DOI:
10.1039/D3MA00235G
(Paper)
Mater. Adv., 2023,
4, 2999-3009
An efficient photocatalysis-self-Fenton system based on Fe(II)-MOF/g-C3N4 for direct hydroxylation of benzene to phenol†
Received
14th May 2023
, Accepted 14th June 2023
First published on 14th June 2023
Abstract
In order to avoid the issues causing weaknesses in the cumene method, a heterojunction catalyst based on Fe(II)-MOF/g-C3N4 was synthesized via in situ synthesis. The combination of Fe(II)-MOF and g-C3N4 was stable. With the synergistic effect of photocatalysis and the Fenton effect, the catalyst could achieve efficient direct hydroxylation of benzene to phenol. The yield and selectivity were 16.4% and 98.8% under the optimum conditions, respectively. And the catalytic activity of the heterojunction still sustained well after 5 reaction cycles. Hence, the explored photocatalysis-self-Fenton system could be applied in the field of direct hydroxylation of benzene to phenol.
Introduction
Phenol, an important industrial chemical, was widely used in the production of various chemical products and intermediates such as phenol resin and bisphenol A. Recently, the cumene method has been found to be the main way of industrial phenol production.1 However, this method has some weaknesses such as high energy consumption, requirement of strong acids and low yield of phenol every time.2 Due to the mild reaction conditions, small energy consumption, and few by-products, the direct hydroxylation of benzene to produce phenol has attracted wide attention. Nevertheless, most of the traditional benzene hydroxylation catalysts needed high temperature and high pressure, and the reaction of phenol was vigorous and it was easily overoxidized.3 Photocatalysis is a green technology that converts renewable solar energy directly into chemical energy, and it could achieve direct hydroxylation of high-efficiency benzene to produce phenol.4
As a new type of porous crystal material, metal–organic frameworks (MOFs) have been widely applied in the field of photocatalysis with their large specific surface area, regular aperture, open pore channels and abundant catalytic sites.5 In particular, due to the presence of extensive ferric oxygen (Fe–O) clusters, iron-based MOFs were attractive in photocatalysis.6 For instance, Zhang et al. prepared a mixed valent Fe-based MOF, which had an excellent catalytic performance in photocatalytic hydrogen production.7 In addition, due to the Fenton reaction, the iron-based MOFs could react with H2O2 to produce a hydroxyl radical, and the photocatalytic benzene hydroxylation could be achieved to prepare phenol.8 Tu has synthesized a novel Fe-based MOF with a topological framework as a recyclable heterogeneous catalyst for benzene hydroxylation.9 The Yang group reported a mixed valent Fe-based MOF, which greatly enhanced Fenton performance and further improved the efficiency of benzene hydroxylation to produce phenol.10
To a certain extent, although MOF materials could be applied to the direct hydroxylation of benzene to prepare phenol, its limited response to light impeded its wide application. Due to its graphene-like two-dimensional (2D) structure, suitable band gap (2.7 eV), visible light absorption, chemical and thermal stability, non-metallic properties and photocatalytic properties, C3N4 had attracted extensive attention as a sustainable material.11 Sanny et al. had designed a bimetallic CuAg@g-C3N4 catalyst system by impregnating copper and silver nanoparticles on the surface of g-C3N4. It achieved ideal results in benzene hydroxylation under visible light.12 In addition, when g-C3N4 was combined with an MOF, it facilitated not only the response of the MOF substrate to light, but also the diffusion of the reactants and products.13 For instance, Fu et al. pointed out that the combination of g-C3N4 and MOF heterojunctions could greatly improve the efficiency of carrier separation and then photocatalytic activity.14 Qi showed that the combination of g-C3N4 and MOFs not only facilitated the absorption of visible light, but also facilitated the separation of electrons and holes, thus improving the performance of the catalyst.15
Herein, a novel photocatalyst Fe(II)-MOF/g-C3N4 was prepared by an in situ composite technique. Fe-based MOFs can undergo the Fenton reaction with hydrogen peroxide to release hydroxyl radicals. g-C3N4 possesses a suitable band gap and could realize the efficient absorption of visible light. The combination of the Fe(II)-MOF and g-C3N4 would exhibit synergistic effects of the Fenton effect and photocatalysis with relatively high yield and better selectivity in the direct hydroxylation of benzene to phenol. Therefore, the synthesized catalyst was expected to play an important role in the field of direct hydroxylation of phenol.
Experimental
Materials and synthesis
Ferrous acetate (>90%) was obtained from Shanghai Aladdin Biochemical Technology Co., Ltd. 1,4-Benzoquinone (97%) was obtained from Guangdong Wong Jiang Chemical Reagent Co., Ltd. 2-Aminoterephthalic acid (AR) was purchased from Zhengzhou Alfa Chemical Co., Ltd. 30% hydrogen peroxide and N,N-dimethylformamide (AR) were obtained from Tianjin Kemiou Chemical Reagent Co., Ltd. The other chemicals were commercially obtained and used without further purification.
Synthesis of the ferrous acetate MOF (Fe(II)-MOF)
In order to embed unstable Fe2+ into the skeleton structure of MOF, Fe(II)-MOF was prepared by the slight adjustment of the synthesis method reported by the Xu team.16 Firstly, Ferrous acetate (1.2 g, 6.90 mmol) was dissolved in 15 mL DMF/ethanol mixture (V/V, 1
:
1). And then, 30 mL DMF solution containing 2-aminoterephthalic acid (1.0 g, 5.52 mmol) was slowly injected into the abovementioned solution under stirring at room temperature. When the solution was mixed evenly, the reaction was heated to 45 °C and stirring was continued for 1 h at a speed of 500 rpm under nitrogen. The product was collected by centrifugation and washed with ethanol several times, dried in a vacuum, and labeled as Fe(II)-MOF for further use.
Synthesis of layered g-C3N4 nanosheets
Layered g-C3N4 nanosheets were prepared by a two-step calcination method. A certain amount of melamine was transferred into a quartz boat and placed in a closed tubular furnace. The temperature was increased to 550 °C at a heating rate of 5 °C min−1, kept for 2 hours, and then the reaction was continued in a flowing air atmosphere for 4 hours. After cooling to room temperature, the grinder ground light yellow powder was labeled g-C3N4.17
Synthesis of Fe(II)-MOF/g-C3N4 composites
The synthesis procedure for Fe(II)-MOF/g-C3N4 was similar to that described above for the preparation of Fe(II)-MOF, except that 0.5 g of layered g-C3N4 powder was added to the solution before the addition of 2-aminoterephthalic acid. Then the reaction time was extended to 4 h. The prepared sample was labeled as Fe(II)-MOF/g-C3N4.16
Characteristics conditions of photocatalytic hydroxylation
The evaluation of photocatalytic performance was carried out in quartz reactors. The LED lamp (5 W) which emitted simulated sunlight full spectrum irradiation (380–760 nm) was used as the light source. Specifically, Fe(II)-MOF/g-C3N4 composites, acetonitrile, trifluoroacetic acid and benzene were added to the reactor, and gently stirred for a period of time. When the temperature of the solution was increased to the setting temperature, the lamp was turned on. H2O2 was titrated into the solution within 30 min. The reaction was maintained for a period. Then, the solution was treated by centrifugation. The qualitative and quantitative analysis of the supernatant was performed using toluene as an internal standard by gas chromatography–mass spectrometry (GC–MS qp2010) and gas chromatography (Agilent 6820). In parallel research experiments, the best reaction conditions were explored by adjusting the ratio of H2O2 and benzene, reaction time and solvent.
The conversion of benzene, the selectivity of phenol and the yield of phenol are calculated as follows:
Characterization of the Fe(II)-MOF/g-C3N4 composites
The morphology of the catalysts was verified by scanning electron microscopy (Phenom Prox) with an operating voltage of 10 kV. The X-ray diffraction (Rigaku Ulitima IV) pattern was obtained with Cu Kα radiation at a scan speed of 10 min−1 from 5 to 60°. The steady-state photoluminescence was tested on a Hitachi F-7100 spectrofluorometer at room temperature. The transient photocurrent measurements, electrochemistry impedance spectroscopy (EIS) and Mott–Schottky plots were evaluated using the electrochemical workstation (CHI600E). The electrochemical information was collected using a standard three-electrode cell: Ag/AgCl electrode (SCE) as the reference electrode and Pt electrode as the counter electrode. The working electrode was prepared using a rectangular FTO glass sheet (2 cm × 1 cm) on which was spread 50 μL N,N-dimethylformamide solution of photocatalysts (10 mg mL−1) evenly. The prepared electrode should be dried in the oven at 80 °C for 2 h.
The quantitative analysis of phenol was performed by gas chromatography (Techcomp GC7900), the injection port temperature was 210 °C, FID was selected as the detector and the temperature was set at 240 °C.
Results and discussion
Sample structure characterization
The crystal structure of the photocatalyst was characterized and analyzed using an X-ray diffractometer. As shown in Fig. 1(a), a series of diffraction peaks of Fe(II)-MOF could be observed such as 9°, 11.8°, 18.08°, 18.99°, 22.25°, 27.6°, and 30.9°, which were consistent with the results reported in the related literature, and no other impurity peaks were observed, that indicated the Fe(II)-MOF material was successfully prepared in the experiment, and the synthesized material had high crystallinity.18 In addition, it could be clearly observed that the Fe(II)-MOF/g-C3N4 and Fe(II)-MOF had very similar XRD peaks, which confirmed that the incorporation of g-C3N4 did not change the stable skeleton structure of MOF material, and a new smaller peak was found at 2θ = 27.7° in the XRD diffraction pattern of the composite material, which could be considered a typical interlayer stack peak (002) attributed to g-C3N4.19 Therefore, XRD results indicated that the two materials were successfully combined.
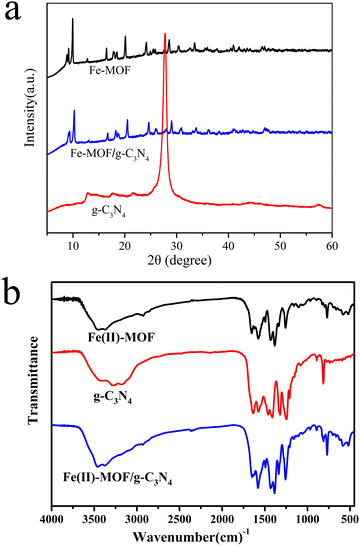 |
| Fig. 1 XRD patterns (a) and infrared spectra (b) of Fe(II)-MOF, layered g-C3N4, and Fe(II)-MOF/g-C3N4. | |
Fourier infrared spectroscopy was used as an analytical tool to characterize the structural characteristics of photocatalyst samples. As shown in Fig. 1(b), the absorption peaks near 1643 cm−1 and 1427 cm−1 were attributed to the symmetric and asymmetric stretching of –COOH in the backbone of the organic ligand (2-amino terephthalate) connected to Fe2+, respectively, which proved that it contained the carboxyl group.16 In addition, the absorption peak at 521 cm−1 corresponded to the vibration peak of Fe–O, which further confirmed the formation of the Fe(II)-MOF material.20 For layered g-C3N4, the wide absorption band between 3000 cm−1 and 3500 cm−1 was caused by the N–H and O–H stretching patterns. The sharp absorption band at 812 cm−1 was attributed to the bending vibration absorption of the carbon nitrogen ring (C–NH–C) in the triazine ring structure.21 Peaks at 1633 cm−1 and 1247 cm−1 were associated with the typical –C
N– tensile vibrations of heptazine derived repeat units and the C–N stretching of aromatic groups, respectively.22 When g-C3N4 was introduced into MOF, the same position peak as mentioned above could be observed in the spectrum of the composite material, that confirmed the successful preparation of Fe(II)-MOF/g-C3N4. It was worth noting that the peak intensity of g-C3N4 was generally reduced, which might be caused by the low content in the composite material, which was consistent with XRD results.
The surface morphology and elemental distribution information of samples were obtained by scanning electron microscopy and EDS analysis. The Fe(II)-MOF exhibited a small and uniform spindle-like morphology (Fig. 2(a) and Fig. SI1, ESI†).24 As shown in Fig. 2(b), layered g-C3N4 formed by continuous calcination of melamine in tubular furnace obviously presented a stacked sheet structure and had a larger specific surface area than the massive g-C3N4, which was favorable for photocatalytic reactions.23 And it could be observed that Fe(II)-MOF and g-C3N4 were tightly bound together (Fig. 2(c)), the relatively large particle size might be caused by the longer reaction time of the composite than that of pure Fe(II)-MOF. And then, the EDS results showed that Fe, C and N elements were uniformly distributed on the composite material, which could prove the successful preparation of Fe(II)-MOF/g-C3N4.
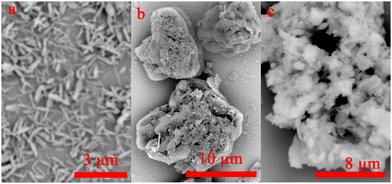 |
| Fig. 2 SEM images of (a) Fe(II)-MOF, (b) layered g-C3N4, (c) Fe(II)-MOF/g-C3N4. | |
In order to better analyze the composition and valence of the elements on the surface of the photocatalyst sample, the X-ray photoelectron spectra of the prepared sample were tested, and the results are shown in Fig. 3(a). The full-scan spectrogram of Fe(II)-MOF/g-C3N4 further proved the presence of Fe, C, N and O in the composite sample, which was consistent with the EDS results. The high-resolution C 1S XPS spectrum could be decomposed into four peaks located at 284.8 eV (C1), 285.9 eV (C2), 287.0 eV, (C3) and 288.7 eV (C4) (Fig. 3(b)). Major C1 was usually attributed to standard carbon, while C2 and C4 peaks at 285.9 and 288.7 eV were attributed to carboxyl groups (O–C
O) in organic ligands.25 The smaller peak (287.0 eV) C3 in the photocatalytic composite Fe(II)-MOF/g-C3N4 was assigned to sp2 hybrid carbon atoms (N–C
N) in g-C3N4.26 The N 1s XPS spectra of the two samples were deconvoluted into three peaks located at 398.5 and 400.4, corresponding to bicoordinated nitrogen (N2C), and tricoordinated nitrogen (N3C) in the heptazine framework, respectively (Fig. 3(e)).27 By analyzing the high-resolution XPS spectrum of Fe 2p of Fe(II)-MOF/g-C3N4 (Fig. 3(c)), three peaks could be further fitted, and peaks with binding energies of 711.4 eV, 717.3 eV and 724.7 eV could be assigned to Fe2+ 2p3/2, satellite peaks and Fe2+ 2p1/2, respectively.28 The binding energy difference between the Fe 2p3/2 peak and the satellite peak is approximately 6 eV. The presence of this “shoulder” satellite peak and the binding energy difference of 6 eV are consistent with the results obtained by other researchers, and are clear evidence of the existence of Fe2+.29–31 The binding energy of the composite peak was slightly higher than that of Fe(II)-MOF, which proved the rapid transfer of electrons and the characteristic of the formation of a heterojunction.
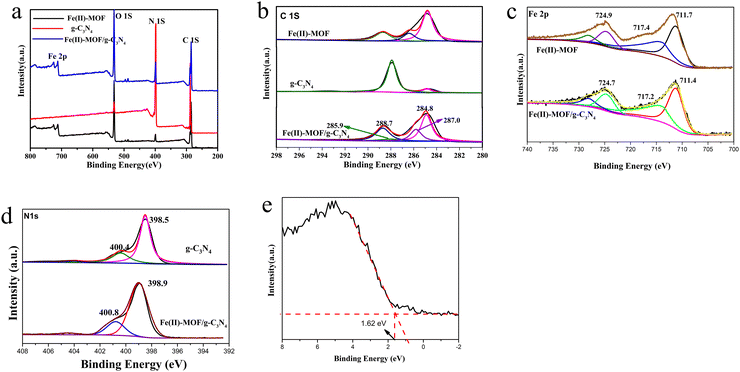 |
| Fig. 3 Full spectrum comparison of XPS of (a) Fe(II)-MOF, layered g-C3N4, and Fe(II)-MOF/g-C3N4; high resolution XPS of (b)C 1S (c) Fe(II) 2p, (d) N 1s and ()valence band (VB) XPS spectra of Fe(II)-MOF/g-C3N4. | |
Performance study of the photocatalytic benzene hydroxylation system
Effect of different catalysts on the hydroxylation of benzene to phenol.
The benzene hydroxylation properties of the photocatalysts Fe(II)-MOF, g-C3N4 and Fe(II)-MOF/g-C3N4 in pure acetonitrile solvents were compared with hydrogen peroxide as the oxidant. The experimental results are illustrated in Table 1. A lower phenol yield could be detected in the post-reaction products catalyzed by g-C3N4, and the yield was only 4.6%. Compared with g-C3N4, the catalyst Fe(II)-MOF could achieve a benzene conversion rate of 6.5%. Under the same conditions, when the composite Fe(II)-MOF/g-C3N4 was used as a catalyst, it exhibited higher photocatalytic performance than any single component (16.6% phenol yield and 98.8% phenol selectivity). Therefore, the best performance of Fe(II)-MOF/g-C3N4 was selected as the photocatalyst for benzene hydroxylation to make phenol in the following experimental exploration.
Table 1 Effect of different photocatalysts on benzene hydroxylation to produce phenol
Entry |
Yield (%) |
Conversion (%) |
Selectivity (%) |
Reaction conditions: catalyst (1: Fe(II)-MOF, 2: g-C3N4, 3: Fe(II)-MOF/g-C3N4, Fe(II)-MOF + g-C3N4 mixture) (1–3: 20 mg, 4: Fe(II)-MOF 14 mg, g-C3N4 6 mg), benzene (1.6 mL, 18.05 mmol), H2O2 (30 wt%) (0.6 mL), acetonitrile: 11 mL, trifluoroacetic acid: 0.1 g, trifluoroacetic acid: 0.1 g, time: 4 h and T: 333 K |
1 |
6.1 |
6.5 |
93.8 |
2 |
4.6 |
4.9 |
93.9 |
3 |
16.4 |
16.6 |
98.8 |
4 |
7.8 |
8.0 |
97.0 |
Effect of the catalyst amount
In order to investigate the effect of the amount of Fe(II)-MOF/g-C3N4 on the performance of benzene hydroxylation to phenol, catalyst samples of different amounts were added under the conditions of 1.6 mL substrate benzene, 11 mL acetonitrile and 0.6 mL oxidant H2O2. The photocatalytic performance of the system was tested at 60 °C for 4 h. As illustrated in Table 2, when there was no photocatalyst, direct hydroxylation was difficult for benzene under light irradiation, and almost no phenol was generated. With the increase of photocatalyst amount, the performance of benzene hydroxylation reaction was greatly improved, mainly because more catalysts could satisfy the quantity of Fe2+ required for the Fenton reaction, and more ·OH was generated to react with the benzene ring to produce phenol.32,33 When the catalyst amount was further increased, the yield of phenol decreased. Similar phenomena could be found in previous references. More catalyst powder in the photocatalytic process might cause the shielding effect, leading to the decrease of light utilization. Moreover, excessive production of hydroxyl radicals led to the self-quenching side reaction. The self-quenching reaction had a fast reaction rate, which was not conducive to the hydroxylation of the benzene reaction. Therefore, the optimal catalyst quality was 20 mg from performance and economic considerations.34–38
Table 2 The effect of the amount of catalyst on the catalytic benzene hydroxylation
Entry |
m (mg) |
Yield (%) |
Conversion (%) |
Selectivity (%) |
Reaction conditions: benzene (1.6 mL, 18.05 mmol), H2O2 (30 wt%) (0.6 mL), acetonitrile: 11 mL, trifluoroacetic acid: 0.1 g, t = 4 h and T = 333 K |
1 |
0 |
0.8 |
0.97 |
82.0 |
2 |
10 |
11.7 |
12.1 |
96.7 |
3 |
20 |
16.4 |
16.6 |
98.8 |
4 |
30 |
13.6 |
14.0 |
97.1 |
5 |
40 |
10.9 |
11.3 |
96.5 |
Effect of the amount of hydrogen peroxide
In the reaction system of photocatalytic benzene hydroxylation, the amount of oxidant (hydrogen peroxide) was another key factor which affected the activity of benzene hydroxylation to phenol. 20 mg of the Fe(II)-MOF/g-C3N4 catalyst, 1.6 mL of benzene and 0.1 g of co-catalyst trifluoroacetic acid were transferred to 11 mL of acetonitrile solvent with different volume ratios of benzene and hydrogen peroxide (8
:
1, 8
:
2, 8
:
3, 8
:
4 and 8
:
5). The effect of H2O2 addition to benzene hydroxylation to phenol was detected at 60 °C for 4 h. As illustrated in Table 3, the photocatalyst could harvest the optimal benzene hydroxylation to phenol, phenol yield and selectivity were 16.4% and 98.8%, respectively, under a volume ratio of 8
:
3. However, only a lower phenol yield was obtained at a higher volume ratio. This might be caused by the reasons that the higher H2O2 concentration to capture the hydroxyl radical was strong which reduced its amount in solution,39 and excessive oxidants would not only further convert phenol into by-products, but also lack economic and environmental protection in practical applications. Therefore, the optimal amount of hydrogen peroxide was 0.6 mL for this experiment.
Table 3 The effect of the added amount of H2O2 on the catalytic benzene hydroxylation
Entry |
n(H2O2)/n(benzene) |
Yield (%) |
Conversion (%) |
Selectivity (%) |
Reaction conditions: catalyst (20 mg), benzene (1.6 mL, 18.05 mmol), acetonitrile: 11 mL, trifluoroacetic acid: 0.1 g, time: 4 h and T: 333 K |
1 |
1/8 |
2.5 |
2.6 |
96.2 |
2 |
2/8 |
7.5 |
7.8 |
96.2 |
3 |
3/8 |
16.4 |
16.6 |
98.8 |
4 |
4/8 |
12.7 |
13.0 |
97.7 |
5 |
5/8 |
11.0 |
11.3 |
97.3 |
Effect of the trifluoroacetic acid amount
When acetonitrile was selected as the reaction solvent, studies had shown that the co-catalyst trifluoroacetic acid would help the system to form a uniform system between the composite and the reaction medium.40 To further investigate the extent of influence of trifluoroacetic acid on the photocatalytic reaction, the masses of the cocatalyst were 0 g, 0.05 g, 0.10 g, 0.15 g and 0.20 g without changing the other reaction conditions, and the results are illustrated in Table 4. When the cocatalyst was not used in the photocatalytic process, the conversion rate of benzene was only 7.3%. When the addition of trifluoroacetic acid was 0.1 g, the best benzene conversion rate (16.6%), phenol yield (16.4%) and selectivity (98.8%) could be obtained. When the amount of trifluoroacetic acid was further increased, the photocatalytic performance was reduced. Similar phenomena have been studied in the published references. The decrease of the hydroxylation of benzene at a higher amount of CF3COOH was apparently due to the decomposition of substantial amount of H2O2via the Fenton reaction to facilitate hydroxyl radical generation before attaining the maximum reaction rate. Indeed, the part of hydroxyl radicals which was involved in the hydroxylation of benzene was decreased, while the part of which led to the self-quenching side reaction was increased.41–45 Therefore, the optimum amount of trifluoroacetic acid was 0.1 g in this system.
Table 4 Effect of the amount of trifluoroacetic acid on the catalytic benzene hydroxylation
Entry |
Mass of TFA (g) |
Yield (%) |
Conversion (%) |
Selectivity (%) |
Reaction conditions: catalyst (20 mg), benzene (1.6 mL, 18.05 mmol), H2O2 (30 wt%) (0.6 mL), acetonitrile: 11 mL, time: 4 h and T: 333 K. |
1 |
0 |
7.0 |
7.3 |
95.9 |
2 |
0.05 |
10.5 |
10.8 |
97.2 |
3 |
0.10 |
16.4 |
16.6 |
98.8 |
4 |
0.15 |
13.0 |
13.3 |
97.7 |
5 |
0.20 |
9.2 |
9.5 |
96.8 |
Effects of the reaction time and temperature
The effect of different reaction times on the selective catalytic oxidation of benzene by Fe(II)-MOF/g-C3N4 is illustrated in Table 5. The experiment of hydroxylation reactions was taken at different times such as 1 h, 2 h, 3 h, 4 h and 5 h. The reaction time had an important impact on the yield of phenol, initially the yield increased with the increase of the reaction time, and then the yield decreased as the time continues to extend. When the reaction lasted for 4 h, the selectivity of phenol reached 98.9%, and the conversion of benzene also increased to 16.6%. And the yield of phenol decreased slightly with the extension of the reaction time, which might be caused by the oxidation of phenol.
Table 5 Effect of the reaction time on the catalytic benzene hydroxylation
Entry |
t (h) |
Yield (%) |
Conversion (%) |
Selectivity (%) |
Reaction conditions: catalyst (20 mg), benzene (1.6 mL, 18.05 mmol), H2O2 (30 wt%) (0.6 mL), acetonitrile: 11 mL, trifluoroacetic acid (0.1 g) and T = 333 K |
1 |
1 |
6.6 |
6.7 |
98.5 |
2 |
2 |
10.1 |
10.3 |
98.1 |
3 |
3 |
14.3 |
14.6 |
97.9 |
4 |
4 |
16.4 |
16.6 |
98.8 |
5 |
5 |
15.3 |
16.9 |
90.5 |
The influence of the reaction temperature was also investigated on the photocatalytic reaction, the experimental systems were placed at 50, 60 and 70 °C respectively. As illustrated in Table 6, initially, the yield increased with the increase of temperature, and then the yield decreased as the temperature continued to rise. The best benzene conversion and phenol selectivity was obtained at 60 °C. That might be because the higher reaction temperature was favorable for the generation of active radicals required during photocatalysis, which accelerated the photocatalytic reaction and increased the benzene conversion and yield of phenol to 16.6% and 16.4%, respectively. The photocatalytic performance of Fe(II)-MOF/g-C3N4 decreased when the reaction temperature reached 70 °C. The self-decomposition of hydrogen peroxide and the deep oxidation of phenol might be caused by the high temperature. And the reaction temperature increased close to the boiling point of benzene, it was easy to lead to the volatilization of the reactant.46 Therefore, the optimal time for benzene hydroxylation was 4 h and the optimal temperature was 60 °C.
Table 6 Effect of the reaction temperature and light on the benzene hydroxylation reaction
T (°C) |
Yield (%) |
Conversion (%) |
Selectivity (%) |
Reaction conditions: catalyst (20 mg), benzene (1.6 mL, 18.05 mmol), H2O2 (30 wt%) (0.6 mL), acetonitrile: 11 mL, trifluoroacetic acid: 0.1 g and t = 4 h, 60d: 60 °C in dark. |
50 |
11.8 |
12.2 |
96.7 |
60 |
16.4 |
16.6 |
98.8 |
70 |
14.3 |
14.8 |
96.6 |
60d |
11.1 |
13.7 |
81.1 |
Performance study of the photocatalytic cycle
Considering the economic benefits and the full utilization of materials, it was essential to further study the regeneration and reusability of photocatalysts. Therefore, the recycling ability of Fe(II)-MOF/g-C3N4 as a heterogeneous photocatalyst was investigated. After the photocatalytic reaction, the catalyst was collected by centrifugation and washed with ethanol the regenerated catalyst was used under the optimal experimental conditions. As shown in Fig. 4, the photocatalytic performance of Fe(II)-MOF/g-C3N4 maintained well after five cycles, and the benzene conversion rate was 14.7%, which was close to the yield with the fresh catalyst. This result proved that the photocatalyst was highly stable and could be recycled.
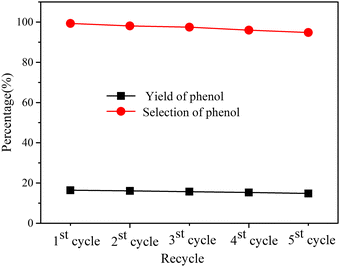 |
| Fig. 4 Recycling of Fe(II)-MOF/g-C3N4. Reaction conditions: catalyst (20 mg), benzene (1.6 mL, 18.05 mmol), H2O2 (30 wt%) (0.6 mL), acetonitrile: 11 mL, trifluoroacetic acid: 0.1 g, t = 4 h and T = 333 K. | |
A comparative study of Fe(II)-MOF/g-C3N4 MOFs and other photocatalysts previously reported for the photocatalytic hydroxylation of benzene to phenol was conducted, and the results are shown in Table 7. The phenol selectivity (99%) and yield (16.4%) obtained using the Fe(II)-MOF/g-C3N4 photocatalyst were higher than or comparable with those obtained using other photocatalysts.
Table 7 Comparison of photocatalytic activity of Fe(II)-MOF/g-C3N4 with other reported photocatalysts for the photocatalytic hydroxylation of benzene to phenol
Sample |
Amount (mg mL−1) |
Time (h) |
Yield (%) |
Ref. |
FeVO4@Al2O3 |
3.75 |
4 |
13.6 |
47
|
ZFO@C-2 |
5 |
— |
15.5 |
48
|
FePc |
6 |
4 |
15.2 |
49
|
UiO-66-NH2-SA-V |
1.5 |
4 |
15.3 |
50
|
Fe-g-C3N4/SBA-15 |
5.5 |
4 |
12.0 |
51
|
Fe(II)-MOF/g-C3N4 |
1.5 |
4 |
16. 4 |
This work |
Enhancement of photocatalytic performance and exploration of the process mechanism
Factors for improving photocatalytic performance.
The light absorption characteristics of the sample could be understood by using the solid UV-visible diffuse reflection test, so as to further study the band structure of photocatalyst samples. As shown in Fig. 5, a single Fe(II)-MOF material had a wide light absorption range of absorbing light from the UV region to the visible region, where the wide absorption band displayed within a wavelength of 400 nm is concerned with the π–π* transition in the NH2-BDC ligand and the band gap is 1.18 eV.52 The light absorption capacity of the material in the visible light region was attributed to the existence of the Fe–O cluster of the iron-metal–organic framework material itself.53 A single g-C3N4 with absorption range from 200 nm to 450 nm had poor response to visible light and the band gap is 2.54 eV. Compared with Fe(II)-MOF, highly similar absorption spectra appeared in the composite Fe(II)-MOF/g-C3N4, and its optical absorption intensity increased significantly in the wavelength range of 200–620 nm and the band gap is 1.56 eV, which proved that the composite had better optical absorption capacity.
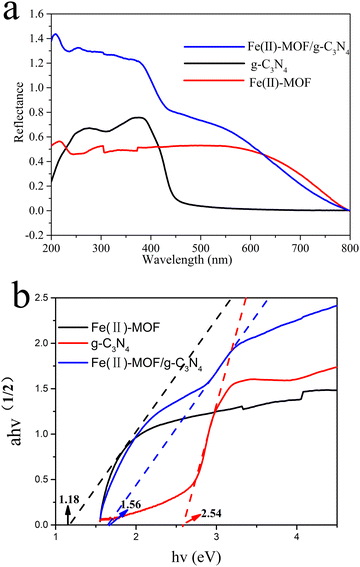 |
| Fig. 5 Solid UV diffuse reflection of Fe (II)-MOF, layered g-C3N4, and Fe(II)-MOF/g-C3N4 (a) and band gap diagram of layered g-C3N4, and Fe(II)-MOF/g-C3N4 (b). | |
The high photogenic-hole charge separation effect played an important role in photocatalytic phenol production. Therefore, the photoluminescence behavior of Fe(II)-MOF, g-C3N4, and Fe(II)-MOF/g-C3N4 were determined at an emission wavelength of 375 nm. Due to the fluorescence quenching behavior of 2-aminoterephthalic acid and Fe2+, the fluorescence intensity of the single Fe(II)-MOF material was close to the baseline (Fig. 6(a)). However, when the Fe(II)-MOF combined with the g-C3N4, Fe(II)-MOF/g-C3N4 showed a lower fluorescence intensity than the g-C3N4 at a wavelength of 440 nm. These results indicated that Fe(II)-MOF/g-C3N4 had a higher photogenerated hole–charge separation efficiency.
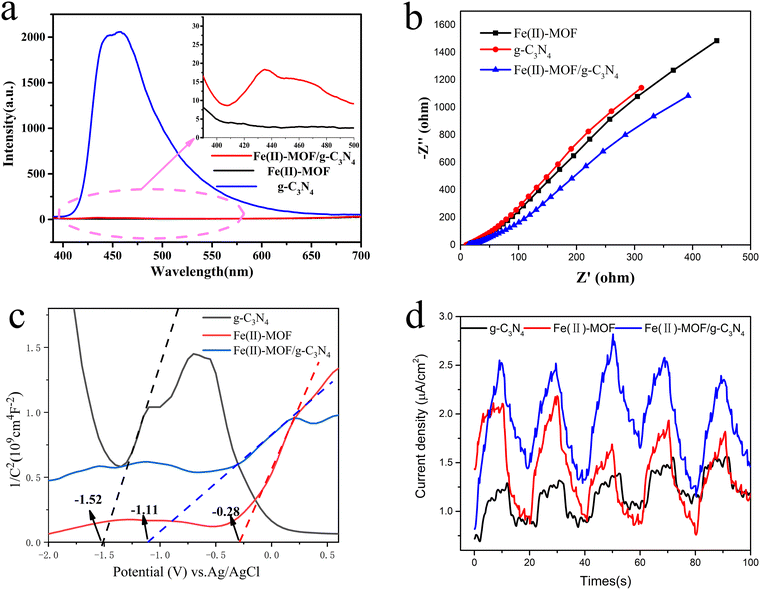 |
| Fig. 6 Photoluminescence profiles (a) of Fe(II)-MOF, layered g-C3N4, and Fe(II)-MOF/g-C3N4, electrochemical impedance profiles (b) and Mot–Schottky diagram (c) of Fe(II)-MOF and Fe(II)-MOF/g-C3N4. Reaction conditions: catalyst (20 mg), benzene (1.6 mL, 18.05 mmol), H2O2 (30 wt%) (0.6 mL), acetonitrile: 11 mL, trifluoroacetic acid: 0.1 g, t = 4 h and T = 333 K. | |
The electrochemical impedance was also employed to further observe the effect of introducing g-C3N4 on photogenic charge–hole on recombination. The EIS Nyquist curves of g-C3N4, Fe(II)-MOF and Fe(II)-MOF/g-C3N4 electrodes were recorded under light avoidance conditions (Fig. 6(b)). The smaller the radius of the Nyquist circle, the smaller the transfer resistance, which indicated the lower the recombination rate of photogenerated carriers. Therefore, the separation efficiency of the electron–hole pair of Fe(II)-MOF/g-C3N4 was significantly higher than that of Fe(II)-MOF and g-C3N4.
In order to explore the influence of g-C3N4 introduction on the band structure, Mott–Schottky curves of g-C3N4, Fe(II)-MOF and Fe(II)-MOF/g-C3N4 catalysts were obtained by the Mott–Schottky measurement method (Fig. 6(c)). The curves reveal that the tangent line presents a positive slope at a frequency of 1000 Hz, which indicated that g-C3N4, Fe(II)-MOF and Fe(II)-MOF/g-C3N4 were all typical N-type semiconductors. The optical properties of Fe(II)-MOF/g-C3N4 were measured by using UV-vis absorption spectra (Fig. 5(a)), and the intrinsic optical bandgap energy (Eg) was calculated according to the Kubelka–Munk function (Fig. 5(b)). Then the Eg of Fe(II)-MOF/g-C3N4 was determined to be 1.56 eV by transforming the plots of absorption spectra. The measured flat band potential of Fe(II)-MOF/g-C3N4 was obtained to be −1.11 eV vs. RHE by fitting the Mott–Schottky plots in Fig. 6(c). As shown in Fig. 6(e), the valence band (VB) XPS spectra of Fe(II)-MOF/g-C3N4 were recorded and their VB was calculated to be 0.51 eV. Furthermore, the conduction band (CB) positions of samples were calculated to be −1.05 eV vs. RHE based on the ECB = EVB − Eg.54,55 In conclusion, as the composite rate of photo charged holes could be effectively suppressed, the photocarrier life could be extended, and the photoelectron could be effectively transferred to produce active free radicals, which could be applied for the photocatalytic benzene hydroxylation reaction.
Generally, the photocatalytic activity was reflected by the photocurrent intensity, the better photocatalytic activity usually resulted by the higher photocurrent intensity.56 Therefore, the separation efficiency of photoinduced charges in the photochemical reactions was characterized by the technique of the transient photocurrent measurement. As shown in Fig. 6(d), the composite Fe(II)-MOF/g-C3N4 possessed a distinctly higher photocurrent density than UiO-66-NH2 under the same conditions. This result revealed that the recombination of photogenerated carriers was inhibited after it was compounded with g-C3N4.
Photocatalytic mechanism
Free radical capture detects the active substance.
The main active substances involved in the photocatalytic benzene hydroxylation process are h+, e−, and ·OH.57 In order to clarify the mechanism of the Fe(II)-MOF/g-C3N4 material in the process of benzene hydroxylation to produce phenol, the active substance that played a major role in the reaction were determined by free radical trapping experiments under the optimal conditions. Isopropanol (IPA), potassium dichromate and disodium ethylenediaminetetraacetate (EDTA-2Na) were employed as scavengers of hydroxyl radical (·OH), electron (e−) and hole (h+), respectively. As shown in Fig. 7, it was obvious that the addition of three capture agents all had an effect on the yield of phenol, and the addition of IPA greatly inhibited the photocatalytic process. This indicated that the phenol production process was mainly driven by the participation of hydroxyl radicals.
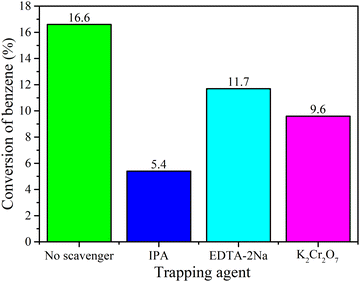 |
| Fig. 7 Free-radical trapping experiments. | |
Basic reaction mechanism
According to the reported work and the experimental results, a possible photocatalytic mechanism of benzene hydroxylation to phenol was proposed in Fig. 8. In this process, visible light irradiation promotes the generation of the intermediate free radicals, contributing to the catalytic performance, and then, Fe(II)-MOF/g-C3N4 reacts with H2O2 to produce ·OH based on the Fenton reaction. And H2O2 can also be trapped by Fe3+ on the surface of the catalyst, forming Fe2+ and completing the cycle of Fe2+/Fe3+. And the generated OH− could be oxidized by h+ to ·OH. Finally, the produced ·OH can attack the benzene molecules to form hydroxyl cyclohexadienyl radical. After losing a proton, phenol was generated.58–63
Fe(II)-system + H2O2 → Fe(III)-system + ·OH + OH− |
Fe(III)-system + H2O2 → Fe(II)-system + ·O2H + H+ |
C6H6 + ·OH + h+ → C6H6O + H+ |
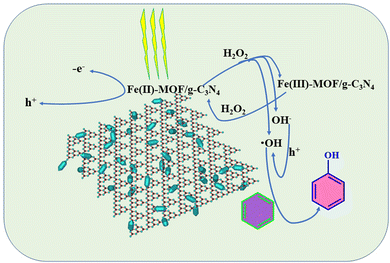 |
| Fig. 8 Schematic diagram of the photocatalytic mechanism. | |
Conclusions
In summary, Fe(II)-MOF/g-C3N4 was successfully prepared by the recombination of the Fe(II)-MOF and g-C3N4via an in situ composite technique. The catalyst could achieve direct hydroxylation of benzene under optimal conditions, with a yield and selectivity of 16.4% and 98.9%, respectively. The catalyst could still maintain high photocatalytic activity after 5 cycles. Therefore, due to the efficient catalytic activity and regeneration performance, the heterogeneous photocatalyst Fe(II)-MOF/g-C3N4 showed great potential in the field of benzene hydroxylation to phenol and provided new enlightenment for this field.
Conflicts of interest
There are no conflicts to declare.
Acknowledgements
This project was financially supported by the 2018 Funding Plan for Young Core Teachers of Zhongyuan University of Technology, the Discipline Strength Improvement Program of Zhongyuan University of Technology: Program for Cultivating Disciplinary Young Master Supervisor (SD202204), and the Basic Scientific Research Program of Zhongyuan University of Technology (K2018QN018).
Notes and references
- Y. Elkasabi, K. Jones and C. A. Mullen,
et al., Spinning band distillation of biomass pyrolysis oil phenolics to produce pure phenol, Sep. Purif. Technol., 2023, 123603 CrossRef CAS
.
- Z. H. Yu, Y. L. Gan and J. Xu,
et al., Direct catalytic hydroxylation of benzene to phenol catalyzed by FeCl3 supported on exfoliated graphitic carbon nitride, Catal. Lett., 2020, 150, 301–311 CrossRef CAS
.
- W. Han, W. Xiang and J. Shi,
et al., Recent Advances in the Heterogeneous Photocatalytic Hydroxylation of Benzene to Phenol, Molecules, 2022, 27(17), 5457 CrossRef CAS PubMed
.
- X. Sun, S. Jiang and H. Huang,
et al., Solar energy catalysis, Angew. Chem., Int. Ed., 2022, 134(29), e202204880 Search PubMed
.
- S. Li, F. Wu and R. Lin,
et al., Enabling photocatalytic hydrogen production over Fe-based MOFs by refining band structure with dye sensitization, Chem. Eng. J., 2022, 429, 132217 CrossRef CAS
.
- D. Wang and Z. Li, Iron-based metal–organic frameworks (MOFs) for visible-light-induced photocatalysis, Res. Chem. Intermed., 2017, 43, 5169–5186 CrossRef CAS
.
- X. Zhang, X. Ma and Y. Ye,
et al., Enhanced photocatalytic hydrogen evolution with a Mixed-Valence iron Metal–Organic framework, Chem. Eng. J., 2023, 456, 140939 CrossRef CAS
.
- D. Wang, M. Wang and Z. Li, Fe-based metal–organic frameworks for highly selective photocatalytic benzene hydroxylation to phenol, ACS Catal., 2015, 5(11), 6852–6857 CrossRef CAS
.
- T. N. Tu, H. T. T. Nguyen and H. T. D. Nguyen,
et al., A new iron-based metal–organic framework with enhancing catalysis activity for benzene hydroxylation, RSC Adv., 2019, 9(29), 16784–16789 RSC
.
- T. Yang, D. Yu and D. Wang,
et al., Accelerating Fe(III)/Fe(II) cycle via Fe(II) substitution for enhancing Fenton-like performance of Fe-MOFs, Appl. Catal., B, 2021, 286, 119859 CrossRef CAS
.
- D. Masih, Y. Ma and S. Rohani, Graphitic C3N4 based noble-metal-free photocatalyst systems: a review, Appl. Catal., B, 2017, 206, 556–588 CrossRef CAS
.
- S. Verma, R. B. Nasir Baig and M. N. Nadagouda,
et al., Hydroxylation of Benzene via C–H activation using bimetallic CuAg@g-C3N4, ACS Sustainable Chem. Eng., 2017, 5(5), 3637–3640 CrossRef CAS PubMed
.
- S. Su, Z. Xing and S. Zhang,
et al., Ultrathin mesoporous g-C3N4/NH2-MIL-101(Fe) octahedron heterojunctions as efficient photo-Fenton-like system for enhanced photo-thermal effect and promoted visible-light-driven photocatalytic performance, Appl. Surf. Sci., 2021, 537, 147890 CrossRef CAS
.
- J. Fu, J. Yu and C. Jiang,
et al., g-C3N4-Based heterostructured photocatalysts. Advanced Energy, Materials, 2018, 8(3), 1701503 Search PubMed
.
- Y. Qi, J. Xu and Y. Fu,
et al., Metal–Organic Framework Templated Synthesis ofg-C3N4/Fe2O3@FeP Composites for Enhanced Hydrogen Production, ChemCatChem, 2019, 11(15), 3465–3473 CrossRef CAS
.
- X. Xu, Y. Chen and Y. Zhang,
et al., Highly stable and biocompatible hyaluronic acid-rehabilitated nanoscale MOF-Fe2+ induced ferroptosis in breast cancer cells, J. Mater. Chem. B, 2020, 8(39), 9129–9138 RSC
.
- D. Huang, Z. Li and G. Zeng,
et al., Megamerger in photocatalytic field: 2D g-C3N4 nanosheets serve as support of 0D nanomaterials for improving photocatalytic performance, Appl. Catal., B, 2019, 240, 153–173 CrossRef CAS
.
- F. Wei, D. Chen and Z. Liang,
et al., Preparation of Fe-MOFs by microwave-assisted ball milling for reducing Cr(VI) in wastewater, Dalton Trans., 2017, 46(47), 16525–16531 RSC
.
- S. B. Vuggili, U. K. Gaur and M. Sharma, 2D/2D facial interaction of nitrogen-doped g-C3N4/In2S3 nanosheets for high performance by visible-light-induced photocatalysis, J. Alloys Compd., 2022, 902, 163757 CrossRef CAS
.
- X. Zhang, X. Song and J. Wang,
et al., CO2 gasification of Yangchangwan coal catalyzed by iron-based waste
catalyst from indirect coal-liquefaction plant, Fuel, 2021, 285, 119228 CrossRef CAS
.
- A. Rm, B. Mm and A. Apgp, Synthesis of g-C3N4/ZnO heterostructure photocatalyst for enhanced visible degradation of organic dye – ScienceDirect, Optik, 2021, 229, 165548 CrossRef
.
- Y. O. Ibrahim and M. A. Gondal, Visible-light-driven photocatalytic performance of a Z-scheme based TiO2/WO3/g-C3N4ternary heterojunctions, Mol. Catal., 2021, 505, 111494 CrossRef CAS
.
- M. Chen, W. Chang and Y. Shi,
et al., Design of highly efficient g-C3N4-based metal monoatom catalysts by two extra-NM1 atoms: density functional theory simulations, Phys. Chem. Chem. Phys., 2021, 23(19), 11472–11478 RSC
.
- P. Xiao, Y. Wang and R. Osuga,
et al., One-pot synthesis of highly active Fe-containing MWW zeolite catalyst: Elucidation of Fe species and its impact on catalytic performance, Adv. Powder Technol., 2021, 32(4), 1070–1080 CrossRef CAS
.
- T. Zhao, C. Cheng and D. Wang,
et al., Preparation of a bimetallic NiFe-MOF on nickel foam as a highly efficient electrocatalyst for oxygen evolution reaction, ChemistrySelect, 2021, 6(6), 1320–1327 CrossRef CAS
.
- J. R. Zhang, Y. Ma and S. Y. Wang,
et al., Accurate K-edge X-ray photoelectron and absorption spectra of g-C3N4 nanosheets by first-principles simulations and reinterpretations, Phys. Chem. Chem. Phys., 2019, 21(41), 22819–22830 RSC
.
- M. Yu, H. Liang and R. Zhan,
et al., Sm-doped g-C3N4/Ti3C2 MXene heterojunction for visible-light photocatalytic degradation of ciprofloxacin, Chin. Chem. Lett., 2021, 32(7), 2155–2158 CrossRef CAS
.
- W. B. Mi, E. Y. Jiang and H. L. Bai, Fe3+/Fe2+ ratio controlled magnetic and electrical transport properties of polycrystalline Fe3(1−δ)O4 films, J. Phys. D: Appl. Phys., 2009, 42(10), 105007 CrossRef
.
- T. Yamashita and P. Hayes, Analysis of XPS spectra of Fe2+ and Fe3+ ions in oxide materials, Appl. Surf. Sci., 2008, 254(8), 2441–2449 CrossRef CAS
.
- S. J. Roosendaal, B. Van Asselen and J. W. Elsenaar,
et al., The oxidation state of Fe (100) after initial oxidation in O2, Surf. Sci., 1999, 442(3), 329–337 CrossRef CAS
.
- P. C. J. Graat and M. A. J. Somers, Simultaneous determination of composition and thickness of thin iron-oxide films from XPS Fe 2p spectra, Appl. Surf. Sci., 1996, 100, 36–40 CrossRef
.
- X. Wei, N. Zhu and X. Huang,
et al., Efficient degradation of sodium diclofenac via heterogeneous Fenton reaction boosted by Pd/Fe@Fe3O4 nanoparticles derived from bio-recovered palladium, J. Environ. Manage., 2020, 260, 110072 CrossRef CAS PubMed
.
- P. P. Xu, L. Zhang and X. Jia,
et al., A novel heterogeneous catalyst NH2-MIL-88/PMo10V2 for the photocatalytic activity enhancement of benzene hydroxylation, Catal. Sci. Technol., 2021, 11(19), 6507–6515 RSC
.
- Y. Shen, J. Yang and C. Ma,
et al., Removal of VOCs from wood by introducing activators to form hydroxyl radicals in situ generation platform, J. Environ. Chem. Eng., 2022, 10(6), 108551 CrossRef CAS
.
- Q. Zhang, M. He and X. Zhang,
et al., Tumor microenvironment Activated Chemodynamic–Photodynamic Therapy by multistage self-Assembly engineered protein nanomedicine, Adv. Funct. Mater., 2022, 32(17), 2112251 CrossRef CAS
.
- B. T. Zhang, Z. Yan and Y. Liu,
et al., Nanoconfinement in advanced oxidation processes, Crit. Rev. Environ. Sci. Technol., 2023, 53(12), 1197–1228 CrossRef CAS
.
- Y. Zhang, H. Wang and T. Zhang,
et al., Improving permselectivity of the polyamide reverse osmosis membrane by a controlled-release sulfate radical modification, Sep. Purif. Technol., 2023, 124067 CrossRef CAS
.
- D. Xu, Q. Duan and Y. Hui,
et al., Photodynamic therapy based on porphyrin-based metal–organic frameworks, J. Mater. Chem. B, 2023 10.1039/D2TB02789E
.
- Z. He, Y. Liu and Y. Xiao, Optimization of Fenton pretreatment for 2-chlorophenol solution, J. Cent. South Univ., 2013, 20(10), 2791–2795 CrossRef CAS
.
- G. Grigoropoulou, J. H. Clark and J. A. Elings, Recent developments on the epoxidation of alkenes using hydrogen peroxide as an oxidant, Green Chem., 2003, 5(1), 1–7 RSC
.
- M. V. Kirillova, Y. N. Kozlov and L. S. Shul’pina,
et al., Remarkably fast oxidation of alkanes by hydrogen peroxide catalyzed by a tetracopper(II) triethanolaminate complex: Promoting effects of acid co-catalysts and water, kinetic and mechanistic features, J. Catal., 2009, 268(1), 26–38 CrossRef CAS
.
- D. Bianchi, R. Bortolo and R. Tassinari,
et al., A novel iron-based catalyst for the biphasic oxidation of benzene to phenol with hydrogen peroxide, Angew. Chem., Int. Ed., 2000, 112(23), 4491–4493 CrossRef
.
- L. Carneiro and A. R. Silva, Selective direct hydroxylation of benzene to phenol with hydrogen peroxide by iron and vanadyl based homogeneous and heterogeneous catalysts, Catal. Sci. Technol., 2016, 6(22), 8166–8176 RSC
.
- K. Jaiswal, M. Mahanta and M. De, Nanomaterials in photocatalysed organic transformations: development, prospects and challenges, Chem. Commun., 2023, 59, 5987–6003 RSC
.
- A. Conde, M. M. Diaz-Requejo and P. J. Pérez, Direct, copper-catalyzed oxidation of aromatic C–H bonds with hydrogen peroxide under acid-free conditions, Chem. Commun., 2011, 47(28), 8154–8156 RSC
.
- S. Farahmand, M. Ghiaci and S. Asghari, Oxo-vanadium(IV) phthalocyanine implanted onto the modified SBA-15 as a catalyst for direct hydroxylation of benzene to phenol in acetonitrile-water medium: A kinetic study, Chem. Eng. Sci., 2021, 232, 116331 CrossRef CAS
.
- H. Liang, Z. Fang and D. Wei,
et al., Improved durability and activity of FeVO4 toward photocatalytic benzene hydroxylation by atomic layer deposited Al2O3, ChemistrySelect, 2022, 7(29), e202201044 CAS
.
- B. Yang, S. Zhang and Y. Gao,
et al., Unique functionalities of carbon shells coating on ZnFe2O4 for enhanced photocatalytic hydroxylation of benzene to phenol, Appl. Catal., B, 2022, 304, 120999 CrossRef CAS
.
- S. Asghari, S. Farahmand and J. S. Razavizadeh,
et al., One-step photocatalytic benzene hydroxylation over iron(II) phthalocyanine: A new application for an old catalyst, J. Photochem. Photobiol., A, 2020, 392, 112412 CrossRef CAS
.
- Y. Fang, L. Zhang and Q. Zhao,
et al., Highly selective visible-light photocatalytic benzene hydroxylation to phenol using a new heterogeneous photocatalyst UiO-66-NH2-SA-V, Catal. Lett., 2019, 149, 2408–2414 CrossRef CAS
.
- X. Chen, J. Zhang and X. Fu,
et al., Fe-g-C3N4-catalyzed oxidation of benzene to phenol using hydrogen peroxide and visible light, J. Am. Chem. Soc., 2009, 131(33), 11658–11659 CrossRef CAS PubMed
.
- T. Van Tran, V. H. Nguyen and L. X. Nong,
et al., Hexagonal Fe-based MIL-88B nanocrystals with NH2 functional groups accelerating oxytetracycline capture via hydrogen bonding, Surf. Interfaces, 2020, 20, 100605 CrossRef
.
- L. Sang, Y. Cheng and R. Yang,
et al., Polyphosphazene-wrapped Fe–MOF for improving flame retardancy and smoke suppression of epoxy resins, J. Therm. Anal. Calorim., 2021, 144, 51–59 CrossRef CAS
.
- X. Dang, R. Yang and Z. Wang,
et al., Efficient visible-light activation of molecular oxygen to produce hydrogen peroxide using P doped g-C3N4 hollow spheres, J. Mater. Chem. A, 2020, 8(43), 22720–22727 RSC
.
- S. Zhao and X. Zhao, Insights into the role of singlet oxygen in the photocatalytic hydrogen peroxide production over polyoxometalates-derived metal oxides incorporated into graphitic carbon nitride framework, Appl. Catal., B, 2019, 250, 408–418 CrossRef CAS
.
- L. Li, J. Wu and B. Liu,
et al., NiS sheets modified CdS/reduced graphene oxide composite for efficient visible light photocatalytic hydrogen evolution, Catal. Today, 2018, 315, 110–116 CrossRef CAS
.
- B. Han, X. Li and Z. Zhang,
et al., A novel strategy to research the mechanism of rutile TiO2 with excellent photocatalytic performance, Nanotechnology, 2021, 33(3), 035704 CrossRef PubMed
.
- F. Zhu, S. Zhou and M. Sun,
et al., Heterogeneous activation of persulfate by Mg doped Ni (OH)2 for efficient degradation of phenol, Chemosphere, 2021, 131647 Search PubMed
.
- S. J. Feng, B. Yue and Y. Wang,
et al., Hydroxylation of benzene over V-HMS catalysts with the addition of Fe as the second metal component, Acta Phys.-Chim. Sin., 2011, 27(12), 2881–2886 CAS
.
- Z. Yang, Z. Wang and J. Wang,
et al., Facet-Dependent Activation of Oxalic Acid over Magnetic Recyclable Fe3S4 for Efficient Pollutant Removal under Visible Light Irradiation: Enhanced Catalytic Activity, DFT Calculations, and Mechanism Insight, Environ. Sci. Technol., 2022, 56(24), 18008–18017 CrossRef CAS PubMed
.
- J. Li, C. Xiao and K. Wang,
et al., Enhanced generation of reactive oxygen species under visible light irradiation by adjusting the exposed facet of FeWO4 nanosheets to activate oxalic acid for organic pollutant removal and Cr(VI) reduction, Environ. Sci. Technol., 2019, 53(18), 11023–11030 CrossRef CAS PubMed
.
- Z. Wang, L. Jiang and K. Wang,
et al., Novel AgI/BiSbO4 heterojunction for efficient photocatalytic degradation of organic pollutants under visible light: interfacial electron transfer pathway, DFT calculation and degradation mechanism study, J. Hazard. Mater., 2021, 410, 124948 CrossRef CAS PubMed
.
- T. Guo, L. Jiang and K. Wang,
et al., Efficient persulfate activation by hematite nanocrystals for degradation of organic pollutants under visible light irradiation: Facet-dependent catalytic performance and degradation mechanism, Appl. Catal., B, 2021, 286, 119883 CrossRef CAS
.
|
This journal is © The Royal Society of Chemistry 2023 |