications in both the academic and industrial arena, such as catalysis,6a,b,35 lithography,36 opto-electronic devices,37 electrochemical devices,38 chemo-sensors,39 cell culture,40 drug delivery,41 tissue engineering,42 and semiconductors.15
Metallogels are a significant class of supramolecular gels, which are formed by the incorporation of metal ions with appropriate LMWG to create a 3D supramolecular soft gel structure, which may exhibit intriguing and unusual features.5 In metallogels, metal ions impart a number of significant functionalities into the metallogel scaffolds, including redox activity,43 magnetic behaviour,44 conductivity,45 actuators,46 catalytic activity,47 optical activity.48 As smart functional materials, supramolecular metallogel systems based on transition metals have recently received interest owing to their affordability and availability. Researchers have recognized several useful metallogels of transition metal ions, such as Co(II),49–51 Ni(II),51,52 Cu(II),15d,e,g Cd(II),15a Fe(II/III),53–55 Zn(II),15h,i Mn(II),5 with strong applications in science.56 However, metallogels with Ni(II)-ion have important applications in fluorescence switching,57 electrocatalysis,58 non-linear property,59 self-healing,60 semiconducting devices,52 catalysis,61 and magnetic materials.62 Recently, Dhibar et al. have reported different metallogel-mediated metal–semiconductor (MS) junction device for Schottky barrier diode application. Metallogel-based Schottky diodes are highly significant in various applications for several reasons. First, metallogels offer tunable electronic properties that can be customized by selecting specific metals and ligands during synthesis. This tunability allows for precise control over the energy band structure and electronic behavior of the Schottky diode, enabling the creation of tailored device characteristics. Second, metallogels exhibit excellent stability, ensuring the long-term performance and reliability of Schottky diodes. Their robust structure and resistance to degradation make them suitable for deployment in diverse environments and applications. Third, metallogels can be fabricated using a range of techniques, including solution processing, self-assembly, and templating methods. This versatility in fabrication enables compatibility with various substrates and facilitates scalable production, facilitating the integration of metallogel-based Schottky diodes into different electronic devices. Furthermore, metallogels can be easily integrated with other electronic components, such as transistors and sensors, owing to their compatibility with different materials and fabrication techniques. This seamless integration allows for the development of multifunctional devices and systems with enhanced performance and expanded functionality. Overall, metallogel-based Schottky diodes offer a unique combination of tunable electronic properties, stability, versatile fabrication methods, tailored surface properties, and compatibility with other electronic components. These characteristics make them indispensable for a wide range of electronic applications, where precise control over device properties and reliable performance are paramount. Following the trend, herein, we have demonstrated the synthetic procedure of a room temperature stable metallogel of nickel(II)-ion and 5-aminoisophthalic acid as a LMWG in DMF medium (Fig. 1) for tunable, stable electronic device fabrication. The vial inversion test of the Ni(II)-metallogel (i.e. Ni@5AIA) shows the stability of Ni@5AIA gel against the gravitational force (Fig. 1). The mechanical property and morphological patterns have been established. With the aim of achieving the capability of the metallogel based metal–semiconductor (MS) junction device, we succeeded in fabricating a Schottky barrier diode (SD).
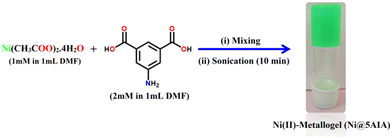 |
| Fig. 1 Synthetic procedure of gelation and photographic image of Ni(II)-metallogel (Ni@5AIA). | |
2. Experimental
2.1. Materials
Nickel(II) acetate tetrahydrate (98%) and 5-aminoisophthalic acid (94%) were purchased from Sigma-Aldrich and used as received. Dry solvents (i.e. N,N-dimethyl formamide (DMF) was used for the entire study).
2.2. Apparatus and measurements
A Shimadzu UV-3101PC spectrophotometer was used to collect UV-Vis absorption spectral data.
Rheology experiments of the gel were done using an Anton Paar 100 rheometer with a cone and plate geometry (CP 25-2). All the mechanical measurements were done by fixing the gap distance between the cone and the plate at 0.05 mm. The gels were scooped onto the plate of the rheometer. An oscillatory strain amplitude sweep experiment was performed at a constant oscillation frequency of 1 Hz for the applied strain range 0.001–10% at 20 °C.
Microstructural features were analysed using a Carl Zeiss SUPRA 55 VP FESEM instrument. The ZEISS, EVO 18 apparatus was used to perform EDX elemental mapping studies.
A Shimadzu FTIR-8400S made FTIR spectrometer was used for IR spectroscopy.
A digital gel melting point measurement apparatus (Aplab MPA-01) was used to test the Tgel of the Ni@5AIA metallogel.
Keithley 2401 sourcemeter interfaced with a computer was used to perform the current–voltage (I–V) characteristics studies of our synthesized metallogel material based thin film device.
2.3. Synthetic procedure of Ni(II)-metallogel (Ni@5AIA)
A greenish-yellow colour stable Ni(II)-metallogel (Ni@5AIA) was synthesized by a one shot mixing of 1 mL DMF solution of nickel(II) acetate tetrahydrate (0.248 g, 1 mmol) and 1 mL DMF solution of 5-aminoisophthalic acid (0.362 g, 2 mmol) followed by the continuous sonication of the mixture for 10 minutes at room temperature (Fig. 1). The vial inversion test of Ni@5AIA metallogel proved its stability against the gravitational force (Fig. 1). The minimum critical gelation concentration (MGC) of Ni@5AIA metallogel was recorded at ∼610 mg mL−1. The concentrations of Ni(CH3COO)2·4H2O and 5-aminoisophthalic acid were varied in a certain range (i.e. 30–610 mg mL−1) to evaluate the MGC of Ni@5AIA metallogel. Herein, the ratio of the Ni@5AIA metallogel forming components was maintained as [Ni(CH3COO)2·4H2O]: [5-aminoisophthalic acid] = 1
:
2, (w/w). The greenish-yellow colour stable Ni@5AIA metallogel was obtained at 610 mg mL−1 concentration of Ni(II)-acetate salt and 5-aminoisophthalic acid in DMF solvent. The gel melting temperature (Tgel) of Ni@5AIA metallogel was recorded as 120 °C ± 2 °C via a digital melting-point measuring apparatus. The stability of the Ni@5AIA metallogel in various pH media was also assessed, and the specific details can be found in the ESI.†
3. Results and discussion
3.1. Rheological analysis
The mechanical properties of the Ni@5AIA metallogel were characterized using a rheometer instrument of angular frequency and strain-sweep measurement. The higher values of storage modulus (G′) than loss modulus (G′′) confirm the sample as gel material. Rheological investigation proved that G′ of Ni@5AIA metallogel is noticeably higher than that of G′′ maintaining at a definite concentration of Ni(CH3COO)2·4H2O and 5-aminoisophthalic acid (i.e. MGC = 610 mg mL−1) (Fig. 2).
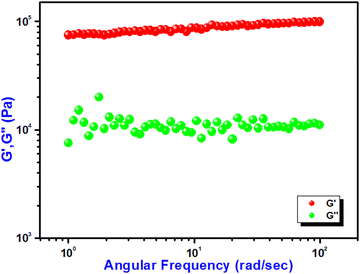 |
| Fig. 2 Angular frequency measurements vs. G′ and G′′ of Ni@5AIA metallogel. | |
The rheological data of Ni@5AIA shows G′ > G′′, which establishes the gel nature with semi-solid like performance. The average storage modulus of Ni@5AIA metallogel (i.e. G′ > 104 Pa) was detected to be significantly higher than the loss modulus (G′′) in favour of the considerable endurance limit of Ni@5AIA metallogel (Fig. 2).
Fig. 3 depicts a strain-sweep experiment on Ni@5AIA metallogel material at a constant frequency of 6.283 rad s−1. Results from the strain-sweep experiment show that the critical strain, the lowest strain for gel breakdown of Ni@5AIA metallogel, occurs at a strain of 0.45% when G′ mixes with G′′ (Fig. 3).
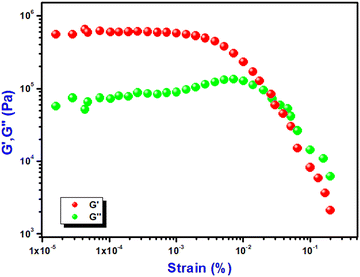 |
| Fig. 3 Strain-sweep measurements of Ni@5AIA metallogel performed at a constant frequency of 6.283 rad s−1. | |
3.2. Microstructural study
The FESEM microstructural pattern of the Ni@5AIA reveals a flake-like hierarchical network (Fig. 4a and b).
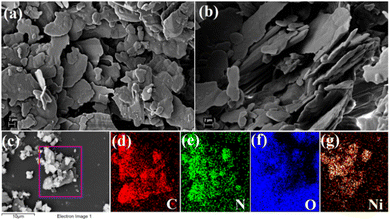 |
| Fig. 4 (a) and (b) FESEM microstructural pattern of Ni@5AIA metallogel. Chemical compositions by EDS are shown in (c)–(g). | |
The FESEM structural arrangements of the Ni@5AIA metallogel were formed due to the combination of Ni(OAc)2·4H2O and 5-aminoisophthalic acid in DMF medium over continuous sonication. EDX elemental mapping confirms the presence of C, N, O, and Ni elements of Ni(OAc)2·4H2O, 5-aminoisophthalic acid, and DMF molecules, accountable for the Ni@5AIA metallogel network formation (Fig. 4c–g).
3.3. FT-IR spectral analysis
The FT-IR spectral data of Ni@5AIA metallogel in xerogel form unveils the key peaks located at 3320–3150, 2940, 1650, 1370, and 1108 cm−1 for OH stretching, –CH stretching, C
O (carboxylic) stretching, –CH3, vs(COO), respectively, and additional peaks centered at 402 cm−1 attributed to Ni–O stretching vibrations (Fig. 5). FT-IR spectral data displays the supramolecular interactions in Ni@5AIA metallogel among the metallogel forming chemical components.
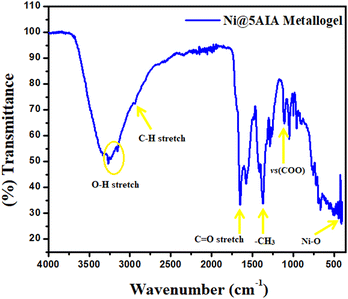 |
| Fig. 5 FT-IR spectra of the xerogel form of Ni@5AIA metallogel. | |
3.4. Fabrication of thin film device
The Schottky device for the gel was made in a sandwich configuration with ITO/Ni@5AIA metallogel/Al structure. Prior to device manufacturing, soap solution, acetone, ethanol, and distilled water were used sequentially in an ultrasonic bath to clean and dry indium tin oxide (ITO)-coated glass substrates (Scheme 1).
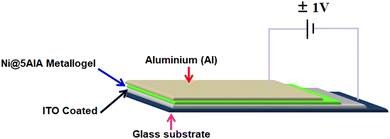 |
| Scheme 1 Schematic diagram of MS junction device based on Ni@5AIA metallogel. | |
3.5. Optical characterization
To measure the optical band gap we recorded the absorption spectra of the synthesized gel in the wavelength region from 360 to 600 nm using a UV-Vis spectrophotometer and the recorded spectra are shown in Fig. 6 (inset). The optical band gap was determined from the analysis of the Tauc plot using the equation
where α = absorption coefficient, h = Planck's constant, ν = frequency of light, A = energy dependent constant (taken as 1), Eg = band gap, n = electron transition process dependent const (for direct transition n = 1/2). (αhν)2vs. hν plot are shown in Fig. 6 where, by the extrapolation of the linear part of the curve we can conclude that the material has a direct optical band gap of 3.37 eV.
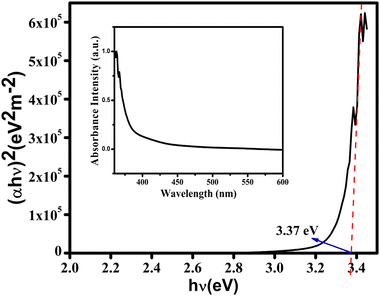 |
| Fig. 6 (αhγ)2versus hγ curves of Ni@5AIA, UV-Vis absorption spectra (inset) were determined with the help of Tauc'sequation. | |
3.6. Electrical characterization of device
We investigated the electrical properties of our synthetic gel. To explore the charge transport properties, we fabricated our synthesized gel-based thin film MS junction devices and measured current density–voltage (J–V) data within a bias range of ±1 V. The J–V characteristics of the gel-based metal–semiconductor junction device are shown in Fig. 7. Our synthetic gel-based device has an approximate conductivity of 1.53 × 10−5 S m−1, similar to that of a semiconductor. The measured J–V curve of the gel-based device shows that it has good rectifying properties, establishing Schottky diode (SD) behavior. The correction ratio (Jon/Joff) of the gel-based SD was determined as 34.77 based on the JV characteristic at ±1 V.
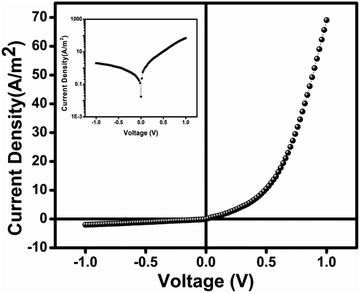 |
| Fig. 7 Current density–voltage (J–V) graph of artificial gel under dark condition. The insets show respective J vs. V plots (in log scale). | |
To examine the J–V characteristics obtained in this case, we used the thermionic emission theory and applied the technique proposed by Cheung to extract some key diode parameters.63 The following general equations were used to initiate the analysis of the J–V curve:63,64
| 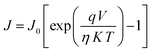 | (1) |
| 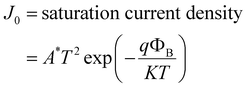 | (2) |
Each parameter has its conventional interpretation. We also calculated the series resistance, ideality factor, and impedance potential height using
eqn (3) and (4).
64,65 | 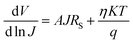 | (3) |
| 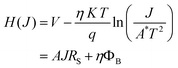 | (4) |
For complex-based devices, the ideality factor (
η) was calculated from the intercept of the d
V/d(ln
J)
vs. J plot, while the barrier height was calculated from the intercept of the
H vs. J plot (
Fig. 8). The calculated ideality factor value shows that the MS junction is not behaving ideally. This departure from the ideal value may be primarily due to impedance height, the presence of series resistance, and inhomogeneity of MS junction interface conditions.
66 The series resistance (
RS) was determined using the slope of the
H vs. J and d
V/d(ln
J)
vs. J plots (
Fig. 8).
Table 1 provides an overview of the estimated barrier potential height (
φB), series resistance (
RS), and ideality factor (
η) for the synthesised complex-based SD. The results shown in the table indicate clearly that both approaches (
eqn (3) and (4)) employing Cheung's functions produce results that are very similar. The synthesized complex can play an important role in the development of metallogel-based semiconductor devices, according to all measured parameters.
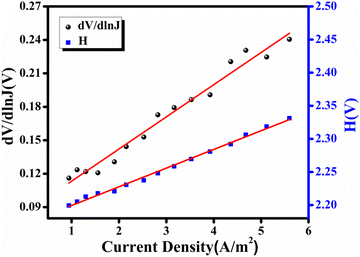 |
| Fig. 8 Under dark conditions, dV/d ln J vs. J and H(J) vs. J curves in double y axis. | |
Table 1 Electrical parameters of gel based SD
Rectification ratio |
Conductivity (S m−1) |
Series resistance (Ω) from |
Ideality factor (η) |
Barrier height φb (eV) |
dV/d ln J vs. J curve |
H vs. J curve |
34.77 |
1.53 × 10−5 |
4.17 K |
3.99 K |
3.18 |
0.68 |
The parameters of our fabricated semiconductor device were then compared with various other reported semiconductor devices and the results are presented in Table 2. From Table 2, it is clear that our synthesized Ni(II)-metallogel has greater electrical conductivity than other materials reported.
Table 2 Comparison table of electrical parameters of Ni(II)-metallogel based device with other reported results
Device based on |
Rectification ratio |
Conductivity (S m−1) |
Ideality factor (η) |
Barrier height (φB) (eV) |
Series resistance RS (Ohm) |
Ref. |
dV/d ln J vs. J |
H(J) vs. J |
Ni(II)-metallogel |
34.77 |
1.53 × 10−5 |
3.18 |
0.68 |
4170 |
3990 |
This work |
Cd-CADS |
19.51 |
1.49 × 10−5 |
0.61 |
0.62 |
2372 |
2220 |
67
|
Cd-N2H4 |
23.53 |
2.04 × 10−6 |
3.01 |
0.47 |
6730 |
6640 |
6h
|
C40H34Cu2N6O18 |
8.46 |
2.02 × 10−6 |
2.78 |
0.47 |
81.7 |
84.3 |
68
|
C20H18CuN2O10 |
8.49 |
2.34 × 10−6 |
2.08 |
0.44 |
50 150 |
53 170 |
68
|
Zn@TA |
37.06 |
7.77 × 10−6 |
3.78 |
0.47 |
3751.26 |
3587.73 |
69
|
Ni-SA |
21.54 |
11.16 × 10−6 |
1.79 |
0.66 |
1700 |
1880 |
70
|
Zn-SA |
20.83 |
6.72 × 10−6 |
2.53 |
0.70 |
2890 |
3000 |
70
|
Cd-SA |
20.11 |
4.02 × 10−6 |
2.61 |
0.73 |
4110 |
4170 |
70
|
Ni-SB |
1.64 |
2.62 × 10−6 |
3.00 |
0.74 |
18 900 |
18 830 |
71
|
Zn-SB |
14.63 |
1.18 × 10−6 |
3.93 |
0.75 |
37 340 |
3930 |
71
|
Fe-metallogel |
42.19 |
4.53 × 10−6 |
2.92 |
0.78 |
14 080 |
13 420 |
72
|
[(NiLB)2(SCN)2Mn] |
— |
5.29 × 10−6 |
1.22 |
0.70 |
4025 |
3016 |
73
|
4. Conclusions
Briefly, a novel supramolecular Ni(II)-metallogel based on 5-aminoisophthalic acid gelator was prepared by immediate mixing of nickel acetate and 5-aminoisophthalic acid in DMF followed by sonication at room temperature. Various non-covalent interactions contribute to the formation of stable Ni@5AIA metallogels at room temperature. The FESEM microstructural analysis of the Ni@5AIA metallogel revealed a flake-like hierarchical architecture of the hydrogel. The mechanical stability of the Ni@5AIA metallogel material was verified by rheological tests. Optical band-gap measurements of our synthesized Ni@5AIA metallogel suggest the semiconducting nature of the metallogel. In addition, a metal–semiconductor junction thin film device was fabricated using Au metal and semiconducting Ni@5AIA metallogel. The nonlinear charge transport of the device obtained from the I–V characteristic graph confirmed the fabrication of a Schottky diode. Thus, the present study of a sandwich-like configuration of ITO/Ni@5AIA metallogel/Au suggests the future possibility of achieving supramolecular Ni2+ metallogel based electronic devices for advanced technology. Indeed, this study on Ni@5AIA metallogel based on 5-aminoisophthalic acid and a nickel(II) source indicates a pioneering method and metallogel for the fabrication of semiconducting devices.
Conflicts of interest
The authors declare no competing financial interests.
Acknowledgements
S. D. is grateful to the UGC, New Delhi, for awarding him Dr DS Kothari Postdoctoral Fellowship (Award letter number: No. F.4-2/2006 (BSR)/CH/19-20/0224). S. B. gratefully acknowledges DST Inspire Faculty Research Grant (Faculty Registration No. IFA18-CH304; DST/INSPIRE/04/2018/000329).
Notes and references
- R. Kuosmanen, K. Rissanen and E. Sievänen, Chem. Soc. Rev., 2020, 49, 1977–1998 RSC
.
- G. M. Whitesides, J. P. Mathias and C. T. Seto, Science, 1991, 254, 1312–1319 CrossRef CAS PubMed
.
- J. W. Steed, Chem. Commun., 2011, 47, 1379–1383 RSC
.
- P. Dastidar, Chem. Soc. Rev., 2008, 37, 2699–2715 RSC
.
- S. Dhibar, A. Dey, S. Majumdar, D. Ghosh, P. P. Ray and B. Dey, ACS Appl. Electron. Mater., 2019, 1, 1899–1908 CrossRef CAS
.
-
(a) S. Dhibar, D. Ghosh, S. Majumdar and B. Dey, ACS Omega, 2020, 5, 2680–2689 CrossRef CAS PubMed
;
(b) S. Dhibar, A. Dey, R. Jana, A. Chatterjee, G. K. Das, P. P. Ray and B. Dey, Dalton Trans., 2019, 48, 17388–17394 RSC
;
(c) S. Dhibar, R. Jana, P. P. Ray and B. Dey, J. Mol. Liq., 2019, 289, 111–126 CrossRef
;
(d) S. Dhibar, A. Dey, D. Ghosh, A. Mandal and B. Dey, J. Mol. Liq., 2019, 276, 184–193 CrossRef CAS
;
(e) S. Dhibar, A. Dey, A. Dey, S. Majumdar, A. Mandal, P. P. Ray and B. Dey, New J. Chem., 2019, 43, 15691–15699 RSC
;
(f) D. Ghosh, S. Dhibar, A. Dey, S. Mukherjee, N. Joardar, S. P. Sinha Babu and B. Dey, J. Mol. Liq., 2019, 280, 1–12 CrossRef
;
(g) S. Majumdar, T. Singha, S. Dhibar, A. Mandal, P. K. Datta and B. Dey, ACS Appl. Electron. Mater., 2020, 2, 3678–3685 CrossRef CAS
;
(h) S. Majumdar, A. Dey, R. Sahu, S. Dhibar, P. P. Ray and B. Dey, ACS Appl. Nano Mater., 2020, 3, 11025–11036 CrossRef CAS
.
-
(a) C. Po, Z. Ke, A. Y. Y. Tam, H. F. Chow and V. W. W. Yam, Chem. – Eur. J., 2013, 19, 15735–15744 CrossRef CAS PubMed
;
(b) S. Ganta and D. K. Chand, Inorg. Chem., 2018, 57, 3634–3645 CrossRef CAS PubMed
;
(c) P. Chen, Q. Li, S. Grindy and N. Holten-Andersen, J. Am. Chem. Soc., 2015, 137, 11590–11593 CrossRef CAS PubMed
.
-
(a) Q. Lin, Q.-P. Yang, B. Sun, Y.-P. Fu, X. Zhu, T.-B. Wei and Y.-M. Zhang, Soft Matter, 2014, 10, 8427–8432 RSC
;
(b) A. M. Amacher, J. Puigmartí-Luis, Y. Geng, V. Lebedev, V. Laukhin, K. Kramer, J. Hauser, D. B. Amabilino, S. Decurtins and S.-X. Decurtins, Chem. Commun., 2015, 51, 15063–15066 RSC
;
(c) S. Sarkar, S. Dutta, P. Bairi and T. Pal, Langmuir, 2014, 30, 7833–7841 CrossRef CAS PubMed
.
-
(a) C. K. Karan and M. Bhattacharjee, ACS Appl. Mater. Interfaces, 2016, 8, 5526–5535 CrossRef CAS PubMed
;
(b) S. Dey, D. Datta, K. Chakraborty, S. Nandi, A. Anoop and T. Pathak, RSC Adv., 2013, 3, 9163–9166 RSC
;
(c) M.-O. M. Piepenbrock, N. Clarke and J. W. Steed, Soft Matter, 2010, 6, 3541–3547 RSC
;
(d) M.-O. M. Piepenbrock, N. Clarke and J. W. Steed, Langmuir, 2009, 25, 8451–8456 CrossRef CAS PubMed
.
-
(a) Z. Yao, Z. Wang, Y. Yu, C. Zeng and K. Cao, Polymer, 2017, 119, 98–106 CrossRef CAS
;
(b) P. Rajamalli, P. Malakar, S. Atta and E. Prasad, Chem. Commun., 2014, 50, 11023–11025 RSC
.
- K. Mitsumoto, J. M. Cameron, R.-J. Wei, H. Nishikawa, T. Shiga, M. Nihei, G. N. Newton and H. Oshio, Chem. – Eur. J., 2017, 23, 1502–1506 CrossRef CAS PubMed
.
- X.-Q. Wang, W. Wang, G.-Q. Yin, Y.-X. Wang, C.-W. Zhang, J.-M. Shi, Y. Yu and H.-B. Yang, Chem. Commun., 2015, 51, 16813–16816 RSC
.
- B. Jiang, L.-J. Chen, G.-Q. Yin, Y.-X. Wang, W. Zheng, L. Xu and H.-B. Yang, Chem. Commun., 2017, 53, 172–175 RSC
.
-
(a) F. Gou, J. Cheng, X. Zhang, G. Shen, X. Zhou and H. Xiang, Eur. J. Inorg. Chem., 2016, 4862–4866 CrossRef CAS
;
(b) N. Kelly, K. Gloe, T. Doert, F. Hennersdorf, A. Heine, J. Marz, U. Schwarzenbolz, J. J. Weigand and K. J. Gloe, Organomet. Chem., 2016, 821, 182–191 CrossRef CAS
.
-
(a) S. Dhibar, A. Dey, S. Majumdar, D. Ghosh, A. Mandal, P. P. Ray and B. Dey, Dalton Trans., 2018, 47, 17412–17420 RSC
;
(b) S. Dhibar, A. Dey, D. Ghosh, S. Majumdar, A. Dey, P. Mukherjee, A. Mandal, P. P. Ray and B. Dey, ChemistrySelect, 2019, 4, 1535–1541 CrossRef CAS
;
(c) S. Dhibar, A. Dey, S. Majumdar, A. Dey, P. P. Ray and B. Dey, Ind. Eng. Chem. Res., 2020, 59, 5466–5473 CrossRef CAS
;
(d) S. Dhibar, A. Dey, S. Majumdar, P. P. Ray and B. Dey, Int. J. Energy Res., 2021, 45, 5486–5499 CrossRef CAS
;
(e) S. Dhibar, S. K. Ojha, A. Mohan, S. P. C. Prabhakaran, S. Bhattacharjee, K. Karmakar, P. Karmakar, P. Predeep, A. K. Ojha and B. Saha, New J. Chem., 2022, 46, 17189–17200 RSC
;
(f) S. Dhibar, H. Dahiya, K. Karmakar, S. Kundu, S. Bhattacharjee, G. C. Nayak, P. Karmakar, G. D. Sharma and B. Saha, J. Mol. Liq., 2023, 370, 121020 CrossRef CAS
;
(g) S. Dhibar, A. Dey, A. Dalal, S. Bhattacharjee, R. Sahu, R. Sahoo, A. Mondal, S. M. Rahaman, S. Kundu and B. Saha, J. Mol. Liq., 2023, 370, 121021 CrossRef CAS
;
(h) S. Dhibar, S. Babu, A. Mohan, G. K. Chandra, S. Bhattacharjee, K. Karmakar, P. Karmakar, S. M. Rahaman, P. Predeep and B. Saha, J. Mol. Liq., 2023, 375, 121348 CrossRef CAS
;
(i) K. Karmakar, A. Dey, S. Dhibar, R. Sahu, S. Bhattacharjee, P. Karmakar, P. Chatterjee, A. Mondal and B. Saha, RSC Adv., 2023, 13, 2561–2569 RSC
;
(j) S. Dhibar, B. Pal, K. Karmakar, S. Kundu, S. Bhattacharjee, R. Sahoo, S. M. Rahaman, D. Dey, P. P. Ray and B. Saha, ChemistrySelect, 2023, 8, e202204214 CrossRef CAS
.
-
(a) Z. Yao, Z. Wang, Y. Yu, C. Zeng and K. Cao, Polymer, 2017, 119, 98–106 CrossRef CAS
;
(b) P. Rajamalli, P. Malakar, S. Atta and E. Prasad, Chem. Commun., 2014, 50, 11023–11025 RSC
.
-
(a) S. Ganta and D. K. Chand, Dalton Trans., 2015, 44, 15181–15188 RSC
;
(b) L. Yang, L. Luo, S. Zhang, X. Su, J. Lan, C.-T. Chen and J. You, Chem. Commun., 2010, 46, 3938–3940 RSC
;
(c) B. Xing, M.-F. Choi, Z. Zhou and B. Xu, Langmuir, 2002, 18, 9654–9658 CrossRef CAS
;
(d) X. Ma, S. Liu, Z. Zhang, Y. Niu and J. Wu, Soft Matter, 2017, 13, 8882–8885 RSC
.
- C. A. Offiler, C. D. Jones and J. W. Steed, Chem. Commun., 2017, 53, 2024–2027 RSC
.
- X. Yu, Z. Wang, Y. Li, L. Geng, J. Ren and G. Feng, Inorg. Chem., 2017, 56, 7512–7518 CrossRef CAS PubMed
.
- M. Suzuki and K. Hanabusa, Chem. Soc. Rev., 2010, 39, 455–463 RSC
.
- P. Terech and R. G. Weiss, Chem. Rev., 1997, 97, 3133–3159 CrossRef CAS PubMed
.
- J. Raeburn and D. J. Adams, Chem. Commun., 2015, 51, 5170–5180 RSC
.
- S. J. Wezenberg, C. M. Croisetu, M. C. A. Stuartab and B. L. Feringa, Chem. Sci., 2016, 7, 4341–4346 RSC
.
- N. Shi, G. Yin, H. Li, M. Hana and Z. Xu, New J. Chem., 2008, 32, 2011–2015 RSC
.
- K. Hanabusa, K. Hiratsuka, M. Kimura and H. Shirai, Chem. Mater., 1999, 11, 649–655 CrossRef CAS
.
- J. W. Steed, Chem. Soc. Rev., 2010, 39, 3686–3699 RSC
.
- C. Tomasini and N. Castellucci, Chem. Soc. Rev., 2013, 42, 156–172 RSC
.
- A. Prathap and K. M. Sureshan, Langmuir, 2019, 35, 6005–6014 CrossRef CAS PubMed
.
- D. K. Smith, Adv. Mater., 2006, 18, 2773–2778 CrossRef CAS
.
-
R. G. Weiss and P. Terech, Molecular Gels: Materials with Self-Assembled Fibrillar Networks, Springer, Dordrecht, 2005 Search PubMed
.
-
(a) T.-A. Asoh and A. Kikuchi, Chem. Commun., 2012, 48, 10019–10021 RSC
;
(b) X. Wang, F. Liu, X. Zheng and J. Sun, Angew. Chem., Int. Ed., 2011, 50, 11378–11381 CrossRef CAS PubMed
;
(c) H. Wang, M. B. Hansen, D. W. P. M. Löwik, J. C. M. van Hest, Y. Li, J. A. Jansen and S. C. G. Leeuwenburgh, Adv. Mater., 2011, 23, H119–H124 CrossRef CAS PubMed
.
-
(a) A. Y.-Y. Tam and V. W.-W. Yam, Chem. Soc. Rev., 2013, 42, 1540–1567 RSC
;
(b) C. Tomasini and N. Castellucci, Chem. Soc. Rev., 2013, 42, 156–172 RSC
;
(c) L. Meazza, J. A. Foster, K. Fucke, P. Metrangolo, G. Resnati and J. W. Steed, Nat. Chem., 2013, 5, 42–47 CrossRef CAS PubMed
.
-
(a) M. Shirakawa, N. Fujita and S. Shinkai, J. Am. Chem. Soc., 2003, 125, 9902–9903 CrossRef CAS PubMed
;
(b) J. R. Moffat, G. J. Seeley, J. T. Carter, A. Burgess and D. K. Smith, Chem. Commun., 2008, 4601–4603 RSC
.
-
(a) Y. Xu, Q. Wu, Y. Sun, H. Bai and G. Shi, ACS Nano, 2010, 4, 7358–7362 CrossRef CAS PubMed
;
(b) S. Burattini, B. W. Greenland, D. H. Merino, W. Weng, J. Seppala, H. M. Colquhoun, W. Hayes, M. E. Mackay, I. W. HamLey and S. J. Rowan, J. Am. Chem. Soc., 2010, 132, 12051–12058 CrossRef CAS PubMed
.
- W. Fang, Y. Zhang, J. Wu, C. Liu, H. Zhu and T. Tu, Chem. – Asian J., 2018, 13, 712–729 CrossRef CAS PubMed
.
- J. Kim, J. A. Hanna, M. Byun, C. D. Santangelo and R. C. Hayward, Science, 2012, 335, 1201–1205 CrossRef CAS PubMed
.
- A. R. Hirst, B. Escuder, J. F. Miravet and D. K. Smith, Angew. Chem., Int. Ed., 2008, 47, 8002–8018 CrossRef CAS PubMed
.
- X. Cheng, J. Pan, Y. Zhao, M. Liao and H. Peng, Adv. Energy Mater., 2018, 8, 1702184 CrossRef
.
-
(a) S. Sarkar, S. Dutta, S. Chakrabarti, P. Bairi and T. Pal, ACS Appl. Mater. Interfaces, 2014, 6, 6308–6316 CrossRef CAS PubMed
;
(b) Q. Lin, T.-T. Lu, X. Zhu, B. Sun, Q.-P. Yang, T.-B. Wei and Y.-M. Zhang, Chem. Commun., 2015, 51, 1635–1638 RSC
.
- W. Wang, H. Wang, C. Ren, J. Wang, M. Tan, J. Shen, Z. Yang, P. G. Wang and L. Wang, Carbohydr. Res., 2011, 346, 1013–1017 CrossRef CAS PubMed
.
- M. R. Saboktakin and R. M. Tabatabaei, Int. J. Biol. Macromol., 2015, 75, 426–436 CrossRef CAS PubMed
.
- Y. Zhao, S. Song, X. Ren, J. Zhang, Q. Lin and Y. Zhao, Chem. Rev., 2022, 122, 5604–5640 CrossRef CAS PubMed
.
- W.-L. Guan, K. M. Adam, M. Qiu, Y.-M. Zhang, H. Yao, T.-B. Wei and Q. Lin, Supramol. Chem., 2020, 32, 578–596 CrossRef CAS
.
- W. H. Binder, L. Petraru, T. Roth, P. W. Groh, V. Pálfi, S. Keki and B. Ivan, Adv. Funct. Mater., 2007, 17, 1317–1326 CrossRef CAS
.
- A. Khan, R.
R. Kisannagar, C. Gouda, D. Gupta and H.-C. Lin, J. Mater. Chem. A, 2020, 8, 19954–19964 RSC
.
- B. Xue, M. Qin, T. Wang, J. Wu, D. Luo, Q. Jiang, Y. Li, Y. Cao and W. Wang, Adv. Funct. Mater., 2016, 26, 9053–9062 CrossRef CAS
.
- B. Zhang and J. N. H. Reek, Chem. – Asian J., 2021, 16, 3851–3863 CrossRef CAS PubMed
.
- S. Biswas, U. Chatterjee, S. Sarkar, F. Khan, D. Bera, M. Mukhopadhyay, S. Goswami, S. Chakrabarti and S. Das, Colloids Surf., B, 2020, 188, 110803 CrossRef CAS PubMed
.
- N. Kelly, K. Gloe, T. Doert, F. Hennersdorf, A. Heine, J. Maerz, U. Schwarzenbolz, J. J. Weigand and K. Gloe, J. Organomet. Chem., 2016, 821, 182–191 CrossRef CAS
.
- L. Yan, C. Liu, L. Shen, J. Li, X. Liu, M. Lv, C. Su and Z. Ye, Chem. Lett., 2018, 47, 640–642 CrossRef CAS
.
- J. H. Lee, Y. E. Baek, K. Y. Kim, H. Choi and J. H. Jung, Supramol. Chem., 2016, 28, 870–873 CrossRef CAS
.
- S. Dhibar, S. K. Ojha, K. Karmakar, P. Karmakar, S. Bhattacharjee, P. Chatterjee, A. K. Ojha and B. Saha, Chem. Afr., 2023 DOI:10.1007/s42250-023-00680-w
.
- J. Chen, T. Wang and M. Liu, Inorg. Chem. Front., 2016, 3, 1559–1565 RSC
.
- J.-L. Zhong, X.-J. Jia, H.-J. Liu, X.-Z. Luo, S.-G. Hong, N. Zhang and J.-B. Huang, Soft Matter, 2016, 12, 191–199 RSC
.
- L. Arnedo-Sánchez, S. Bhowmik, S. Hietala, R. Puttreddy, M. Lahtinen, L. D. Cola and K. Rissanen, Dalton Trans., 2017, 46, 7309–7316 RSC
.
- X. Li, J. Shi, Y. Gao, H.-C. Lin and B. Xu, J. Am. Chem. Soc., 2011, 133, 17513–17518 CrossRef CAS PubMed
.
- V. Singh, S. Kala, T. Rom, A. K. Paul and R. Pandey, Dalton Trans., 2023, 52, 7088–7103 RSC
.
- H. Guo, Q. Feng, K. Xu, J. Xu, J. Zhu, C. Zhang and T. Liu, Adv. Funct. Mater., 2019, 29, 1903660 CrossRef
.
- G. Lepcha, T. Singha, S. Majumdar, A. K. Pradhan, K. S. Das, P. K. Datta and B. Dey, Dalton Trans., 2022, 51, 13435–13443 RSC
.
- P. Terech, M. Yan, M. Maréchal, G. Royal, J. Galveza and S. K. P. Velu, Phys. Chem. Chem. Phys., 2013, 15, 7338–7344 RSC
.
- N. Malviya, C. Sonkar, B. K. Kundu and S. Mukhopadhyay, Langmuir, 2018, 34, 11575–11585 CrossRef CAS PubMed
.
-
(a) E. M. M. Ibrahim, L. H. Abdel-Rahman, A. M. Abu-Dief, A. Elshafaie, S. K. Hamdan and A. M. Ahmed, Mater. Res. Bull., 2018, 107, 492–497 CrossRef CAS
;
(b) A. Elshafaie, L. H. Abdel-Rahman, A. M. Abu-Dief, S. K. Hamdan, A. M. Ahmed and E. M. M. Ibrahim, NANO, 2018, 13, 1850074 CrossRef CAS
;
(c) L. H. Abdel Rahman, A. M. Abu-Dief, R. M. El-Khatib, S. M. Abdel-Fatah, A. M. Adam and E. M. M. Ibrahim, Appl. Organomet. Chem., 2018, 32, e4174 CrossRef
; L. H. Abdel Rahman, A. M. Abu-Dief, R. M. El-Khatib, S. M. Abdel-Fatah, A. M. Adam and E. M. M. Ibrahim, Appl. Organomet. Chem., 2018, 32, e4174 Search PubMed
.
- R. Jana, S. Sil, A. Dey, J. Datta and P. P. Ray, AIP Adv., 2018, 8, 125104 CrossRef
.
- J. Datta, A. Dey, S. K. Neogi, M. Das, S. Middya, R. Jana, S. Bandopadhyay, A. Layek and P. P. Ray, IEEE Trans. Electron Devices, 2017, 64, 4724–4730 CAS
.
- S. K. Cheung and N. W. Cheung, Extraction of Schottky diode parameters from forward current-Voltage characteristics, Appl. Phys. Lett., 1986, 49, 85–87 CrossRef CAS
.
- J. Datta, M. Das, S. Sil, S. Kumar, A. Dey, R. Jana, S. Bandyopadhyay and P. P. Ray, Mater. Sci. Semicond. Process., 2019, 91, 133–145 CrossRef CAS
.
- S. Majumdar, B. Pal, R. Sahu, K. S. Das, P. P. Ray and B. Dey, Dalton Trans., 2022, 51, 9007–9016 RSC
.
- A. Hossain, A. Dey, S. K. Seth, P. P. Ray, P. Ballester, R. G. Pritchard, J. Ortega-Castro, A. Frontera and S. Mukhopadhyay, ACS Omega, 2018, 3, 9160–9171 CrossRef CAS PubMed
.
- S. Majumdar, A. Dey, R. Sahu, G. Lepcha, A. Dey, P. P. Ray and B. Dey, Mater. Res. Bull., 2023, 157, 112003 CrossRef CAS
.
- G. Lepcha, S. Majumdar, B. Pal, K. T. Ahmed, I. Pal, B. Satpati, S. R. Biswas, P. P. Ray and B. Dey, Langmuir, 2023, 39, 7469–7483 CrossRef CAS PubMed
.
- G. Lepcha, B. Pal, S. Majumdar, K. T. Ahmed, I. Pal, S. R. Biswas, P. P. Ray and B. Dey, Mater. Adv., 2023, 4, 2595–2603 RSC
.
- S. Saha, B. Pal, K. S. Das, P. K. Ghose, A. Ghosh, A. De, A. K. Das, P. P. Ray and R. Mondal, ChemistrySelect, 2022, 7, e202203307 CrossRef CAS
.
- M. Das, M. Das, S. Ray, U. K. Das, S. Laha, P. P. Ray, B. C. Samanta and T. Maity, New J. Chem., 2022, 46, 21103–21114 RSC
.
|
This journal is © The Royal Society of Chemistry 2023 |
Click here to see how this site uses Cookies. View our privacy policy here.