DOI:
10.1039/D2MA01003H
(Review Article)
Mater. Adv., 2023,
4, 389-407
A focused review on structures and ionic conduction mechanisms in inorganic solid-state proton and hydride anion conductors
Received
27th October 2022
, Accepted 21st November 2022
First published on 28th November 2022
Abstract
Solid-state proton and hydride anion conductors are an important family of materials as electrolytes for solid state electrochemical cells such as fuel cells, batteries, sensors, and gas separation membranes. Searching for new proton and hydride-anion conductors has been an active research area for many decades. The focus of this article is on reviewing the types and mechanisms of each proton/hydride-anion conductor developed and their pros and cons. This review starts off with the most studied and most promising perovskite structured oxides as proton conductors, followed by other types of perovskite-related structures such as the Ruddlesden–Popper phase, pyrochlores and rare earth orthoniobates/orthotantalates. This review then moves to solid polyanionic compounds as proton conductors, including sulfates, nitrates, and phosphates, which is followed by hydrates and nanocomposites. This review finally discusses the types and conduction mechanisms of new hydride-anion conductors that emerged recently.
1. Introduction
Many solids exhibiting fast proton (H+) and hydride ion (H−) conduction have been reported for decades. It is generally understandable that H+ and H− move fast in solids due to their size and charge.1 Like any ionic conductors, H+/H− conductors can be used as an electrolyte in electrochemical cells for H2 conversion and storage; the latter is a vital clean technology to combat climate crisis and build a carbon pollution-free economy. In general, H+/H− conductors are found in both organic and inorganic materials. Organic materials, such as Nafion (perfluorosulfonic acid polymer), show high proton conductivity at low temperatures (<90 °C) under hydrated conditions, while inorganic materials, on the other hand, show H+/H− conduction in a higher and wider temperature range. In this review, we will focus primarily on the inorganic H+/H− conductors. Despite many existing reviews on H+ conducting materials in the literature, they are mostly focused on material properties and applications in energy conversion and storage.2–5 A review encompassing both H+/H− conductors with a focus on structure and ionic conduction mechanisms is still lacking. In this article, we aim to fill this gap by reviewing recent progress of inorganic solid-state fast H+/H− conductors in the perspectives of synthesis, structure and phase transformations, conductivity, and conduction mechanisms.
The inorganic proton conductors reviewed in this article include solid oxides, solid polyanionic salts, and hydrates. The solid-oxide family includes perovskites, Ruddlesden–Popper intergrowths, and ortho-niobates/ortho-tantalates; the solid polyanionic salt family includes inorganic acids, sulphates, and phosphates/pyrophosphates; the hydrate family includes hygroscopic mesoporous materials. Compared to the well-studied proton conductors, hydride anion (H−) conductors are under studied. Although H− has a larger size than H+, it is still small enough for fast ion transport and is a strong reducing agent that could find unique applications in energy storage and conversion devices. For these reasons, there has been growing interest in H− conductors in recent years. This class of materials includes hydrides, nitride-hydrides, perovskite oxyhydrides, and hydroflurides. In the following, we review the key aspects of these H+/H− conductors.
2. Solid oxide proton conductors
2.1 Perovskite oxides
One of the most studied proton conductors is perovskite oxides. The typical chemical formula of the perovskite oxides is ABO3, where A and B denote two different cations, see Fig. 1. A typical ABO3 structure consists of a large 12-coordinated A cation (A = Ca, Sr, Ba, La, and Gd), and a small 6-coordinated B cation (B = Nb, W, Ce, Zr, Ti, Mn, Fe, Co, and Ga). The ideal ABO3 structure is primitive cubic, but many ABO3 exhibit a lower symmetry (e.g., hexagonal and orthorhombic) with slightly distorted unit cells because of the ionic size effect as described by the Goldschmidt's tolerance factor. Based on the types of A and B cations, ABO3 can be an ionic conductor, semiconductor, and electronic conductor, finding applications in electrochemical devices such as solid oxide fuel cells and catalytic membrane reactors.6–12
 |
| Fig. 1 The ideal structure of ABO3.13 | |
For a perovskite oxide to be a proton conductor, a proton in the form of
on the O2−-sub-lattice can be generated by a hydration process under humid conditions or a hydrogenation process under reducing conditions as illustrated by the following Kröger–Vink defect reactions:
|  | (1) |
|  | (2) |
Clearly, the concentration of OH− is favored by higher partial pressure of H2O (pH2O), oxygen vacancy (
) concentration, and lattice oxygen (
) concentration. Since the reactions are exothermic, e.g., the enthalpy (ΔH°) of the reaction (1) for BaCe0.9Y0.1O3−δ and BaZr0.8Y0.2O3−δ are −163.3 and −79.5 kJ mol−1, respectively,4 the proton concentration (
) decreases with temperature.3,14,15
Two proton conducting mechanisms have been proposed to describe the H+ transport in the perovskite-type oxides:15,16 (1) the Grotthuss mechanism, Fig. 2(a), where H+ migrates via reorientation of H+ and the formation and cleavage of H-bonds with the adjacent lattice O, and (2) the vehicle mechanism, Fig. 2(b), in which H+ is first bonded with O2− to form OH− (or (
) by Kröger–Vink notations) and then diffuses through
. There has been increasing evidence in recent years showing that both Grotthuss and vehicular mechanisms are involved in the H+ transport in perovskite oxides.
 |
| Fig. 2 Schematics of two H+ conducting mechanisms in a BaZrO3-based perovskite oxide: (a) the Grotthuss mechanism and (b) the vehicle mechanism.17 | |
Due to the presence of
, perovskite proton conductors can also be good oxide-ion conductors, depending on the conditions. For example, H+ conductivity dominates under high pH2O, low pO2, and low temperature, whereas O2− conductivity is favored by dry and high temperatures.18 The magnitude of proton conductivity also depends on the material composition. For example, Ba-containing perovskites such as BaCeO3 and BaZrO3 are among the best in all proton conductors studied so far.
The first reported perovskite H+ conductor was doped SrCeO3.19,20 Since then, doped BaZrO3 and BaCeO3 have become the most studied proton conductors due to their higher conductivity.10,21–26 In general, doped BaCeO3 exhibit a higher conductivity (e.g. ∼2 × 10−2 S cm−1 at 600 °C) than doped BaZrO3,27,28 but their chemical stability in H2O and CO2 is generally poorer. To achieve a good balance among chemical stability, ionic conductivity, and mechanical property, “solid solution” mixing between BaCeO3 and BaZrO3 is a widely adopted strategy. However, to further increase the degree of hydration and thus proton conductivity, the B site needs to be partially doped by rare earth cations such as Y3+, Yb3+, Gd3+, and Sm3+. For example, Y- and Zr-doped BaCeO3 (BaCe1−x−yZrxYyO3−δ, BCZY) has been one of the most studied systems for H+ conductors with high conductivity and chemical stability under CO2, H2O, or H2S-containing atmosphere.29,30 Similarly, Y-, Zr- and Yb-triple-doped BaCeZrO3 with compositions of BaZr0.1Ce0.7Y0.2−xYbxO3−δ (x = 0 to 0.2, BZCYYb) has also been reported with good ionic conductivity and chemical stability.23Fig. 3 shows the comparison of ionic conductivity in 3%H2O moistened O2 among BZCYYb, YSZ (Y2O3-stabilized ZrO2), GDC (Gd2O3-doped CeO2) and BZCY; it is evident that BZCYYb shows the highest conductivity below 750 °C.
 |
| Fig. 3 Ionic conductivities of BZCYYb, BZCY, GDC, and YSZ as measured at 400° to 750 °C in wet oxygen (with ∼3 vol % H2O).23 | |
The fuel cell performance with BZCYYb as an electrolyte was further demonstrated with a high faradaic efficiency (90–98%) and >97% overall electric-to-H2 energy conversion efficiency (based on the lower heating value of H2) at a current density of 1 A
cm−2.22 With a co-fed CO2 and H2O, the same cell was shown with a round-trip efficiency (electricity-to-H2-to-electricity) >75% and stable operation at a degradation rate of <30
mV per 1000
h.
A similar composition, BaZr0.4Ce0.4Y0.1Yb0.1O3 (BZCYYb4411), has been tested as a H+-conducting electrolyte in a solid oxide cell setting with a mixed conductor PrBa0.5Sr0.5Co1.5Fe0.5O5+δ (PBSCF) as the air electrode, and a composite of Ni–BZCYYb4411 as the fuel electrode, see Fig. 4(a) and (b).31 The low-temperature solid oxide fuel cell (SOFC) performance, see Fig. 4(c), is outstanding and the long-term steam electrolysis performance under 500 °C and constant 1.3 V, see Fig. 4(d), is also high and stable cells, further demonstrating the potential of BaCeO3–BaZrO3-based materials as a good H+ conductor.
 |
| Fig. 4 (a) Cross-sectional view of 15 μm thick, dense electrolyte BZCYYb4411 and a 15 μm thick, porous PBSC air electrode supported on a porous fuel electrode. (b) Microstructure of the PBSCF air electrode. (c) V–I and P–I curves under fuel cell modes. (d) Long-term steam electrolysis performance at 550 °C and a fixed 1.3 V.31 | |
Despite the broad interest in perovskite H+ conductors, practical applications of these conductors in large and thin film formats have been severely limited due to the poor mechanical strength, high reactivity with adjacent components, and difficult processing conditions. Therefore, how to implement the BaCeO3–BaZrO3-based H+-conductors into solid oxide cell (SOC) stacks remain an engineering challenge.
2.2 Perovskite proton conductors mixed with molten carbonate
To overcome the chemical stability issues and manufacturing difficulties associated with the BZY H+-conductor, a mixed proton and carbonate ion conductor (MPCC) comprising of a pre-sintered proton conductor BaZr0.8Y0.2O3−d (BZY) skeleton infiltrated with molten carbonate (MC) phase was reported.32 The H+-conductivity of MPCC reached 0.33 S cm−1 at 600 °C in 3% H2O–air and and 0.38 S cm−1 in 3%H2O–H2, which is roughly two orders of magnitude higher than pure BZY, see Fig. 5(a). With the aid of MC, not only is the H+-conductivity of BZY improved, but also a fully dense microstructure is achieved by the MC phase.
 |
| Fig. 5 Plots of effective ionic conductivity of BZY-(Li0.62K0.38)2CO3 (MC) (a) vs. temperature in 3%H2O–air and 3%H2O–H2.32 Migration pathways for H+-migration along the Y direction in MPCC, (b) side view and (c) top view. The migration pathways are marked with arrows.33 | |
To explain the conductivity enhancement at high temperature (e.g. T = 600 °C), a new mechanism based on defect reactions involved in the BZY and MC phases was proposed:
|  | (3) |
At the BZY/MC interface, simultaneous H+ conduction takes place in the bulk BZY and along the interface of BZY/MC, where extensive H+ exchange and transfer can occur. To support the above hypothesis, a density functional theory (DFT) was conducted on the system to map out the pathways and energetics of H+ migration in BYZ/MC.33 The calculations explicitly show that the H+ migration prefers a curved pathway along the ZrO2-terminated (110) surface in BaZrO3, see Fig. 5(b) and (c). However, the MC phase provides an alternate, more conductive pathway for H+ to migrate along the surface of BaZrO3, thus facilitating the H+ exchange between BaZrO3 and MC phase across the interface. The study modeled H+ migration in CO32−, Li2CO3 crystals, and (Li2CO3)8 clusters and show that the H+ transfer in (Li2CO3)8 clusters, a surrogate of MC, rather than in solid carbonates, is fast, confirming the feasible and fast H+ transport in the MC phase.34 Overall, the experimental and theoretical results suggest the potential application of dual-phase BYZ/MC MPCC as an electrolyte for intermediate-temperature solid oxide cells.
2.3 Ruddlesden–Popper oxides
The Ruddlesden–Popper (RP) oxides refer to the family with a general formula of An+1BnO3n+1, where n ≥ 1; A is a rare-earth or an alkaline-earth element, and B is a transition metal.35 The formula can also be otherwise viewed as (AO)(ABO3)n, where n is the number of connected layers of corner-sharing BO6 octahedra. The structure is built of consecutive perovskite (ABO3)n blocks alternating with rock-salt AO layers along the c crystallographic axis, see Fig. 6.
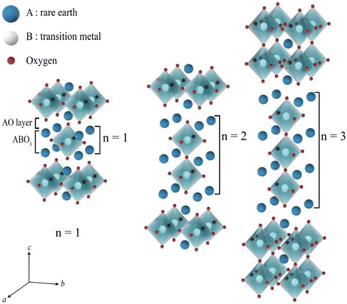 |
| Fig. 6 Idealized representation of the crystal structures of Ruddlesden–Popper (RP) phases.36 | |
Ln2NiO4+δ (Ln = La, Nd, and Pr) is an n = 1 RP compound. They have recently attracted attention due to their low thermal expansion coefficients (TECs), high oxygen bulk diffusion and surface exchange coefficients, which makes them a good candidate for SOCs. While being oxide-ion conductive, Ln2NiO4+δ is also conductive to protons and electrons, thus expanding the active sites for electrochemical reactions.37Fig. 7 shows the possible crystallographic sites for H2O insertion in Ln2MO4+δ (Ln = rare earth element) to produce protons via the following defect reaction:36
|  | (4) |
Here,

represents H attached to the interstitial O and

represents H attached to the lattice O. This mechanism is different from perovskite proton conductors mentioned above, where H
2O reacts with

to produce

. Here in the structure of Ln
2NiO
4+δ, the interstitial O (

) is a prevalent point defect. Therefore, it is envisioned that H
+ hops between

and

to enable H
+ conduction. While the above mechanism still needs experimental verification, many studies agree that the H
+ conducting mechanism is different between RP and traditional perovskite oxides.
 |
| Fig. 7 The crystal structure of Ln2MO4+δ with the arrow indicating the possible water insertion sites.36 | |
The applications of RP materials in H+-conducting solid oxide electrolysis cells (SOECs) have been tried in laboratory studies. The results are sometimes contradicting. For example, an H+-SOEC based on BaZr0.2Ce0.6Y0.2O3−δ (BZCY) electrolyte and Pr2NiO4+δ (PNO) anode was shown with good chemical compatibility and catalytic activity towards water splitting.37 In another study on Ln2NiO4+δ (Ln = La, Pr, Nd) in steam and CO2 environments, however, notable secondary hydroxides and carbonates were detected on the surface after being exposed to high steam pressure (40 bar) at 550 °C.38
Despite numerous early works, the H+ transport mechanisms and properties in RPs are not well understood and attained as perovskite counterparts.39 More comprehensive and in-depth studies are, therefore, needed for understanding H+ conducting mechanisms and measuring H+ conductivity in RP oxides in the future.
2.4 Pyrochlores
Pyrochlore oxides with the composition of A2B2O7 have also been found to be proton conductive.40–42 The acceptor doped A2B2O7 have been shown with improved proton conductivity over the undoped A2B2O7.43 The doped La1.95Ca0.05Zr2O7 exhibits a total conductivity of 6.8 × 10−2 at 600 °C, see Fig. 8(a), in which partial H+ conduction is dominating.43 The oxygen vacancies created by the acceptor doping are the vehicles for proton conduction as illustrated by reaction (1). MD simulations support the necessity of acceptor doping in A2B2O7 on either A- or B-site for long-range H+ conduction.44Fig. 8(b) shows the structure of La2Zr2O7, in which Zr ions (invisible in Fig. 8(b), embedded inside the octahedra) are located at the centers of the distorted octahedra and O ions (small, white balls) at their corners. The La ions (large, dark-shaded balls) together with O′ (medium-size, white balls) constitute an interpenetrating chain-like network. The suggested proton pathway is illustrated in Fig. 8(c) by following (ii) → (ii) → (v) → (v) → (ii) → (ii) → …. Notably, only sites (ii) and (v) must be visited to form a continuous trajectory. The (ii) to (ii) separation is 1.54 Å, whereas (v) to (v) and (ii) to (v) are 0.76 Å and 1.22 Å, respectively. It is also found that negatively charged dopants are associated with H+, and consequently, the proton diffusivity is hindered in their vicinity. However, the binding energy of the associate is quite small in the case of Sr and Ca, making them a reasonable conductor.44
 |
| Fig. 8 (a) Arrhenius plot of conductivity of (La1.95Ca0.05)Zr2O7 in wet H2, (■) σH, (•) σO and (○) σtotal represent partial proton, oxide-ion conductivity, and total conductivity, respectively;43 (b) La2Zr2O7 structure; (c) suggested proton conduction pathway.44 | |
Several applications using pyrochlores as electrolytes in fuel cells, hydrogen sensors, hydrogen separation membranes, and electrochemical ammonia synthesis have been demonstrated. However, the major issue for pyrochlores is the low ionic conductivity, high sintering temperature (>1600 °C), making their practical use in SOCs less attractive.
2.5 Rare-earth ortho-niobates and ortho-tantalates
The rare-earth ortho-niobates and ortho-tantalates refer to AMO4 (A = rare earth; M = Nb, Ta). With 1% Ca acceptor doping, RE1−xAxMO4 exhibits prominent proton conductivity in wet atmospheres below 800 °C. The highest proton conductivity was observed in 1% Ca-doped LaNbO4, which was on the order of 10−3 S cm−1 at 800 °C, see Fig. 9(a).45 However, this class of material experiences monoclinic fergusonite-type structures to tetragonal scheelite-type structures as temperature increases. The proton conductivity suffers a sudden drop as the structure is transformed from a tetragonal to a monoclinic structure, see Fig. 9(a).
 |
| Fig. 9 (a) Protonic conductivities of 1% Ca-doped RENbO4;45 (b) proton migration paths with low potential barriers in the tetragonal phase of LaNbO4. Proton migration pathways are labelled as 1–4 in the figure.47 | |
In addition, the proton mobility in rare-earth ortho-niobates and ortho-tantalates is found to decrease with decreasing ion size, which is understandable because it leads to decreased lattice parameter, the polarizability of the lattice and proton-hosting oxygen sublattice. Typically, the smaller, stiffer lattices give rise to a less dynamic oxygen lattice, leading to its lowered momentum to match proton transfer. The proton mobility is also thought to be favored by the symmetry of the lattice.46
Using DFT method, the in-plane H+-migration pathways in tetragonal LaNbO4 are identified, see Fig. 9(b), in which H+ diffuses by an inter-tetrahedral mechanism within a cation sublattice.47 Briefly, pathways 1 and 2 show the proton rapidly shift position between two adjacent a sites and b sites, respectively, with a comparable potential barrier (0.04 eV and 0.06 eV, respectively) for so-called “oscillatory proton transfer”. Pathway 3 is the additional migration path connecting a and b sites for long-distance proton migration within the plane with the third lowest potential barrier of 0.41 eV. It is also found that the proton rotates around the oxide ion before and after it jumps between the two tetrahedrons, i.e. rotation and hopping, which has also been found computationally in other proton conducting oxides, such as BaCeO3 and BaZrO3. For the out-of-plane proton migration, pathway 4 describes proton migration between two layers with a higher potential barrier of 0.74 eV. So far, only a few literature are available on the proton conductivity in rare-earth ortho-niobates and ortho-tantalates, leaving great space for future exploration of this class of materials.
3. Solid polyanionic salts as proton conductors
3.1 Sulfates
Solid sulphates such as Li2SO4, Li2Mg(SO4)2, LiAgSO4, and Rb2SO4 were first found to be proton conducting in the early 1970s.48 Among these, Li2SO4 exhibits proton conductivity in its α-phase stable between 577 °C (first-order transition temperature) and 860 °C (melting temperature). The proton conducting α-phase has a face centered cubic structure with a space group of Fm3m, see Fig. 10(a). It was later discovered that the first-order transition temperature of the proton-conducting phase could be reduced to ∼450 °C by adding a small amount of Al2O3 into Li2SO4.50,51
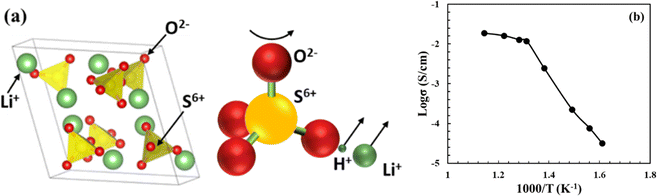 |
| Fig. 10 (a) Crystal structure of the face-centered cubic α-Li2SO4, where SO4 tetrahedron and its rotation pushing out of Li+ and H+ are illustrated.49 (b) Arrhenius plot of proton conductivity of Li2SO4-45 mol%Al2O3 (reproduced from ref. 50). | |
The mechanism of proton conduction follows the paddle wheel model,49 in which steps of proton-attachment to SO42−, i.e. (H-SO4)−, rotation of SO42−, and pushing-out of H+/Li+ are considered, see Fig. 10(a). The proof of proton conductivity was provided by hydrogen concentration cells. The cell with Li2SO4–Al2O3 as the electrolyte showed zero EMF (electromotive force) in O2 atmospheres, but proportional to the logarithm of partial pressure of H2.50 A high proton conductivity in the order of 10−2 to 10−1 S cm−1 has been observed in α-Li2SO4, see Fig. 10(b).50
3.2 Nitrates
Like solid sulphates, solid nitrates (MNO3, M = Li, Na, K, Rb, Cs, etc.) were also found to exhibit high proton conductivity. It was also found that proton conductivity increases with crystallinity of nitrates and cationic radius as a result of the soft M–O bonds and open crystal structures.52 To stabilize and increase the conductivity of MNO3, Al2O3 is often added. The conductivities of RbNO3–Al2O3 and CsNO3–Al2O3 measured by hydrogen concentration cells are comparable to Li2SO4–Al2O3, in the order of 10−2 to 10−1 S cm−1 above 400 °C, see Fig. 11(a).53 For face-centered cubic RbNO3, for example, protons migrate through the interfacial coordinative mechanism between RbNO3 and Al2O3, see Fig. 11(b).
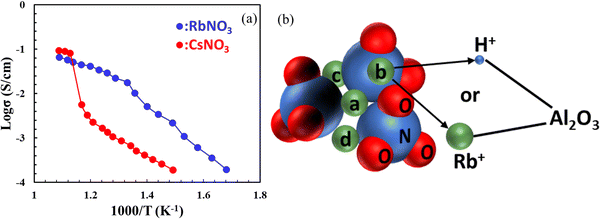 |
| Fig. 11 (a) Arrhenius plot of proton conductivity for CsNO3- and RbNO3–Al2O3 composites (reproduced from ref. 52); (b) a schematic showing bonding between the M+ and NO3− may be broken, which provides a coordination site for the proton. Thus, both Rb+ and H+ may stay at the interface between two phases in the RbNO3–Al2O3 system; here a, b, c, and d represent four non-equivalent M-sites around NO3− (reproduced from ref. 53). | |
The major drawbacks of solid sulphates and nitrates, however, are their weak mechanical strength and low chemical and electrochemical stability, as they could decompose at higher temperatures if not properly stabilized. For instance, nitrates may decompose at a temperature >350 °C,54 while sulphates may undergo reduction to form H2S and H2O in H2-containing atmospheres.55 These drawbacks have limited the practical applications of solid sulphates and nitrates.
3.3 Pyrophosphates
Compared to sulphates and nitrates, phosphates or complex heteropolyacids, such as pyrophosphates, have a better chemical stability. An early study by Zhu et al. suggests that K3PO4–Al2O3 with a cubic α-phase similar to sulphates and nitrates has a proton conductivity of 10−3 to 10−2 S cm−1 at a temperature above 550 °C.50 A series of studies have also shown that Sr or Ca doped rare earth phosphates (LnPO4, Ln = La, Ce, Pr, Nd, and Sm) exhibit high proton conductivity under wet reducing conditions.56–60
The protons’ conduction mechanisms in doped phosphates are generally understood as follows:58
|  | (5) |
|  | (6) |
where

represents Sr dopant on the La-lattice;

represents (P
2O
7)
4− residing on the (PO
4)
3− lattice;

represents proton species residing on the (PO
4)
3− lattice. Assisted by
1H and
31P magic angle spinning-nuclear magnetic resonance (MAS-NMR) and Fourier transform (FT)-Raman spectroscopy measurements, the formation of pyrophosphate ions

at adjacent orthophosphate ions (PO
4)
3− induced by the doping of Sr
2+ into La
3+ has been confirmed, which becomes the active site for water incorporation and the formation of (HPO
4)
2− at (PO
4)
3− sites, resulting in proton conduction.
The conductivities of 1 mol% Sr-doped LaPO4 and CePO4 reached 10−5.2–10−3.5 S cm−1 and 10−5.2–10−3.4 S cm−1, at 500–925 °C, respectively, under H2O/H2 and D2O/D2 atmospheres (pH2O = 4.2 kPa and pH2 = 3 kPa); the results are shown in Fig. 12.56 The lowered conductivity in D2O/D2 indirectly confirmed the nature of proton conduction in these materials.
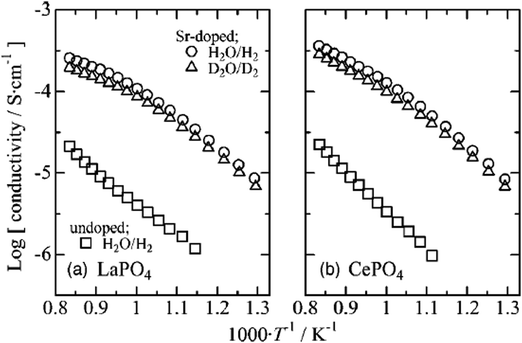 |
| Fig. 12 Conductivities of undoped and 1 mol % Sr-doped (a) LaPO4 and (b) CePO4 under wet reducing conditions; pH2O or pD2O = 4.2 kPa, and pH2 or pD2 = 3 kPa.56 | |
In addition to phosphates, solid acid pyrophosphates TP2O7 (T = Sn, Ti, Si, Ge, Ce, and Zr) have also been found to exhibit proton conductivity in the order of 10−3 to 10−2 S cm−1 within 100 to 400 °C under anhydrous conditions.61–64 The pyrophosphates typically have a face centered rock salt cubic structure with TO6 and P2O7 units sharing corners, see Fig. 13(a). Moreover, Nagao et al. reported that partial substitution of In3+ for Sn4+ in SnP2O7 can improve the proton conductivity from 5.56 × 10−2 to 1.95 × 10−1 S cm−1 at 250 °C, see Fig. 13(b).65 By studying the H/D isotope effect, the proton conduction in TP2O7 was proposed to follow the hopping mechanism, i.e., migration via dissociation of O–H bonds. Moreover, it was also found that the presence of P2O7 ions has a deficiency in the lattice of TP2O7, leading to a lower proton conductivity than undoped pyrophosphates, due to the decrease in proton mobility.
 |
| Fig. 13 (a) Crystal structure of TiP2O7,66 TiO6 octahedra and PO4 tetrahedra are shown in blue and green in color, respectively, and (b) Arrhenius plot of the conductivity of Sn1−xInxP2O7 in dry air (pH2O = ∼0.0075 atm).65 | |
The improved stability of phosphates and pyrophosphates, compared to solid sulphates and nitrates, makes them more practical. Recently, the use of pyrophosphates composite electrolytes has drawn attention due to their stability in a wider voltage and temperature range. For example, Hibino et al. reported that the Sn0.95Al0.05H0.05P2O7 (SAPO)-polytetrafluoroethylene (PTFE) composite shows a high proton conductivity of 0.02 S cm−1 at 200 °C with a highly condensed H3PO4 electrode ionomer in a supercapacitor configuration. Such an electrolyte also exhibits a wide voltage range of ±2 V. The resultant performance of such a supercapacitor reaches an energy density of 32 W h kg−1 at 3 A g−1 and stable 7000 cycles from room temperature to 150 °C.67 Given their relative low cost and ease of preparation, they remain an attractive class of proton conductors for future consideration.
3.4 Solid acids
A group of solid acids, MHAO4 and M3H(AO4)2 with monovalent alkali metal cations M = Rb, Cs, NH4 and tetrahedral oxyanions AO4 (A = S, P, Se) exhibits super proton conductivity above a phase transition temperature between 50 and 250 °C even in a dry atmosphere. This class of material was first reported by Baronov et al.,68 in which high proton conductivity (10−3–10−2 S cm−1 at 140–230 °C) was observed in CsHSO4 and Rb3H(SeO4)2, see Fig. 14(a). The high proton conductivity was attributed to the unique proton disorder in H-bond networks. CsH2PO4 was found later to have a higher transition temperature (230 °C) and decomposition temperature (375 °C in high humidity) than CsHSO4 (140 °C and 200–230 °C, respectively) and exhibit a higher proton conductivity of 2.2 × 10−2 S cm−1 at 200 °C and 4.0 × 10−2 S cm−1 at 240 °C, respectively.69 The proton conduction mechanism in these acids follows successive intra-bond jumps, reorientations of the O–H group and breaking of an old H-bond and forming of a new one, see Fig. 14(b).70
 |
| Fig. 14 (a) Arrhenius plot of proton conductivity of CsH2PO4, Rb3HSeO4, and CsHSO4 (reproduced from ref. 55) and (b) illustration of two proton diffusion movements in CsH2PO4, confirmed via1H pulsed field gradient NMR, 1H NMR spin-lattice relaxation time (Tl), and quasi-elastic neutron scattering measurements.70 | |
The potential of phosphate-based solid acids for application in fuel cells has been shown by Haile et al.55 With a thick CsHSO4 membrane (thickness: ∼1.5 mm) as the electrolyte, the work showed a promising OCV of 1.11 V, and output of 44 mA cm−2 at 150 °C. With a 25 μm thick CsH2PO4 as an electrolyte film, the fuel cell produced a peak power density of 415 mW cm−2 at 240 °C under humidified (pH2O = 0.3 atm) H2 and pure O2.69 However, a further application of solid acids in fuel cells is limited by the strict operating conditions (e.g. high humidity and narrow temperature range). Similar to pyrophosphates, a combination of solid acids with a metal–organic coordination framework shows promising conductivity and stability, especially under low humidity. For example, Ponomareva et al. reported that a composite of CsH5(PO4)2 and a metal–organic coordination framework, Cr-MIL-101 matrix, exhibits a conductivity of ∼10−2 S cm−1 at 130 °C at RH = 10–15%.71 The high proton conductivity under low RH suggests that the hybrid solid acids composite could find potential applications in fuel cells and H2 pumps.
4. Hydrates as proton conductors
The materials that become proton conductive when they are in a fully hydrated state are classified as proton-conducting hydrates. Since this class of materials relies upon the degree of adsorbed water to conduct protons, their operating temperature is typically low.
4.1 Mesoporous nanocomposites
Some mesoporous inorganic materials with nanosized pores in 2 to 50 nm become proton conductive due to physically adsorbing water molecules. Yamamda et al. reported an inorganic TiO2–P2O5 mesoporous nanocomposite with a proton conductivity of 10−2 S cm−1 at 160 °C, under fully saturated humidification conditions, see Fig. 15.72 The high proton conductivity is attributed to the strong P-POH chemisorption enabled by P2O5 under high humidity conditions.73
 |
| Fig. 15 (a) Proton conductivity of (○) TiO2–P2O5 mesoporous nanocomposites and (□) mesoporous silica measured from the RT to 160 °C under 100% relative humidity (RH) conditions. (b) Arrhenius plots of the conductivity of the TiO2–P2O5 mesoporous nanocomposites. A solid line is the least-squares fitting. The activation energy (Ea) of the proton transport was estimated from the slope. Reprinted with permission from J. Am. Chem. Soc., 2005, 127(38), 13092–13093. Copyright (2007) American Chemical Society.72 | |
Similarly, Marschall et al. reported that highly ordered mesoporous silicas with sulfonic acid groups (SO3H) when combined with Nafion to form a functional group (Si-MCM-41, Mobil Composition of Matter No. 41) exhibited very high proton conductivity up to 0.2 S cm−1 under fully saturated humidification conditions.74 The high proton conductivity in the presence of water is derived from the Grotthus mechanism, see Fig. 16.75 In this mechanism, the proton transport is realized by protons hopping from one water molecule to another. To a smaller extent, the diffusion of H3O+ also enhances proton transport. At higher temperatures, hopping and diffusion become faster since the rotation and vibration of anchoring propyl chains increase, allowing the SO3H groups at the end of the chains to encounter each other more freely, thus facilitating the direct proton transport. A recent study by Vinothkannan et al. shows substantially high proton conductivity (45.1 mS cm−1) under RH = 50% and 120 °C for the optimized Nafion/SO3H-1 wt% unzipped graphite nanofiber (UGNF) membrane.76
 |
| Fig. 16 (a) Proton conductivity of 40% SO3H-MCM-41 (mw) at 0% (◆), 50% (○), and 100% (●) RH, Reprinted with permission from Chem. Mater. 19, 26 (2007): 6401–6407. Copyright (2007) American Chemical Society.74 and (b) simplified schematic representation of proton transport via the surface mechanism, Grotthuss mechanism, and vehicle mechanism.75 | |
In contrast to Nafion fouls, for which the proton conductivity decreases drastically above 100 °C due to the water loss, water seems to be kept inside the channels of mesoporous nanocomposites due to the geometry of the pores which fixes the sulfonic acid groups, thus maintaining proton conductivity even at higher than 100 °C. It was also found that the proton conductivity increased with the extent of substitution of metasilicate with MCM. The highest proton conductivity of 0.2 S cm−1 was achieved with a 40% SO3H-MCM-41 (mw) sample due to the high pore filling with SO3H groups and the absence of parasitic pores.
4.2 Heteropolyacid hydrates
Early studies have found that some heteropolyacids in a hydrated state, such as H4SiW12O40·28H2O, H3PW12O40·29H2O, and H3PMo12O40·29H2O, exhibit high proton conductivity.77–79 The proton conductivities are in the order of 10−2 and 10−1 S cm−1 at room temperature. Large and globular anions (e.g. [Mo12PO40]3−) with Keggin structures are present in these hydrates, with crystal water showing violet thermal motion which can be easily dehydrated and rehydrated. These crystal waters contribute to the high proton conductivity, but only within a narrow window. Temperature and humidity are key factors in maintaining stable hydrates, where 80 °C and 70–80% RH are typically required conditions. In addition, pelletization or other processing methods can lead to the loss of crystallization in these hydrates. Thus, some porous oxides such as SiO2 and Al2O3 have been often used as a matrix to reinforce the stability and robustness of heteropolyacid hydrates, which makes their application in solid electrolytes, sensors, and electrochromic displays possible.80,81
4.3 Layered hydrates
Another group of hydrates studied as a proton conductor has a layered structure. Examples include HUO2PO4·4H2O,81 α-Zr(HPO4)2·nH2O,82 γ-Zr(PO4)(H2PO4)·2H2O,83 and γ-Zr sulfoaryl phosphonates.84 They are acid groups that exhibit high proton conductivity of 10−4 to 10−2 S cm−1 over a wider temperature range, but thermally more stable than heteropolyacids hydrates. In these compounds, a phase transition occurred above ∼50 °C,85 resulting in a significant increase in proton conductivity, due to the formation of an interlamellar H-bonded network with abundant H3O+ and high concentration of H+.
The conduction mechanism is similar to that in mesoporous nanocomposites, with a hybrid Grotthuss-vehicle mechanism involving an intermolecular transfer (hopping) and an intramolecular transfer of protons. The former could be facilitated by the high concentration of H3O+ in the structure, and the latter is most likely facilitated by the high concentration of H-bond vacancies.
Some oxides, such as Sb2O5,86 SnO2,87 V2O5, ZrO288 and VO2,89 are also found to be proton conductive in their hydrated states. Proton conductivities of 10−4 to 10−2 S m−1 are found below 300 °C. Typically, maintaining saturated water vapor pressure is required to achieve sustainable proton conductivity. The proton conduction in such hydrates typically follows a pathway involving first, rotation around the O atom, and then migration between O atoms, see Fig. 17.
 |
| Fig. 17 Migration pathways for H+ in H-VO2.89 | |
Despite the high proton conductivity of all these hydrates, their practical application is still limited due to the difficulty of retaining water and poor thermal stability during operation.
5. Hydrides as hydride-anion conductors
In chemistry, a hydride is formally an anion of hydrogen, H−. Therefore, hydrides often refer to compounds and ions in which hydrogen is covalently attached to a less electronegative element. In such cases, the H center has a nucleophilic character, which contrasts with the protic character of acids.
5.1 Hydrides
Early studies revealed that some alkaline earth metal hydrides, such as CaH2 and SrH2, exhibit hydride anion (H−) conductivity up to 10 S cm−1 above 780 °C,90 and application of such materials as electrolytes for fuel cells was also reported.91 More recently, a study on BaH2/BaD2 by Verbraeken et al. suggested that the high proton conductivity was attributed to H− and achieved in a Ni2In-type structure transformed from a cotunnite structure at ∼470 °C, where a sharp increase in conductivity was observed.92 The phase transition from cotunnite to high-symmetry Ni2In type structures was also promoted by high pressure. A large concentration of hydride vacancies was also observed in the structure with a composition of roughly BaH/D1.84, which contributes to the proton conductivity. In addition, small Ba-to-H or deuteride bond length, high isotropic temperature factors for D1 and D2 (7.6 and 11.6 Å), small mass and high mobility of D ions, see Fig. 18(a), all give rise to the superionic conductivity in BaD2. The conductivity of BaH2 is in the range of 0.04–0.2 S cm−1 between 470 and 630 °C, see Fig. 18(b).
 |
| Fig. 18 (a) Crystal structure of BaD2, and (b) Arrhenius plot of hydride-anion conductivity of BaH2.92 | |
Despite the high hydride anion conductivity, the narrow operation window largely restricts the practical application of BaH2. More recently, Ubukata et al. reported a solid solution of Ba2−δH3−2δX (X = Cl, Br, or I) with a layered anion-ordered structure, which exhibited excellent hydride conductivity at low temperatures (e.g. 1 mS cm−1 at 200 °C).93 The layered anion ordering and Schottky defects in such materials likely suppress the structural transition commonly occurring during cooling, thus retaining a highly symmetric hexagonal lattice at lower temperatures for H− conduction. Furthermore, AELiH3 (AE: alkaline earth metals) perovskites are also found to exhibit high H−-conduction at low temperatures (e.g. 5.0 × 10−6 S cm−1 at room temperature for Sr0.925Na0.075LiH2.925),94 implying that the employment of aliovalent cations on the A site in perovskite-type hydrides leads to the formation of hydrogen vacancies, thus enabling fast H−-conduction even at very low temperatures. These new discoveries widen the potential of hydrides for applications over a wider temperature range.
5.2 Perovskite and fluorite-type oxyhydrides
Perovskite and fluorite-type oxyhydrides containing H− in the lattice, such as La2−xSrxLiH1+xO3−x,95 Ba2ScHO3,96 LaSrCoO3H0.7,97etc., have been shown to conduct H− in a similar manner to the above BaH2 hydrides. Kobayashi et al. reported that rare-earth lithium oxyhydrides of La2−x−ySrx+yLiH1−x+yO3−y conduct H− over oxygen sublattices. One of the promising compositions, La2LiHO3, has been successfully synthesized into a K2NiF4-type structure with an orthorhombic structure at 923 K and 2 GPa for 30 min. In this structure, both H− and O2− are ordered on the axial anion sites, see Fig. 19(a). The conductivity of La2−xSrxLiH1−xO3 series (0 ≤ x ≤ 0.2, y = 0) is shown in Fig. 19(b), suggesting that Sr-induced vacancies increase H− conductivity. With La2LiHO3 as the electrolyte, a cell consisting of Ti/o-La2LiHO3/TiH2 has been constructed and tested; the results are shown in Fig. 19(c).95 The cell exhibited an initial open circuit voltage of 0.28 V at 300 °C, consistent with the theoretical value of a Ti/TiH2 redox couple. Under a constant discharge current of 0.5 mA, a steep drop-off in the cell voltage from 0.28 to 0.06 V was observed for the first reaction step, corresponding to the increase in the H− content at the anode, followed by the cell voltage decreasing gradually to 0.0 V as H− was continued added into Ti, implying the reaction between H and Ti was completed.
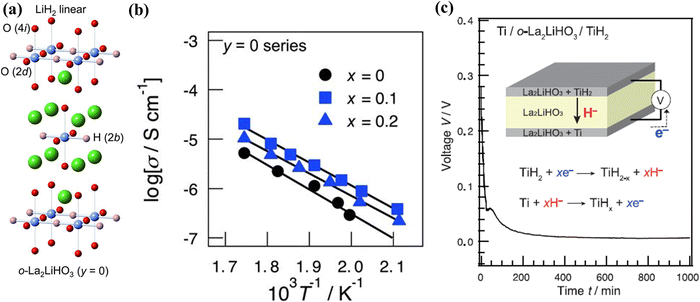 |
| Fig. 19 (a) The crystal structure of o-La2LiH3O3, (b) Arrhenius plot of the ionic conductivity of La2−xSrxLiH1−xO3 (y = 0, 0 ≤ x ≤ 0.2), and (c) discharge curve of cell Ti/o-La2LiHO3/TiH2. The inset shows the cell configuration and the proposed electrochemical reactions.95 | |
The synchrotron XRD indicates that the La2LiHO3 electrolyte is stable when in contact with the Ti and TiH2 electrodes during the reaction, while phase changes were detected for both the cathode and anode. In the cathode, the initial δ-TiH2 phase was found to transform into α-Ti upon releasing hydrogen; the corresponding lattice shrinkage was confirmed by the shift to a higher 2θ in the XRD pattern.
A more recent work shows that Ba–Li oxyhydride, Ba1.75LiH2.7O0.9, synthesized under ambient pressure with a K2NiF4-type structure exhibited a high H− conductivity (∼10−2 S cm−1 at 300 °C) after an order–disorder transition.98 Overall, H− conductivity in such oxide-based framework structures is found to be almost independent of temperature but closely related to the anion ordering and vacancies.
Other than the H−-conducting oxyhydrides, mixed-electronic and ionic conductivity was also found in some oxyhydrides such as BaTiO3−xHx.99 The high electronic conduction (e.g. 10−4 S cm−1 at 25 °C in BaTiO2.4H0.6) might find these materials as electrodes in an electrochemical cell.
Fluorite-type oxyhydrides are another group of hydrides with high H− conductivity comparable to perovskite oxyhydrides. LaH3−2xOx (x = 0.54) demonstrates the highest H−-conductivity of 2.6 × 10−2 S cm−1 at T = 342 °C, see Fig. 20.100 The crystal structure of LaD2O0.5, an isotopic analogue of LaH2O0.5, is tetragonal, as determined by neutron powder diffraction (NPD). The light mass and large polarizability of H− and the framework comprising of densely packed H− in LaH3−2xOx are crucial factors that enable significant temperature dependence of the H− conductivity.
 |
| Fig. 20 (a) Crystal structure of LaD2O0.5 determined by NPD. Solid and dashed lines represent the unit cells of the FCC and tetragonal structures, respectively. Green, pink, yellow, and grey colors denote the fractions of La3+, D−, O2−, and vacancies at each crystallographic site, respectively, and (b) Arrhenius plots of LaH3−2xOx and H− conductivity measured by AC impedance spectroscopy.100 | |
The successful demonstration of an all-solid-state electrochemical cell using oxyhydrides as an electrolyte presents the possibility of developing solid electrochemical devices based on H− conductors. Although recent studies have shown the possibility of ambient pressure synthesis of oxyhydrides, the requirement for high pressure in synthesizing the desired oxyhydrides and the lack of stability study are major obstacles for practical applications of oxyhydrides.
5.3 Nitrohydrides and hydrofluorides
Like oxyhydrides, H− diffusion was also observed in nitrohydrides such as Ba2NH,101,102 Sr2NH,103 and Sr2LiH2N.104 Studies have discovered that these nitrohydrides exhibit layered structures at high concentrations (up to 15%) of H-vacancies, which favors H− diffusion. The H− migration proceeds in the planes occupied by H−, in uncorrelated jumps between regular lattice sites, see Fig. 21(a). The high and pure mobility of H− gives rise to the high conductivity of 1 S cm−1 above certain temperatures (e.g. 525 K for Ba2NH), see Fig. 21(b). However, there is a lack of systematic study on the origin of such high conductivity, leaving nitrohydrides group wide open for further investigations.
 |
| Fig. 21 (a) Layered type structure of Ba2NH with distorted Ba-octahedra centered alternately by H− and N3−, with corresponding distance, (b) Arrhenius plot of H− conductivity in Ba2NH, and (c) diffusion of hydride anions in Ba2NH via uncorrelated jumps between regular hydrogen sites.101 | |
Hydrofluorides, for example, CaF2−xHx,105 and LiBaF3−xHx,106 have a perovskite structure, in which ionic radii of H− and F− are similar (rH− = 2.08 Å, rF− = 1.33 Å). EMF measurements reveal the high conductivity of LiBaF3−xHx in H2 atmosphere, varying from 10−5–10−2 S cm−1 at temperatures of 330–650 °C, see Fig. 22. However, such hydride compounds are subject to oxidation and thus suffered stability issues, making them difficult in applications.
 |
| Fig. 22 Arrhenius plots of conductivity of LiBaF3−xHx in H2: □x = 0, ◊x = 0.05, ○x = 0.1, Δx = 0.2, ● LiBa0.9K0.1F2.8H0.1.106 | |
6. Summary
In summary, the most investigated H+ and H− conductors have been reviewed in this article in terms of structures, conduction mechanisms, conductivities, and optimal conducting conditions. The representative conductors for each class are summarized in Table 1.
Table 1 Representative H+/H− conductors and their corresponding conductivities and optimal operating conditions
Material |
Conductivity (S cm−1) |
Optimal operating conditions |
Ref. |
Oxides as H+ conductors |
Perovskites |
BaCe0.9Y0.1O3−δ (BCY) |
1.8 × 10−2–7 × 10−2 at 600–1000 °C |
500–750 °C |
27
|
BaZr0.9Y0.1O3−δ (BZY) |
1.6 × 10−3–6 × 10−3 at 600–1000 °C |
500–750 °C |
28
|
BaZr0.1Ce0.7Y0.1Yb0.1O3−δ (BZCYYb) |
5 × 10−3–5 × 10−2 at 400–750 °C |
500–750 °C |
23
|
RP oxides |
BaLaIn0.9Ti0.1O4.05 |
7.4 × 10−8–2.6 × 10−6 at 250–450 °C |
|
39
|
Pyrochlores oxides |
(La1.95Ca0.05)Zr2O7−δ |
6.3 × 10−5–1.1 × 10−3 at 400–900 °C |
500–800 °C |
41
|
Rare-earth ortho-niobates and ortho-tantalates |
1% Ca-doped LaNbO4 |
2 × 10−5–9 × 10−4 at 400–1000 °C |
700–1000 °C |
45
|
Perovskites with MC |
BZY-(Li0.62K0.38)2CO3 |
0.01–0.78 at 400–660 °C |
500–650 °C |
33
|
|
Solid polyanionic compounds as H+ conductors |
Phosphates/pyrophosphates |
1 mol% Sr-doped LaPO4 |
8.7 × 10−6–2.6 × 10−4 at 500–930 °C |
500–900 °C |
56
|
1 mol% Sr-doped CePO4 |
8.8 × 10−6–3.8 × 10−4 at 500–930 °C |
500–900 °C |
56
|
Sn0.9In0.1P2O7 |
7.1 × 10−2–1.8 × 10−1 at 75–300 °C |
200–300 °C |
65
|
CsHSO4 |
1.6 × 10−7–3.5 × 10−2 at 100–190 °C |
140–190 °C |
55
|
Rb3H(SeO4)2 |
9.6 × 10−8–8.3 × 10−3 at 75–235 °C |
180–235 °C |
55
|
CsH2PO4 |
3.1 × 10−6–2.71 × 10−2 at 155–252 °C |
200–250 °C, pH2O ≥ 0.4 atm |
68
|
Sulphates and nitrates |
Li2SO4-45 mol%Al2O3 |
3.2 × 10−5–1.8 × 10−2 at 350–600 °C |
450–600 °C |
50
|
RbNO3–Al2O3 |
2 × 10−4–6.6 × 10−2 at 325–650 °C |
500–650 °C |
52 and 53
|
CsNO3–Al2O3 |
1.9 × 10−4–9.4 × 10−2 at 400–650 °C |
550–650 °C |
52 and 53
|
|
Hydrates as H+ conductors |
Mesoporous nanocomposites |
TiO2–P2O5 |
6 × 10−3–1.5 × 10−2 at 50–200 °C |
50–200 °C, 100%RH |
72
|
40% SO3H-MCM-41 |
1 × 10−3–2 × 10−2 at 60–140 °C |
60–140 °C, 100%RH |
74
|
Heteropolyacid hydrates |
H4SiW12O40·28H2O |
2 × 10−2 at 25 °C |
<100 °C, >70%RH |
77
|
H3PW12O40·29H2O |
8 × 10−2 at 25 °C |
<100 °C, >70%RH |
78
|
H3PMo12O40·29H2O |
1.7 × 10−1 at 25 °C |
<100 °C, >70%RH |
79
|
Layered hydrates |
HUO2PO4·4H2O |
5 × 10−3 at 25 °C |
<100 °C, high RH |
82
|
Oxide hydrates |
V2O5·nH2O, ZrO2·nH2O |
1 × 10−2 at 25 °C |
<150 °C, 100%RH |
89
|
|
H− conductors |
Hydrides |
BaH2 |
5.4 × 10−6–1.68 × 10−1 at 100–630 °C |
470–630 °C |
92
|
Sr0.925Na0.075LiH2.925 |
5.0 × 10−6 at 25 °C |
25–150 °C |
94
|
Perovskite oxyhydrides |
La2Sr0.1LiH0.9O3 |
3.86 × 10−7–2.04 × 10−5 at 200–300 °C |
200–300 °C |
95
|
Ba1.75LiH2.7O0.9 |
2.24 × 10−7–1.38 × 10−2 at 210–350 °C |
300–350 °C |
98
|
LaH1.92O0.54 |
9.24 × 10−10–2.6 × 10−2 at 85–342 °C |
250–342 °C |
100
|
Nitrohydrides and hydrofluorides |
Ba2NH |
3.5 × 10−6–1.1 at 35–435 °C |
350–400 °C |
101
|
LiBaF2.8H0.2 |
4 × 10−4–1.7 × 10−2 at 350–600 °C |
350–600 °C |
106
|
Overall, despite some results shown in electrochemical cells and H2 separation membranes, no industrial scale devices have been demonstrated for proton and hydride-anion conductors. The main challenges for H+/H− based electrochemical cells to be practical are poor chemical, thermal and mechanical stabilities, difficulty to process and chemical reactivity under operating conditions. To advance H+/H− based electrochemical cells, further scientific studies and engineering efforts are greatly needed.
Conflicts of interest
There are no conflicts to declare.
Acknowledgements
We would like to thank the National Science Foundation for the financial support under award number 1801284 and the Department of Energy, Office Fossil Fuels, National Energy Technology Laboratory for the financial support under award number DE-FE-0032111.
References
- P. F. Lang and C. S. Barry, Ionic radii for Group 1 and Group 2 halide, hydride, fluoride, oxide, sulfide, selenide and telluride crystals, Dalton Trans., 2010, 39(33), 7786–7791, 10.1039/C0DT00401D.
- L. Malavasi, M. Karlsson and M. Coduri, Structure-Property Correlation in Oxide-Ion and Proton Conductors for Clean Energy Applications: Recent Experimental and Computational Advancements, J. Mater. Chem. A, 2022 10.1039/D1TA10326A.
- C. Duan, J. Huang, N. Sullivan and R. O’Hayre, Proton-conducting oxides for energy conversion and storage, Appl. Phys. Rev., 2020, 7(1), 011314, DOI:10.1063/1.5135319.
- K.-D. Kreuer, Proton-conducting oxides, Annu. Rev. Mater. Res., 2003, 33(1), 333–359, DOI:10.1146/annurev.matsci.33.022802.091825.
- H. Iwahara, Proton conducting ceramics and their applications, Solid State Ionics, 1996, 86, 9–15, DOI:10.1016/0167-2738(96)00087-2.
- N. Danilov, A. Tarutin, J. Lyagaeva, G. Vdovin and D. Medvedev, CO2-promoted hydrogen production in a protonic ceramic electrolysis cell, J. Mater. Chem. A, 2018, 6(34), 16341–16346, 10.1039/C8TA05820B.
- S. Rajendran, N. K. Thangavel, H. Ding, Y. Ding, D. Ding and L. M. R. Arava, Tri-doped BaCeO3–BaZrO3 as a chemically stable electrolyte with high proton-conductivity for intermediate temperature solid oxide electrolysis cells (SOECs), ACS Appl. Mater. Interfaces, 2020, 12(34), 38275–38284, DOI:10.1021/acsami.0c12532.
- R. Murphy, Y. Zhou, L. Zhang, L. Soule, W. Zhang, Y. Chen and M. Liu, A new family of proton-conducting electrolytes for reversible solid oxide cells: BaHfxCe0.8−xY0 1Yb0.1O3−δ, Adv. Funct. Mater., 2020, 30(35), 2002265, DOI:10.1002/adfm.202002265.
- A. P. Tarutin, M. Yu Gorshkov, I. N. Bainov, G. K. Vdovin, A. I. Vylkov, J. G. Lyagaeva and D. A. Medvedev, Barium-doped nickelates Nd2–xBaxNiO4+δ as promising electrode materials for protonic ceramic electrochemical cells, Ceram. Int., 2020, 46(15), 24355–24364, DOI:10.1016/j.ceramint.2020.06.217.
- M. Sakbodin, E. Schulman, S. Cheng, Y.-L. Huang, Y. Pan, P. Albertus, E. D. Wachsman and D. Liu, Direct Nonoxidative Methane Conversion in an Autothermal Hydrogen-Permeable Membrane Reactor, Adv. Energy Mater., 2021, 11(46), 2102782, DOI:10.1002/aenm.202102782.
- G. Ma, F. Zhang, J. Zhu and G. Meng, Proton conduction in La0.9Sr0.1Ga0.8Mg0.2O3−α, Chem. Mater., 2006, 18(25), 6006–6011, DOI:10.1021/cm0612954.
- N. Xu, C. Zhang and K. Huang, Proton-mediated energy storage in intermediate-temperature solid-oxide metal–air batteries, J. Mater. Chem. A, 2018, 6(42), 20659–20662, 10.1039/C8TA08180H.
- C. J. Bartel, C. Sutton, B. R. Goldsmith, R. Ouyang, C. B. Musgrave, L. M. Ghiringhelli and M. Scheffler, New tolerance factor to predict the stability of perovskite oxides and halides, Sci. Adv., 2019, 5(2), eaav0693, DOI:10.1126/sciadv.aav0693.
- M. Papac, V. Stevanović, A. Zakutayev and R. O’Hayre, Triple ionic–electronic conducting oxides for next-generation electrochemical devices, Nat. Mater., 2021, 20(3), 301–313, DOI:10.1038/s41563-020-00854-8.
- H. Wang, X. Wang, B. Meng, X. Tan, K. S. Loh, J. Sunarso and S. Liu, Perovskite-based mixed protonic–electronic conducting membranes for hydrogen separation: recent status and advances, J. Ind. Eng. Chem., 2018, 60, 297–306, DOI:10.1016/j.jiec.2017.11.016.
- M. Ni and Z. Shao, Fuel cells that operate at 300 to 500 C, Science, 2020, 369(6500), 138–139, DOI:10.1126/science.abc9136.
- H. Su and Y. H. Hu, Degradation issues and stabilization strategies of protonic ceramic electrolysis cells for steam electrolysis, Energy Sci. Eng., 2022, 10(5), 1706–1725, DOI:10.1002/ese3.1010.
- W. Wang, D. Medvedev and Z. Shao, Gas humidification impact on the properties and performance of perovskite-type functional materials in proton-conducting solid oxide cells, Adv. Funct. Mater., 2018, 28(48), 1802592, DOI:10.1002/adfm.201802592.
- H. Iwahara, T. Esaka, H. Uchida and N. Maeda, Proton conduction in sintered oxides and its application to steam electrolysis for hydrogen production, Solid State Ionics, 1981, 3, 359–363, DOI:10.1016/0167-2738(81)90113-2.
- H. Uchida, N. Maeda and H. Iwahara, Relation between proton and hole conduction in SrCeO3-based solid electrolytes under water-containing atmospheres at high temperatures, Solid State Ionics, 1983, 11(2), 117–124, DOI:10.1016/0167-2738(83)90048-6.
- K.-D. Kreuer, W. Münch, A. Fuchs, U. Klock and J. Maier, Proton conducting alkaline earth zirconates and titanates for high drain electrochemical applications, Solid State Ionics, 2001, 145(1–4), 295–306, DOI:10.1016/S0167-2738(01)00953-5.
- C. Duan, R. Kee, H. Zhu, N. Sullivan, L. Zhu, L. Bian, D. Jennings and R. O’Hayre, Highly efficient reversible protonic ceramic electrochemical cells for power generation and fuel production, Nat. Energy, 2019, 4(3), 230–240, DOI:10.1038/s41560-019-0333-2.
- L. Yang, S. Wang, K. Blinn, M. Liu, Z. Liu, Z. Cheng and M. Liu, Enhanced sulfur and coking tolerance of a mixed ion conductor for SOFCs: BaZr0.1Ce0.7Y0.2–xYbxO3–δ, Science, 2009, 326(5949), 126–129, DOI:10.1126/science.1174811.
- S. Choi, J. K. Chris, Y. Liang, X. Zhang, I. Takeuchi, H.-I. Ji and S. M. Haile, Exceptional power density and stability at intermediate temperatures in protonic ceramic fuel cells, Nat. Energy, 2018, 3(3), 202–210, DOI:10.1038/s41560-017-0085-9.
- L. Bi, P. S. Shahid and E. Traversa, Y-doped BaZrO3 as a chemically stable electrolyte for proton-conducting solid oxide electrolysis cells (SOECs), J. Mater. Chem. A, 2015, 3(11), 5815–5819, 10.1039/C4TA07202B.
- G. C. Mather and M. S. Islam, Defect and dopant properties of the SrCeO3-based proton conductor, Chem. Mater., 2005, 17(7), 1736–1744, DOI:10.1021/cm047976l.
- Y. Yoo and N. Lim, Performance and stability of proton conducting solid oxide fuel cells based on yttrium-doped barium cerate-zirconate thin-film electrolyte, J. Power Sources, 2013, 229, 48–57, DOI:10.1016/j.jpowsour.2012.11.094.
- C. Y. R. Vera, H. Ding, D. Peterson, W. T. Gibbons, M. Zhou and D. Ding, A mini-review on proton conduction of BaZrO3-based perovskite electrolytes, J. Phys.: Energy, 2021, 3(3), 032019, DOI:10.1088/2515-7655/ac12ab.
- S. Barison, M. Battagliarin, S. Boldrini, G. Chiodelli and L. Doubova, Monica Fabrizio, Rosalba Gerbasi, and Lorenzo Malavasi. BaCe1−x−yZrxYyO3−d Proton Conductors: The Role of the Synthetic Route on their Properties, ECS Trans., 2008, 11(33), 89, DOI:10.1149/1.3038912.
- S. Barison, M. Battagliarin, T. Cavallin and L. Doubova, Monica Fabrizio, Cecilia Mortalo, Stefano Boldrini, Lorenzo Malavasi, and Rosalba Gerbasi. High conductivity and chemical stability of BaCe1−x−yZrxYyO3−δ proton conductors prepared by a sol–gel method, J. Mater. Chem., 2008, 18(42), 5120–5128, 10.1039/B808344D.
- S. Choi, C. D. Timothy and M. H. Sossina, Protonic ceramic electrochemical cells for hydrogen production and electricity generation: exceptional reversibility, stability, and demonstrated faradaic efficiency, Energy Environ. Sci., 2019, 12(1), 206–215, 10.1039/C8EE02865F.
- X. Li, N. Xu, L. Zhang and K. Huang, Combining proton conductor BaZr0.8Y0.2O3−δ with carbonate: Promoted densification and enhanced proton conductivity, Electrochem. Commun., 2011, 13(7), 694–697, DOI:10.1016/j.elecom.2011.04.012.
- X. Lei, K. Huang and C. Qin, Enhanced interfacial proton migration on BaZr (Y) O3 by molten carbonate: a first principles study, Solid State Ionics, 2016, 289, 48–54, DOI:10.1016/j.ssi.2016.02.018.
- X. Lei, C. Qin and K. Huang, Energetics of proton transfer in alkali carbonates: a first principles calculation, RSC Adv., 2015, 5(69), 56205–56209, 10.1039/C5RA07975F.
- G. Nirala, D. Yadav and S. Upadhyay, Ruddlesden–Popper phase A2BO4 oxides: Recent studies on structure, electrical, dielectric, and optical properties, J. Adv. Ceram., 2020, 9(2), 129–148, DOI:10.1007/s40145-020-0365-x.
- A. P. Tarutin, G. L. Julia, D. A. Medvedev, L. Bi and A. A. Yaremchenko, Recent advances in layered Ln2NiO4+δ nickelates: Fundamentals and prospects of their applications in protonic ceramic fuel and electrolysis cells, J. Mater. Chem. A, 2021, 9(1), 154–195, 10.1039/D0TA08132A.
- W. Li, B. Guan, L. Ma, S. Hu, N. Zhang and X. Liu, High performing triple-conductive Pr2NiO4+δ anode for proton-conducting steam solid oxide electrolysis cell, J. Mater. Chem. A, 2018, 6(37), 18057–18066, 10.1039/C8TA04018D.
- S. Upasen, P. Batocchi, F. Mauvy, A. Slodczyk and P. Colomban, Protonation and structural/chemical stability of Ln2NiO4+δ ceramics vs. H2O/CO2: High temperature/water pressure ageing tests, J. Alloys Compd., 2015, 622, 1074–1085, DOI:10.1016/j.jallcom.2014.11.017.
- N. Tarasova, I. Animitsa, A. Galisheva and V. Pryakhina, Protonic transport in the new phases BaLaIn0.9M0.1O4.05 (M = Ti, Zr) with Ruddlesden–Popper structure, Solid State Sci., 2020, 101, 106121, DOI:10.1016/j.solidstatesciences.2020.106121.
- H. Yamamura, H. Nishino and K. Kakinuma, Ac conductivity for Eu2Zr2O7 and La2Ce2O7 with pyrochlore-type composition, J. Ceram. Soc. Jpn., 2004, 112(10), 553–558, DOI:10.2109/jcersj.112.553.
- T. Omata, K. Okuda, S. Tsugimoto and S. Otsuka-Matsuo-Yao, Water and hydrogen evolution properties and protonic conducting behaviors of Ca2+-doped La2Zr2O7 with a pyrochlore structure, Solid State Ionics, 1997, 104(3–4), 249–258, DOI:10.1016/S0167-2738(97)00433-5.
- T. Shimura, M. Komori and H. Iwahara, Ionic conduction in pyrochlore-type oxides containing rare earth elements at high temperature, Solid State Ionics, 1996, 86, 685–689, DOI:10.1016/0167-2738(96)00148-8.
- T. Omata and S. Otsuka-Yao-Matsuo, Electrical properties of proton-conducting Ca2+-doped La2Zr2O7 with a pyrochlore-type structure, J. Electrochem. Soc., 2001, 148(6), E252, DOI:10.1149/1.1369370.
- M. E. Björketun, S. K. Christopher, B. J. Nyman and G. Wahnström, Protonic defects in pure and doped La2Zr2O7 pyrochlore oxide, Solid State Ionics, 2008, 178(31–32), 1642–1647, DOI:10.1016/j.ssi.2007.10.014.
- R. Haugsrud and T. Norby, Proton conduction in rare-earth ortho-niobates and ortho-tantalates, Nat. Mater., 2006, 5(3), 193–196, DOI:10.1038/nmat1591.
- K. D. Kreuer, On the complexity of proton conduction phenomena, Solid State Ionics, 2000, 136, 149–160, DOI:10.1016/S0167-2738(00)00301-5.
- H. Fjeld, K. Toyoura, R. Haugsrud and T. Norby, Proton mobility through a second order phase transition: theoretical and experimental study of LaNbO4, Phys. Chem. Chem. Phys., 2010, 12(35), 10313–10319, 10.1039/C002851G.
- B. Heed, B. Zhu, B.-E. Mellander and A. Lundén, Proton conductivity in fuel cells with solid sulphate electrolytes, Solid State Ionics, 1991, 46(1–2), 121–125, DOI:10.1016/0167-2738(91)90139-3.
- B. Zhu and B.-E. Mellander, Intermediate temperature fuel cells with electrolytes based on oxyacid salts, J. Power Sources, 1994, 52(2), 289–293, DOI:10.1016/0378-7753(94)02145-7.
-
B. Zhu and B.-E. Mellander, Novel proton conducting ceramics for solid state fuel cells, ECS Proceedings Volumes 1993, 1993, vol. 1, p. 156 DOI:10.1149/199304.0156PV.
- B. Zhu, Z. H. Lai and B.-E. Mellander, Structure and ionic conductivity of lithium sulphate–aluminum oxide ceramics, Solid State Ionics, 1994, 70, 125–129, DOI:10.1016/0167-2738(94)90296-8.
- B. Zhu and B.-E. Mellander, Proton conduction in nitrate-based oxides and related ceramics at intermediate temperatures, Solid State Ionics, 1994, 70, 285–290, DOI:10.1016/0167-2738(94)90324-7.
- B. Zhu and B.-E. Mellander, Proton conduction in salt-ceramic composite systems, Solid State Ionics, 1995, 77, 244–249, DOI:10.1016/0167-2738(94)00275-W.
- N. F. Uvarov, P. Vaněk, Y. I. Yuzyuk, V. Železný, V. Studnička, B. B. Bokhonov, V. E. Dulepov and J. Petzelt, Properties of rubidium nitrate in ion-conducting RbNO3–Al2O3 nanocomposites, Solid State Ionics, 1996, 90(1–4), 201–207, DOI:10.1016/S0167-2738(96)00400-6.
- S. M. Haile, A. B. Dane, C. R. I. Chisholm and R. B. Merle, Solid acids as fuel cell electrolytes, Nature, 2001, 410(6831), 910–913, DOI:10.1038/35073536.
- N. Kitamura, K. Amezawa, Y. Tomii, T. Hanada, N. Yamamoto, T. Omata and S. Otsuka-Yao-Matsuo, Electrical conduction properties of Sr-doped LaPO4 and CePO4 under oxidizing and reducing conditions, J. Electrochem. Soc., 2005, 152(4), A658, DOI:10.1149/1.1861993.
- T. Norby and N. Christiansen, Proton conduction in Ca-and Sr-substituted LaPO4, Solid State Ionics, 1995, 77, 240–243, DOI:10.1016/0167-2738(94)00274-V.
- K. Amezawa, H. Maekawa, Y. Tomii and N. Yamamoto, Protonic conduction and defect structures in Sr-doped LaPO4, Solid State Ionics, 2001, 145(1–4), 233–240, DOI:10.1016/S0167-2738(01)00963-8.
- S. Gallini, M. Hänsel, T. Norby, M. T. Colomer and J. R. Jurado, Impedance spectroscopy and proton transport number measurements on Sr-substituted LaPO4 prepared by combustion synthesis, Solid State Ionics, 2003, 162, 167–173, DOI:10.1016/S0167-2738(03)00220-0.
- N. Kitamura, K. Amezawa, Y. Tomii and N. Yamamoto, Protonic conduction in rare earth orthophosphates with the monazite structure, Solid State Ionics, 2003, 162, 161–165, DOI:10.1016/S0167-2738(03)00219-4.
- G. Alberti, M. Casciola, S. Cavalaglio and R. Vivani, Proton conductivity of mesoporous zirconium phosphate pyrophosphate, Solid State Ionics, 1999, 125(1–4), 91–97, DOI:10.1016/S0167-2738(99)00162-9.
- M. Nagao, T. Kamiya, P. Heo, A. Tomita, T. Hibino and M. Sano, Proton conduction in In3+-doped SnP2O7 at intermediate temperatures, J. Electrochem. Soc., 2006, 153(8), A1604, DOI:10.1149/1.2210669.
- Y. Li, T. Kunitake, Y. Aoki and E. Muto, Efficient, anhydrous proton-conducting nanofilms of Y-doped zirconium pyrophosphate at intermediate temperatures, Adv. Mater., 2008, 20(12), 2398–2404, DOI:10.1016/j.ssi.2008.01.046.
- X. Sun, S. Wang, Z. Wang, X. Ye, T. Wen and F. Huang, Proton conductivity of CeP2O7 for intermediate temperature fuel cells, Solid State Ionics, 2008, 179(21–26), 1138–1141, DOI:10.1016/j.ssi.2008.01.046.
- M. Nagao, T. Kamiya, P. Heo, A. Tomita, T. Hibino and M. Sano, Proton conduction in In3+-doped SnP2O7 at intermediate temperatures, J. Electrochem. Soc., 2006, 153(8), A1604, DOI:10.1149/1.2210669.
- S.-H. Lee, S.-T. Lee, D.-H. Lee, S.-M. Lee, S.-S. Han and S.-K. Lim, CsHSO4/TiP2O7 composite membrane for high temperature (>150 °C) proton exchange membrane fuel cells, Int. J. Hydrogen Energy, 2015, 40(37), 12770–12775, DOI:10.1016/j.ijhydene.2015.07.128.
- T. Hibino, K. Kobayashi, M. Nagao and S. Kawasaki, High-temperature supercapacitor with a proton-conducting metal pyrophosphate electrolyte, Sci. Rep., 2015, 5(1), 1–7, DOI:10.1038/srep07903.
- A. I. Baranov, B. V. Merinov, A. V. Tregubchenko, V. P. Khiznichenko, L. A. Shuvalov and N. M. Schagina, Fast proton transport in crystals with a dynamically disordered hydrogen bond network, Solid State Ionics, 1989, 36(3–4), 279–282, 10.1039/B604311A.
- S. M. Haile, R. I. C. Calum, K. Sasaki, D. A. Boysen and T. Uda, Solid acid proton conductors: from laboratory curiosities to fuel cell electrolytes, Faraday Discuss., 2007, 134, 17–39, 10.1039/B604311A.
- A. Ishikawa, H. Maekawa, T. Yamamura, Y. Kawakita, K. Shibata and M. Kawai, Proton dynamics of CsH2PO4 studied by quasi-elastic neutron scattering and PFG-NMR, Solid State Ionics, 2008, 179(40), 2345–2349, DOI:10.1016/j.ssi.2008.10.002.
- V. G. Ponomareva, A. K. Konstantin, R. D. Gus’kov, I. N. Bagryantseva, N. F. Uvarov and V. P. Fedin, Proton conducting hybrid compounds based on CsH5 (PO4)2 metal–organic coordination frameworks, Solid State Ionics, 2019, 343, 115084, DOI:10.1016/j.ssi.2019.115084.
- M. Yamada, D. Li, I. Honma and H. Zhou, A self-ordered, crystalline glass, mesoporous nanocomposite with high proton conductivity of 2 × 10−2 S cm−1 at intermediate temperature, J. Am. Chem. Soc., 2005, 127(38), 13092–13093, DOI:10.1021/ja052914x.
- M. Nogami, H. Matsushita, Y. Goto and T. Kasuga, A Sol–Gel-Derived Glass as a Fuel Cell Electrolyte, Adv. Mater., 2000, 12(18), 1370–1372, DOI:10.1002/1521-4095(200009)12:18<1370::AID-ADMA1370>3.0.CO;2-1.
- R. Marschall, J. Rathouský and M. Wark, Ordered functionalized silica materials with high proton conductivity, Chem. Mater., 2007, 19(26), 6401–6407, DOI:10.1021/cm071164i.
- P. Choi, H. J. Nikhil, T. M. Thampan and R. Datta, Consideration of thermodynamic, transport, and mechanical properties in the design of polymer electrolyte membranes for higher temperature fuel cell operation, J. Polym. Sci., Part B: Polym. Phys., 2006, 44(16), 2183–2200, DOI:10.1002/polb.20858.
- M. Vinothkannan, A. R. Kim, S. Ramakrishnan, Y.-T. Yu and D. J. Yoo, Advanced Nafion nanocomposite membrane embedded with unzipped and functionalized graphite nanofibers for high-temperature hydrogen-air fuel cell system: The impact of filler on power density, chemical durability and hydrogen permeability of membrane, Composites, Part B, 2021, 215, 108828, DOI:10.1016/j.compositesb.2021.108828.
- O. Nakamura, I. Ogino and T. Kodama, Temperature and humidity ranges of some hydrates of high-proton-conductive dodecamolybdophosphoric acid and dodecatungstophosphoric acid crystals under an atmosphere of hydrogen or either oxygen or air, Solid State Ionics, 1981, 3, 347–351, DOI:10.1016/0167-2738(81)90111-9.
- K.-D. Kreuer, Fast proton transport in solids, J. Mol. Struct., 1988, 177, 265–276, DOI:10.1016/0022-2860(88)80093-0.
- O. Nakamura, T. Kodama, I. Ogino and Y. Miyake, High-conductivity solid proton conductors: Dodecamolybdophosphoric acid and dodecatungstophosphoric acid crystals, Chem. Lett., 1979,(1), 17–18, DOI:10.1246/cl.1979.17.
- U. B. Mioč, K. M. Slobodan, D. Malović, V. Stamenković, P. Colomban, M. M. Mitrović and R. Dimitrijević., Structure and proton conductivity of 12-tungstophosphoric acid doped silica, Solid State Ionics, 1997, 97(1–4), 239–246, DOI:10.1016/S0167-2738(97)00089-1.
- N. Lakshmi and S. Chandra, Proton conducting composites of heteropolyacid hydrates (phosphomolybdic and phosphotungstic acids) dispersed with insulating Al2O3, Phys. Status Solidi A, 2001, 186(3), 383–399, DOI:10.1002/1521-396X(200108)186:3<383::AID-PSSA383>3.0.CO;2-9.
- A. T. Howe and G. S. Mark, Studies of layered uranium(VI) compounds. I. High proton conductivity in polycrystalline hydrogen uranyl phosphate tetrahydrate, J. Solid State Chem., 1979, 28(3), 345–361, DOI:10.1016/0022-4596(79)90085-9.
- G. Alberti, M. Casciola, U. Costantino, A. Peraio and T. Rega, Proton-conducting solid dispersions of silica and zirconium phosphate pyrophosphate, J. Mater. Chem., 1995, 5(11), 1809–1812, 10.1039/JM9950501809.
- G. Alberti and M. Casciola, Layered metalIV phosphonates, a large class of inorgano-organic proton conductors, Solid State Ionics, 1997, 97(1–4), 177–186, DOI:10.1016/S0167-2738(97)00070-2.
- G. Alberti, L. Boccali, M. Casciola, L. Massinelli and E. Montoneri, Protonic conductivity of layered zirconium phosphonates containing—SO3H groups. III. Preparation and characterization of γ-zirconium sulfoaryl phosphonates, Solid State Ionics, 1996, 84(1–2), 97–104, DOI:10.1016/S0167-2738(96)83011-6.
- M. G. Shilton and T. H. Arthur, Rapid H+ conductivity in hydrogen uranyl phosphate-A solid H+ electrolyte, Mater. Res. Bull., 1977, 12(7), 701–706, DOI:10.1016/0025-5408(77)90129-5.
- N. Miura and N. Yamazoe, Development of new chemical sensors based on low-temperature proton conductors, Solid State Ionics, 1992, 53, 975–982, DOI:10.1016/0167-2738(92)90279-X.
- Y.-M. Li, M. Hibino, M. Miyayama and T. Kudo, Proton Conductivity of Some Hydrated Compounds at Intermediate Temperature up to 150 °C under High Water Vapor Pressure, Electrochemistry, 2001, 69(1), 2–5, DOI:10.5796/electrochemistry.69.2.
- K. Zhu, T. Wu, S. Sun, W. van den Bergh, M. Stefik and K. Huang, Synergistic H+/Zn2+ dual ion insertion mechanism in high-capacity and ultra-stable hydrated VO2 cathode for aqueous Zn-ion batteries, Energy Storage Mater., 2020, 29, 60–70, DOI:10.1016/j.ensm.2020.03.030.
- M. C. Verbraeken, E. Suard and J. T. S. Irvine, Structural and electrical properties of calcium and strontium hydrides, J. Mater. Chem., 2009, 19(18), 2766–2770, 10.1039/B820173K.
- B. Zhu and X. Liu, LiF–CaH2 alumina electrolytes for intermediate temperature fuel cell applications, Electrochem. Commun., 2000, 2(1), 10–14, DOI:10.1016/S1388-2481(99)00139-3.
- M. C. Verbraeken, C. Cheung, E. Suard and J. T. S. Irvine, High H− ionic conductivity in barium hydride, Nat. Mater., 2015, 14(1), 95–100, DOI:10.1038/nmat4136.
- H. Ubukata, F. Takeiri, K. Shitara, C. Tassel, T. Saito, T. Kamiyama, T. Broux, A. Kuwabara, G. Kobayashi and H. Kageyama, Anion ordering enables fast H− conduction at low temperatures, Sci. Adv., 2021, 7(23), eabf7883, DOI:10.1126/sciadv.abf7883.
- T. Hirose, T. Mishina, N. Matsui, K. Suzuki, T. Saito, T. Kamiyama, M. Hirayama and R. Kanno, Fast Hydride-Ion Conduction in Perovskite Hydrides AE LiH3, ACS Appl. Energy Mater., 2022, 5(3), 2968–2974, DOI:10.1021/acsaem.1c03633.
- G. Kobayashi, Y. Hinuma, S. Matsuoka, A. Watanabe, M. Iqbal, M. Hirayama, M. Yonemura, T. Kamiyama, I. Tanaka and R. Kanno, Pure H-conduction in oxyhydrides, Science, 2016, 351(6279), 1314–1317, DOI:10.1126/science.aac9185.
- F. Takeiri, A. Watanabe, A. Kuwabara, H. Nawaz, N. I. P. Ayu, M. Yonemura, R. Kanno and G. Kobayashi, Ba2ScHO3: H-conductive layered oxyhydride with H-site selectivity, Inorg. Chem., 2019, 58(7), 4431–4436, DOI:10.1021/acs.inorgchem.8b03593.
- C. A. Bridges, F. Fernandez-Alonso, J. P. Goff and M. J. Rosseinsky, Observation of Hydride Mobility in the Transition-Metal Oxide Hydride LaSrCoO3HO7, Adv. Mater., 2006, 18(24), 3304–3308, DOI:10.1002/adma.200601266.
- F. Takeiri, A. Watanabe, K. Okamoto, D. Bresser, S. Lyonnard, B. Frick and A. Ali,
et al., Hydride-ion-conducting K2NiF4-type Ba–Li oxyhydride solid electrolyte, Nat. Mater., 2022, 21(3), 325–330, DOI:10.1038/s41563-021-01175-0.
- Y. Kobayashi, O. J. Hernandez, T. Sakaguchi, T. Yajima, T. Roisnel, Y. Tsujimoto and M. Morita,
et al., An oxyhydride of BaTiO3 exhibiting hydride exchange and electronic conductivity, Nat. Mater., 2012, 11(6), 507–511, DOI:10.1038/nmat3302.
- K. Fukui, S. Iimura, T. Tada, S. Fujitsu, M. Sasase, H. Tamatsukuri, T. Honda, K. Ikeda, T. Otomo and H. Hosono, Characteristic fast H− ion conduction in oxygen-substituted lanthanum hydride, Nat. Commun., 2019, 10(1), 1–8, DOI:10.1038/s41467-019-10492-7.
- F. Altorfer, W. Bührer, B. Winkler, G. Coddens, R. Essmann and H. Jacobs, H-jump diffusion in barium-nitride-hydride Ba2NH, Solid State Ionics, 1994, 70, 272–277, DOI:10.1016/0167-2738(94)90322-0.
- B. Wegner, R. Essmann, J. Bock, H. Jacobs and P. Fischer, Structure and H-ionic-conductivity of barium hydride nitride, Ba2H (D) N, Eur. J. Solid State Inorg. Chem., 1992, 29(6), 1217–1227 CAS.
- R. Chemnitzer, G. Auffermann, D. M. Többens and R. Kniep., (Sr2N) H: Untersuchungen zur Redox-Intercalation von Wasserstoff in Sr2N, Z. Anorg. Allg. Chem., 2005, 631(10), 1813–1817, DOI:10.1002/zaac.200500105.
- A. J. E. Rowberg and C. G. Van de Walle, Hydride conductivity in nitride hydrides, ACS Appl. Energy Mater., 2021, 4(6), 6348–6355, DOI:10.1021/acsaem.1c01208.
- R. Leveque, M. Zanne, D. Vergnat-Grandjean and J.-F. Brice, Etude de la conducivité ionique des hydrurofluorures CaF2−xHx, J. Solid State Chem., 1980, 33(2), 233–243, DOI:10.1016/0022-4596(80)90124-3.
- M. Kamata, M. Atsushi and E. Takao, Synthesis and Ionic Conductivity of LiBaF3−xHx, Denki Kagaku Oyobi Kogyo Butsuri Kagaku, 1998, 66(4), 443–445, DOI:10.5796/kogyobutsurikagaku.66.443.
|
This journal is © The Royal Society of Chemistry 2023 |
Click here to see how this site uses Cookies. View our privacy policy here.