DOI:
10.1039/D2GC02783F
(Paper)
Green Chem., 2023,
25, 183-195
Improving methanol assimilation in Yarrowia lipolytica via systematic metabolic engineering combined with compartmentalization†
Received
26th July 2022
, Accepted 4th October 2022
First published on 11th October 2022
Abstract
Renewable methanol is an attractive non-food feedstock that can replace sugar-based materials for biochemical production. However, the metabolic construction of a synthetic methylotroph to achieve methanol conversion for cell growth and chemical production remains a challenge due to toxic intermediates and complicated metabolic pathways. Herein, we rationally engineered the oleaginous yeast Yarrowia lipolytica for the achievement of methanol utilization and value-added chemicals (mainly succinic acid) production. The artificially constructed methylotrophic Y. lipolytica showed 3.40 g L−1 consumption of methanol when synergistically using methanol and xylose as carbon sources. Then, through the rewiring of the peroxisomal Xu5P recycle pathway to improve precursor supply, the recombinant strain showed enhanced cell growth and methanol utilization. Transcriptome analysis revealed that the downregulation of key genes in the glycolysis pathway and upregulation of key genes in the amino acid biosynthesis pathway and TCA cycle can rebalance the methylotrophic metabolism. Furthermore, the heat shock protein 70 (hsp70) was found to enable the recombinant Y. lipolytica to efficiently use methanol for cell growth and metabolism. After the inactivation of the succinate dehydrogenase subunit 5 (sdh5), 0.92 g L−1 of succinic acid can be synthesized from methanol on its own, demonstrating its great potential for bioconversion of methanol into valuable chemicals. This study represents a successful example for the biological conversion of methanol to value-added chemicals.
Introduction
Metabolic construction of microbial cell factories to utilize renewable feedstocks for the production of valuable chemicals is the main goal of green biomanufacturing. Currently, biomanufacturing heavily relies on accessible sugars as the dominant feedstocks, leading to the undesirable competition in food and feed industries. Thus, the exploitation of non-food and low-cost alternative feedstocks to replace edible sugars is crucial for the sustainable development of biomanufacturing.1 The broadly available and energy-rich C1 liquid compound, methanol, has been considered as a promising candidate owing to its low-cost, renewability and increased availability.2,3 Methanol can be readily produced from methane, the major component of natural gas with an estimated worldwide recoverable amount of over 7 quadrillion cubic ft.4 Meanwhile, the progress in methanol synthesis via CO2 hydrogenation has also opened up a pathway for renewable methanol production.5 Compared to traditional carbon sources, such as glucose and glycerol, methanol is more reductive and contains higher energy, which will be beneficial for cell growth and biosynthesis.6 Thus, the exploitation of microbial cell factories to utilize methanol for bioproduction is desirable.
Methylotrophs are microorganisms possessing the capability to utilize one-carbon compounds, including methanol and methane, as sole carbon and energy sources for microbial growth. Although natural methylotrophs have been comprehensively exploited to synthesize single-cell proteins, poly-3-hydroxybutyrate and amino acids from methanol, their industrialization is still not economically feasible due to slow growth kinetics and the relative lack in the understanding of the physiology, metabolism and regulation of methylotrophs, as well as the lack of efficient genetic engineering tools.7–9 Advancements in synthetic biology and metabolic engineering in the last three decades have offered greater opportunities to genetically engineer microbial cell factories for methanol-based bio-manufacturing. Specifically, the genetic modification of non-methylotrophic model organisms into synthetic methylotrophs has emerged as a promising alternative, which can bypass the technical drawbacks inherent in attempting to engineer natural methylotrophs.10 For example, our group has successfully constructed methylotrophic Escherichia coli, which was able to produce succinic acid from methanol in glucose-based medium. Furthermore, the reducing power generated from methanol benefited the synthesis of succinic acid.11 More recently, a breakthrough has been achieved in which the synthetic methylotrophic E. coli could grow on methanol on its own through the rational design of its metabolic pathway and laboratory evolution.12
Although the process of exploiting bacteria as synthetic methylotrophs was ahead of yeasts, previous investigations have shown that yeasts provide a number of advantageous properties over bacteria in biotechnological production.13 For example, yeasts usually possess more efficient protein expression systems, protein assembly and folding, and post-translational modifications.37,38 Another striking advantage of yeasts is that they usually have a higher methanol tolerance than bacteria, indicating their greater potential for methanol metabolism.13 Furthermore, they can tolerate a lower pH, which is especially beneficial for organic or amino acid production. In addition, the presence of peroxisomes will protect cells from harmful metabolic intermediates of methanol, especially formaldehyde.13 In this regard, baker's yeast (Saccharomyces cerevisiae), and some nonconventional yeasts with specific features of interest, such as high secretion ability or lipophilic compound accumulation (Yarrowia lipolytica), are regarded as desirable chassis cells for the construction of synthetic methylotrophs.16–18,39S. cerevisiae had first been constructed as a methylotrophic yeast by equipping a methanol assimilation module, which led to 0.26 g L−1 pyruvate using methanol and the yeast extract as a co-substrate.14,15 More recently, Y. lipolytica has been engineered for methanol assimilation by construction of a chimeric assimilation pathway, rewiring of the native precursor supply pathway and laboratory evolution.19 However, the focuses of these studies were more on the utilization of methanol rather than on value-added chemicals production. Thus, it is critical to exploit efficient synthetic methylotrophic yeasts as cell factories for the production of valuable platform chemicals from methanol.
In this study, synthetic methylotrophic Y. lipolytica was constructed to convert methanol into biomass components and value-added chemicals by rational metabolic engineering. Specifically, we showed that the introduction of the methanol assimilation module from Pichia pastoris into the peroxisomes of Y. lipolytica enabled the engineered strain to utilize methanol as the sole substrate with the assistance of yeast extract, resulting in superior growth with improved biomass yields. Then, the xylose utilization module and peroxisomal Xu5p recycle pathway were constructed to generate formaldehyde acceptor Xu5P. Transcriptome analysis was subsequently conducted to reveal the global regulation responses of cells to the improved methanol utilization. Finally, to demonstrate the usefulness of this strain for metabolite production, we also knocked out succinate dehydrogenase for the accumulation of succinic acid by the engineered Y. lipolytica strain.
Materials and methods
Strains and plasmids
All strains used in this work are shown in Table S1.†Y. lipolytica Po1f (ATCC #MYA-2613), a leucine and uracil auxotroph strain, was used as the host for DNA assembly and integration. E. coli DH5α was used as a host for recombinant DNA manipulation and plasmid maintenance. Plasmids required for gene overexpression were constructed using vectors 113 and pINA1312 as backbones. The genes PpAox (NCBI-GeneID: 8201223), PpCat (NCBI-GeneID: 8198267), PpDak (NCBI-GeneID: 8200330) and PpDas (NCBI-GeneID: 8199663) involved in the methanol metabolism module were amplified and fused with peroxisomal targeting signals SKL at the C-terminus by PCR from the genome of Pichia pastoris. The genes MgXyr (NCBI-GeneID: 5127438), MgXdh (GenBank: MF139767.1), and MgXyk (NCBI-GeneID: 5124047) involved in the xylose metabolism module were amplified by PCR from the genome of Meyerozyma guilliermondii. The genes PpFba2p (NCBI-GeneID: 8196585), PpTal2p (NCBI-GeneID: 8198238), and PpFbp1p (NCBI-GeneID:8199670) involved in peroxisomal Xu5p regeneration were amplified and fused with peroxisomal targeting signals SKL at the C-terminus by PCR from the genome of Pichia pastoris. The strains and plasmids used in this study are summarized in ESI Table 1.†
Media and culture conditions
E. coli DH5α harboring synthetic plasmids were grown in LB medium (5 g L−1 of yeast extract, 10 g L−1 of tryptone, and 10 g L−1 of NaCl) at 37 °C and 200 rpm for 12 h. 50 mg L−1 of kanamycin or ampicillin was added to the LB broth as required. P01f and its derivatives were cultivated in glass test tubes at 30 °C and 200 rpm in YPD medium or synthetic defined (SD) medium. YPD media contained 20 g L−1 of glucose, 10 g L−1 of yeast extract and 20 g L−1 of peptone. The SD medium used in this study contained 2 g L−1 (NH4)2SO4, 1 g L−1 NaCl, 0.5 g L−1 MgCl2·6H2O, 0.3 g L−1 NH4Cl, 0.3 g L−1 KCl, 0.2 g L−1 KH2PO4, 0.015 g L−1 CaCl2·6H2O, 1.5 mg L−1 FeCl2·4H2O, 0.2 mg L−1 CoCl2·6H2O, 0.1 mg L−1 MnCl2·4H2O, 0.07 mg L−1 ZnCl2, 0.003 mg L−1 H3BO3, 0.036 mg L−1 Na2MoO4·2H2O, 0.024 mg L−1 NiCl2·6H2O and 0.002 mg L−1 CuCl2·2H2O. When the SD medium was used, different concentrations of xylose and methanol were selectively added as carbon sources. For the whole-cell catalysis experiment, the cells were first grown in sugar-based medium and then collected and transferred into methanol-based medium. For starvation-enabled adaptive evolution (SAE), strain Yl-002 was cultivated in glucose first and allowed to sit in carbon-depleted medium for 14 days. After that, starved cells were washed with sterile water, and then transferred into 50 mL of fresh YNB medium with 20 g L−1 of xylose. The plates of YSC media consisted of 20 g L−1 glucose, 0.79 g L−1 CSM supplement (MP Biomedicals), 6.7 g L−1 yeast nitrogen base without amino acids (YNB) and 20 g L−1 agar. The YSC without leucine (YSC-Leu) and YSC without uracil (YSC-Ura) plates were prepared in the same way using 0.69 g L−1 CSM-leucine or 0.77 g L−1 CSM-uracil in place of CSM, respectively. YSC plates containing different concentrations of hygromycin (0.3 g L−1 as a gradient) were used for the screening of mutants with hygromycin as the selection marker.
For bioconversion of methanol to SA, cells were first grown in SD medium with 20 g L−1 xylose and shaken at 30 °C and 200 rpm for 16–20 h until the optical density (OD600) reached ∼5. Then, yeast cells were washed twice with water and harvested by centrifugation at 8000 rpm for 10 min, and transferred into SD medium with 20 g L−1 methanol for fermentation. When evaluating the effect of different nitrogen sources on SA production of the strain Yl-005, 2 g L−1 YNB, peptone, and the yeast extract were added to the SD medium containing 20 g L−1 methanol, respectively, and the culture method remains the same.
DNA manipulation
All DNA manipulation was performed according to the standard molecular cloning protocols and all restriction enzymes were purchased from Takara. The constructed plasmids were linearized with the NotI restriction enzyme and purified by gel recovery, and then transformed into the genome of Y. lipolytica cells using the Frozen-EZ yeast transformation II™ kit. YSC-Leu, YSC-Ura and YSC + hygromycin plates were used to screen corresponding transformants. To disrupt the TCA cycle for SA accumulation, sdh5 (encoding succinate dehydrogenase, accession number of NC_006072) in the chromosome of Y. lipolytica was knocked out by using the CRISPR-Cas9 system in which the hygromycin was used as a selection marker.36 The positive transformants were further verified by gene sequencing (Genescript, Nanjing, China).
Analytical methods
Cell growth was determined by measuring OD600 using an AOE INSTRUMENTS-UV1800 spectrophotometer. The residual methanol and xylose concentrations were determined by using a biosensor analyzer and high-performance liquid chromatography (HPLC). Briefly, the culture was harvested and centrifuged at 12
000g for 10 min at room temperature and the supernatant was used for methanol and xylose determination. For methanol analysis, a convenient and simple method was adopted. The SIEMAN Biosensors Analyzer S-10 equipped with an alcohol oxidase membrane was used. Xylose concentrations were measured by HPLC. A UVD 170U ultraviolet detector at a wavelength of 215 nm, and an ion exchange chromatographic column (Bio Rad Aminex HPX-87H column, USA) were used. The products were eluted at 55 °C with 0.25 mM H2SO4 as the mobile phase at a flow rate of 0.5 mL min−1.
Transcriptome analysis
The strain Yl-004 was cultivated in SD medium supplemented with and without 20 g L−1 of methanol and 20 g L−1 of xylose. Cultivation conditions were 30 °C and 200 rpm. Cells at the middle exponential phase (∼72 h) were collected for RNA isolation. The OD260/OD280 of RNA extracts floated within a reasonable range between 1.89 and 2.12 and the concentration was also sufficient for subsequent experiments. RNA preparation, library construction and sequencing on Illumina HiSeq were achieved using Personalbio (Shanghai, China). The FASTQC software (v.0.10.1) was used to assess the quality of raw sequence reads. Based on the quality assessment results, low-quality readings and bases from both ends of raw Illumina readings were removed and trimmed using the NGSQC Toolkit (v.2.3.3). After the high-quality readings were aligned against the Po1f reference genome using the BWA alignment software (v.0.7.17), the mapping results were sorted and indexed using SAM tools software (v.1.9). Raw read counts from BAM files were obtained using HTSeq (v.0.11.2) software. The raw-count table was further processed with the DESeq function of the DeSeq2 package (v.1.18.1) to obtain gene expression data. Genes with a false discovery rate (FDR) <0.05 and log
2 (fold change) >0.5 or <−0.5 were considered to be differentially expressed. Pearson's linear correlation coefficients between variables were calculated using the R package ‘stats’ and plotted using ‘corrplot’. Principal component analysis was performed using the ‘stats’ package and plotted using the ‘ggord’ package.
Real-time quantitative PCR
Yl-003 cells at 72 h were selected for relative gene expression levels, at which point the cells were still in the methanol induction phase. Takara RNAiso Plus (Takara) was used to extract total RNA. The extracted mRNA was reversely transcribed to cDNA using the HiScript II Q RT SuperMix for qPCR kit. The reaction system was prepared according to the ChamQ™ SYBR qPCR master mix (code: Q341-02/03) and examined on Step One Plus, a fluorescence quantitative PCR instrument. All experiments were performed in biological triplicate in 96-well plates using an ABI 7500 real-time PCR system (Applied Biosystems). The β-actin gene was used to standardize the mRNA levels. The mRNA level of target genes was calculated via the 2−ΔΔCT method.
Determination of 13C-labeled intracellular metabolites
For 13C-labeled intracellular metabolite analysis, Yl-003 and Yl-004 were cultivated in SD medium supplemented with 20 g L−1 of 13C-methanol (99% atom enrichment, Sigma-Aldrich, MO, USA) and 20 g L−1 of xylose for 72 h. The sample cells were quickly injected into −40 °C 60% (v/v) methanol for 30 s to quench the cellular metabolism. The mixture was centrifuged at 4000g for 5 min at 4 °C and the supernatant was removed. The resulting cell pellets were washed with Milli-Q water twice to remove residual media, and then centrifuged at 4000g for 5 min at 4 °C and the supernatant was removed. The treated cells were ground under liquid nitrogen and 50 mg was accurately weighed into a 1.5 ml centrifuge tube. Additionally, 1 mL of −40 °C 50% (v/v) methanol was added into the centrifuge tube and then frozen and thawed three times with liquid nitrogen. The mixture was centrifuged at 12
000g for 3 min at 4 °C and 200 μL of the supernatant was transferred into a new centrifuge tube. The sample was quickly frozen in liquid nitrogen and then placed in a vacuum freeze dryer to freeze-dry. For derivatization, 50 μL of 20 mg mL−1 methoxylamine hydrochloride in pyridine was added to the samples, which were then vortexed occasionally and incubated at 40 °C for 80 min. Then, the samples were mixed with 80 μL of N-methyl-N-tri-methylsilyltrifluoroacetamide, vortexed occasionally and incubated at 40 °C for 80 min. After derivatization, the samples were centrifuged at 12
000g for 10 min, and the supernatant was transferred to new GC vials.
The derivatized amino acid samples were analyzed by GC/Q-TOF-MS using an Agilent 7890 A GC coupled with a 7200 Accurate-Mass Q-TOF (Agilent Technologies, Germany). A DB-5MS ultra inert column (30 m × 0.25 mm, 0.25 μm film thickness) was used (Agilent Technologies, USA). The sample (1 μL) was injected into the GC in splitless mode. The oven temperature was programmed as follows: 60 °C for 1 min, 8 °C min−1 to 132 °C, 2 °C min−1 to 150 °C, 5 °C min−1 to 185 °C, and 10 °C min−1 to 325 °C, 5 min hold. Mass spectra of the amino acids were in the mass range of 50–650 m/z at an acquisition rate of 5 spectra per s. The temperatures of the ion source and transfer line were 250 °C and 290 °C, respectively. Electron ionization was carried out at 70 eV. Agilent Mass Hunter Qualitative Analysis software was used for peak detection and mass spectral deconvolution. The annotation of amino acids was performed via matching their mass fragmentation patterns with those in the National Institute of Standards and Technology mass spectral library (match factor >80%). 13C-labeling was determined from the measured mass isotopomer data as described above.
Results and discussion
Metabolic construction of a methanol assimilation module in Y. lipolytica
In natural methylotrophic yeasts, methanol is first oxidized to formaldehyde and hydrogen peroxide (H2O2) by peroxisomal unspecific alcohol oxidase (Aox). Then toxic H2O2 is converted to oxygen and water by catalase (Cat). Meanwhile, formaldehyde is assimilated into glycerol aldehyde-3-phosphate (GAP) and dihydroxyacetone (DHA) in combination with xylulose 5-phosphate (Xu5P) by dihydroxyacetone synthase (Das) (Fig. 1A). Although the presence of native alcohol dehydrogenases in Y. lipolytica can oxidize methanol to formaldehyde, the lack of downstream assimilation pathways hinders the further utilization of methanol. Thus, the whole methanol assimilation module was first introduced and anchored into the peroxisomes of Y. lipolytica to make it capable of utilizing methanol. For simplification, we named the metabolically engineered Y. lipolytica as strain Yl-001. Subcellular localization of proteins into the peroxisomes using the SKL signal peptide was experimentally confirmed through the fused green fluorescent protein (gfp). The strain carrying gfp fused with SKL showed a punctate fluorescence pattern (Fig. S1†), characteristic of peroxisomes.20
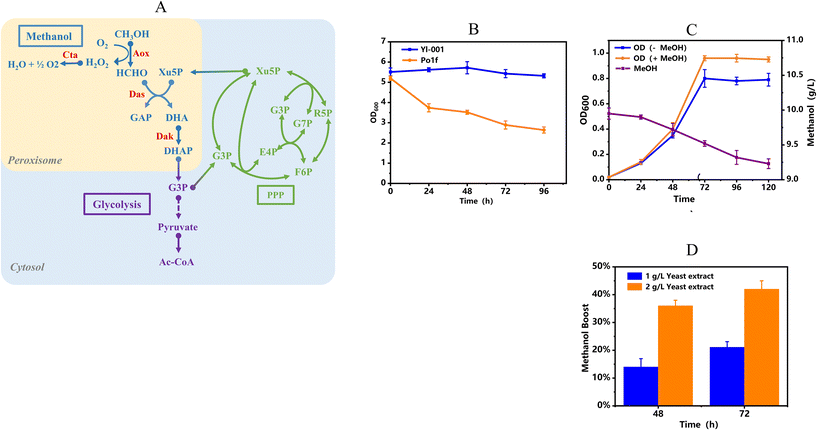 |
| Fig. 1 Metabolic construction of the methanol assimilation pathway in Y. lipolytica. (A) Metabolic construction of the methanol assimilation pathway in Y. lipolytica. Heterologous enzymes involved in methanol assimilation are highlighted in red. Dotted lines indicate multiple reactions. (B) Cell growth of enrichment-cultivated strains Po1f and Yl-001 using methanol as the sole carbon source. (C) Cell growth and methanol consumption of strain Yl-001 in SD medium supplemented with 1 g L−1 of yeast extract, with or without 10 g L−1 of methanol. The consumption of methanol has been corrected by subtracting the evaporation of methanol in the empty control. (D) Methanol boosting represents the increase of OD600 in the presence of methanol compared to the absence of methanol. SD medium supplemented with 1 g L−1 or 2 g L−1 of yeast extract was used as the control. The data represent the means ± standard deviations (n = 3). | |
Unsurprisingly, strain Yl-001 with the only methanol assimilation module did not exhibit appreciable methanol-dependent growth, which was consistent with prior reports of synthetic methylotrophy.14,19 To further verify the effectiveness of the methanol utilization module in Y. lipolytica, the strategy of whole-cell catalysis was adopted. As shown in Fig. 1B, the OD600 value of wild strain Po1f was decreased by 50.4% after being transformed into the SD medium containing 10 g L−1 of methanol, which may be attributed to the incapability of methanol utilization and toxic effects of methanol. In contrast, the OD600 value of the recombinant strain Yl-001 was not affected and even increased slightly after 48 h owing to the presence of the methanol assimilation pathway.
In our previous studies, it was found that yeast extract could facilitate the cell growth of the recombinant methylotroph from methanol and improve the methanol utilization rate.11,14 To further investigate the effect of methanol on the cell growth of strain Yl-001, yeast extract was added into SD medium. Indeed, yeast extract in SD medium promoted the cell growth of strain Yl-001 from methanol. For example, when 1 g L−1 of yeast extract was co-fed with 10 g L−1 of methanol, the OD600 value of strain Yl-001 reached its highest value 0.96 at 72 h, which showed a remarkable improvement compared to that from the medium without methanol (Fig. 1C). To improve this phenotype, the yeast extract was increased to 2 g L−1, and cultures were transferred five times in an attempt to acclimate strain Yl-001 to this medium. As shown in Fig. 1D, the presence of methanol in the medium resulted in a 42.0% boost of methanol utilization compared to the yeast extract-only medium, confirming the increase of methanol-specific growth in liquid medium containing yeast extract.
The sole overexpression of the methanol assimilation pathway has been proved to have limited effects on methanol utilization.40 The reason could be that the natural methanol metabolism pathways often involve multistep cycles, such as the xylulose monophosphate (XuMP) pathway, where the metabolic flux must be accurately balanced between downstream metabolism and the regeneration of Xu5P for formaldehyde assimilation.41 Besides, the synthesis from methanol to amino acids has been reported to be a challenge.42 This explained why yeast extract, which is composed primarily of amino acids, was able to stimulate synthetic methanol metabolism in Y. lipolytica to support the improved cell growth.
Metabolic construction of the xylose utilization pathway in Y. lipolytica to provide the precursor of Xu5P
Among the dysfunctional regulation of the metabolic network in strain Yl-001, the most prominent contradiction is the insufficient supply of precursors, which act as C1-acceptors (Xu5p in yeasts and Ru5p in bacteria) in formaldehyde assimilation. The introduction of the auxiliary carbon source utilization pathway, especially xylose, has been repeatedly employed to stimulate methanol metabolism as reported in several studies, as it can be quickly metabolized to Xu5p or Ru5p.5,21 Although all essential genes for xylose metabolism existed in the genome of Y. lipolytica, no cell growth was observed when using xylose as the sole carbon source. Accordingly, strain Yl-002 was metabolically constructed by introducing an exogenous xylose uptake pathway into strain Yl-001 (Fig. 2A).22 However, initial benchmarking growth assays showed that strain Yl-002 was still unable to grow in minimal media with xylose as the only carbon source (ESI Fig. 1A†), which is also consistent with other reports.23 Furthermore, a starvation-enabled adaptive evolution (SAE) was adopted and enabled strain Yl-002 to utilize xylose for growth after 500 h. The evolved strain was accordingly isolated and designated as strain Yl-003, which showed cell growth on xylose (ESI Fig. 1B†). During the cultivation, the maximum OD600 of strain Yl-003 reached 5.9, and 9.6 g L−1 of xylose was consumed after 72 h (Fig. 2B). Besides, 3.8 g L−1 of citric acid was detected in the fermentation broth, indicating that xylose was metabolized into the central metabolic pathway (Fig. 2B).
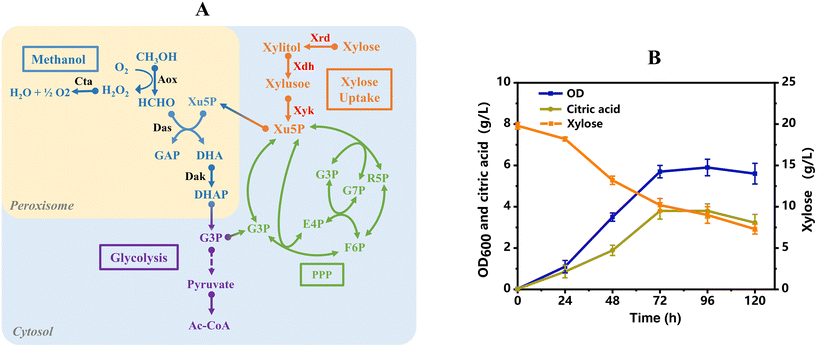 |
| Fig. 2 Engineering a xylose utilization pathway in strain Yl-001. (A) Xylose could be metabolized to Xu5P for formaldehyde assimilation. Heterologous enzymes involved in xylose metabolism are highlighted in red. Dotted lines indicate multiple reactions. (B) Fermentation result of strain Yl-003 using 20 g L−1 of xylose as the carbon source in SD medium. The data represent the means ± standard deviations (n = 3). | |
Co-utilization of xylose and methanol by the recombinant Y. lipolytica
To further evaluate the methanol and xylose co-utilization capability of strain Yl-003, the SD medium containing 20 g L−1 of xylose with and without 10 g L−1 methanol was used. It was found that the presence of methanol resulted in the improvement of cell growth, as the OD600 value was increased by 29.3% after 72 h (Fig. 3A). Besides, the specific xylose uptake rate was increased from 0.130 g L−1 h−1 to 0.175 g L−1 h−1 in the exponential phase with the supplementation of methanol (Fig. 3A). As mentioned above, the methanol metabolism requires the participation of Xu5P, which would accelerate the uptake of xylose. To verify this hypothesis, different concentrations of methanol were added into the SD medium with 20 g L−1 of xylose. As expected, the xylose uptake rate was indeed enhanced with the increase of methanol concentration from 0 to 20 g L−1 (Fig. 3B). In addition, the supplementation of 5 g L−1, 10 g L−1, 15 g L−1, and 20 g L−1 of methanol resulted in 1.18-, 1.25-, 1.21-and 1.34-fold increases in cell biomass compared to that with sole xylose (Fig. 3D). Higher concentrations of methanol also led to higher substrate consumption rates. As seen in Fig. 3C, the specific methanol consumption reached 3.4 g L−1 after 72 h with 20 g L−1 of methanol. It is worth noting that when the methanol concentration reached 25 g L−1, the cell growth was inhibited, which affected xylose and methanol uptake mainly due to the toxicity of methanol.
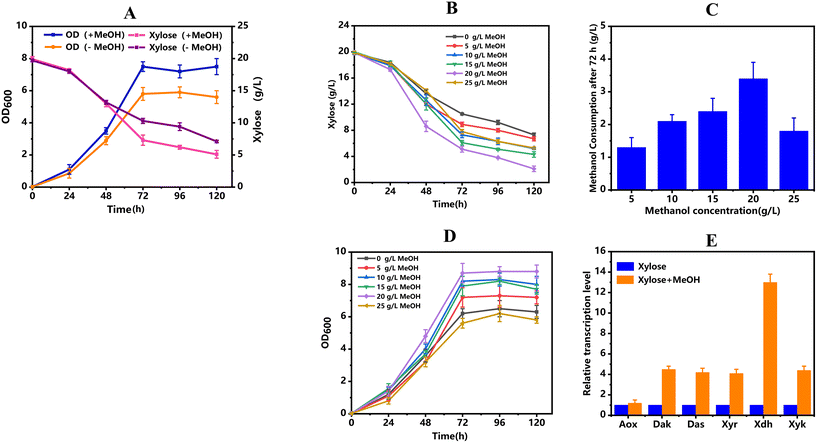 |
| Fig. 3 Co-utilization of methanol and xylose by the recombinant strains. (A) Cell growth and xylose uptake of strain Yl-003 cultivated in SD medium with and without 10 g L−1 of methanol and 20 g L−1 of xylose. (B) Xylose uptake of strain Yl-003 at different concentrations of methanol. (C) Methanol consumption of strain Yl-003 at 72 h at different concentrations of methanol. The consumption of methanol has been corrected by subtracting the evaporation of methanol in the empty control. (D) Cell growth of strain Yl-003 at different concentrations of methanol. (E) Relative transcription levels of Aox, Dak, Das, Xyr, Xdh and Xyk in Yl-003 using methanol and xylose as co-substrates compared to that using xylose as the sole carbon source. The data represent the means ± standard deviations (n = 3). | |
To elaborate on the underlying co-metabolism mechanism of methanol and xylose, the relative transcription levels of relevant genes were measured via RT-qPCR. As shown in Fig. 3E, when methanol and xylose were used as co-substrates, the relative transcription levels of most genes were significantly upregulated compared to those using xylose as the sole carbon source. For example, the transcription levels of genes das and dak occurring in the methanol utilization module were upregulated by 4.0- and 4.5-fold. Considering that das and dak are the key genes in the downstream metabolism of formaldehyde, it was hypothesized that formaldehyde metabolism to G3P is the rate-limiting step of methanol utilization in synthetic methylotrophic yeasts. Moreover, the overexpressed exogenous genes involved in xylose metabolism also showed obvious upregulation. This phenomenon was probably caused by the consumption of Xu5p, which can be co-metabolized with formaldehyde and can accelerate the xylose uptake, indicating that methanol and xylose metabolism may have coordinated effects. However, it was found that the co-utilization of xylose and methanol only enhanced cell growth and xylose consumption, but did not increase methanol consumption in previously reported methylotrophic Y. lipolytica.19 The discrepant result between the two studies may be due to the different requirements of precursors in the respective methanol assimilation module.
Rewiring of the Xu5P recycle pathway in the peroxisome
The increased methanol consumption caused by the introduction of xylose demonstrated the importance of precursors in methanol metabolism. However, an efficient synthetic methylotroph should be able to regenerate adequate precursors by itself, rather than relying on auxiliary carbon sources. It was reported that natural methylotrophic yeasts, such as P. pastoris, possess a specific Xu5p regeneration pathway in peroxisomes.24 This special pathway is similar to the non-oxidative PPP, which has not been found in non-methylotrophic yeasts such as S. cerevisiae and Y. lipolytica. Thus, in order to strengthen the methanol utilization capacity in strain Yl-003, the peroxisomal Xu5P recycle pathway was constructed by overexpressing relevant genes fused with the SKL signal peptide, resulting in the recombinant strain Yl-004 (Fig. 4A).
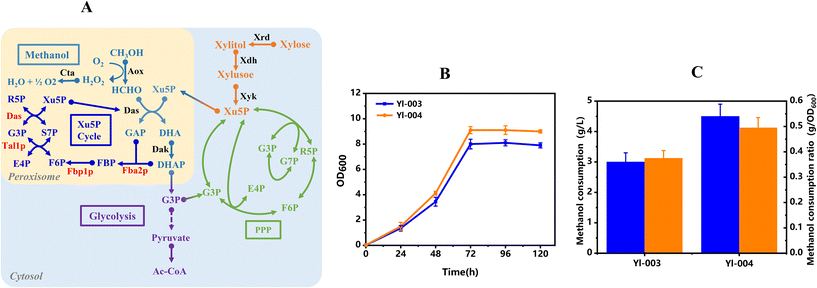 |
| Fig. 4 Metabolic engineering of peroxisomal non-oxidative PPP in strain Yl-003. (A) Metabolic construction of Xu5P recycle pathways in the peroxisome. Heterologous enzymes involved in Xu5P recycle pathways are highlighted in red. Dotted lines indicate multiple reactions. (B) Cell growth of strains Yl-003 and Yl-004 cultivated in SD medium supplemented with 20 g L−1 of xylose and 20 g L−1 of methanol. (C) Methanol consumption and methanol consumption ratio of strains Yl-003 and Yl-004 cultivated in SD medium supplemented with 20 g L−1 of xylose and 20 g L−1 of methanol. The consumption of methanol has been corrected by subtracting the evaporation of methanol in the empty control. The data represent the means ± standard deviations (n = 3). | |
Compared to strain Yl-003, strain Yl-004 showed significantly improved cell growth and methanol utilization when using 20 g L−1 of methanol and 20 g L−1 of xylose as co-substrates. The maximal OD600 value of 9.0 was achieved by strain Yl-004 at 72 h, and was 11.1% higher than that of strain Yl-003 (Fig. 4B). Besides, the increased methanol consumption from 3.0 g L−1 to 4.5 g L−1 at 72 h indicated that the peroxisomal Xu5P recycle pathway could provide more formaldehyde acceptors for methanol assimilation (Fig. 4C). In addition, the discrepancy in the methanol consumption rate demonstrated that higher methanol consumption was caused by improved methanol metabolism capability rather than increased cell biomass. These results demonstrated that the peroxisome-based compartmentalization strategy through the rewiring of the peroxisomal Xu5P recycle pathway could efficiently strengthen the ability of methanol assimilation in methylotrophic Y. lipolytica.
In synthetic methylotrophic bacteria, several strategies for Ru5P regeneration have been employed, such as weakening the glycolysis pathway and strengthening PPP and inactivating the downstream metabolism pathways of Ru5P.12,25,26 However, due to the slow process of exploiting yeasts as synthetic methylotrophs for methanol utilization, the Xu5P regeneration mechanism is still in its initial stages. Considering that strengthening PPP in the cytoplasm to generate more Xu5P may not be feasible for yeasts, in which the methanol assimilation mainly occurs in the peroxisome, an artificially designed peroxisomal non-oxidative PPP was accordingly constructed in strain Yl-003. Indeed, the recombinant strain Yl-004 showed a significant improvement in both cell growth and methanol utilization, indicating that peroxisomal non-oxidative PPP was helpful for the regeneration of Xu5P. This result may pave the way for the metabolic construction of autonomous synthetic methylotrophic yeasts through the regeneration of Xu5P.
13C-methanol incorporation into intracellular metabolites and biomass
To further investigate the effect of the rewired peroxisomal Xu5P recycle pathway on methanol assimilation, the 13C-methanol labeling approach was used. Initially, strains Yl-003 and Yl-004 were cultivated in SD medium with 20 g L−1 of xylose and 20 g L−1 of 13C-methanol as carbon sources. After 72 h, strain Yl-004 exhibited significantly improved average carbon labeling in the glycolytic and TCA cycle intermediates as well as in amino acids when compared to strain Yl-003 (Fig. 5A and B). For example, the 13.2% glycerol aldehyde-3-phosphate (3PG) pool in strain Yl-004 contained M + 1 labeling and 4.5% contained M + 2 labeling, indicating 7.4% average carbon labeling, which was increased by 57% compared to that in strain Yl-003. For phosphoenolpyruvic acid (PEP), 6.7% and 9.3% of the pool contained M + 1 labeling with 2.4% and 4.8% containing M + 2 labeling, while 0% and 2.1% of the pool contained M + 3 labeling in strains Yl-003 and Yl-004, respectively. The average carbon labeling in PEP at 72 h was increased from 3.8% in strain Yl-003 to 8.4% in strain Yl-004, representing a 2.2-fold improvement. Interestingly, the downstream intermediate of pyruvate (Pyr) in 3PG and PEP also showed an increase in the 13C labeling level from 6.9% in strain Yl-003 to 11.2% in strain Yl-004. Up to 15.7% of the pyruvate pool contained M + 1 labeling, and 8.9% contained M + 2 labeling in strain Yl-004.
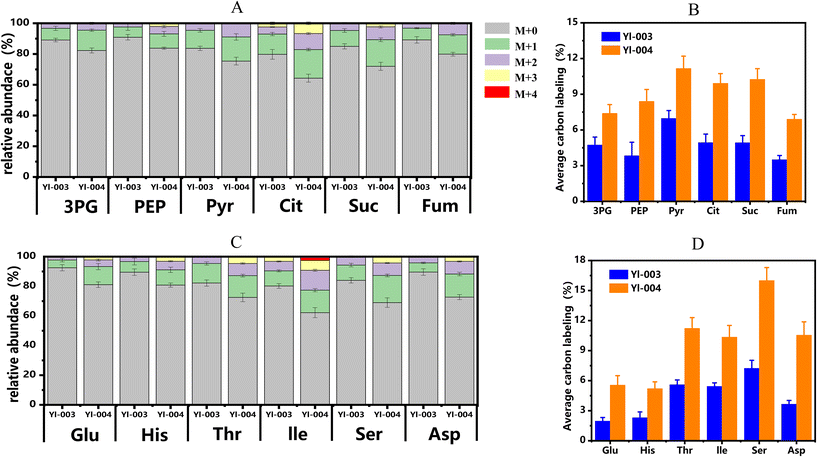 |
| Fig. 5
13C-labeling in intracellular metabolites and amino acids. (A) Relative abundance of glycolytic and TCA cycle intermediates at 72 h. (B) Average carbon labeling of glycolytic and TCA cycle intermediates at 72 h. (C) Relative abundance of amino acids at 72 h. (D) Average carbon labeling of amino acids at 72 h. The data represent the means ± standard deviations (n = 3). | |
Additionally, several organic acids crucial for cell growth and energy generation in the TCA cycle were labeled by carbons derived from 13C-methanol. For instance, it was found that 13.2%, 4.6% and 2.4% of the citric acid (Cit) pool contained M + 1, M + 2 and M + 3 labeling in strain Yl-003, while 18.6%, 10.4% and 6.7% of the Cit pool contained M + 1, M + 2 and M + 3 labeling in strain Yl-004, respectively. The average carbon labeling in Cit at 72 h was increased from 4.9% in strain Yl-003 to 9.9% in strain Yl-004 with a 2.0-fold improvement. The C4 TCA intermediate of succinic acid (Suc) was labeled by up to three 13C-methanol-derived carbons, whose average carbon labeling percentage was 10.3% in strain Yl-004, representing a 2.1-fold improvement compared to strain Yl-003, in which 4.9% average carbon labeling was observed. Similarly, the average carbon labeling in fumaric acid (Fum) at 72 h was increased from 3.5% in strain Yl-003 to 6.9% in strain Yl-004, leading to a 2.0-fold improvement. 7.6% and 12.6% of the pool contained M + 1 labeling, and 3.2% and 7.5% contained M + 2 labeling in strains Yl-003 and Yl-004, respectively. These results showed that the carbons from methanol passed through the glycolysis and TCA cycles, and were able to enter the metabolic pathways for organic acid production.
Amino acids are the basic building blocks of proteins, whose biosynthesis and catabolism play crucial roles in cell growth and metabolism. It was observed that serine showed higher average carbon labeling levels (7.2% in strain Yl-003 and 16.0% in strain Yl-004) than other amino acids (Fig. 5C and D). This may be attributed to the native reductive glycine pathway (rGlyP), as it could recirculate the carbon atoms lost by methanol dissimilation into the central carbon cycle. This result corresponded with the upregulation of the related genes involved in rGlyP (see detailed analysis in the section Transcriptome analysis to reveal metabolic regulation). In addition, the average carbon labeling in Glu, His, Ile and Asp at 72 h was increased from 2.0%, 2.3%, 5.4% and 3.7% in strain Yl-003 to 5.6%, 5.2%, 10.4% and 10.6% in strain Yl-004, respectively. Previous studies indicated that the biosynthesis of Thr from methanol was challenging in synthetic methylotrophic E. coli. However, owing to differences in metabolic networks, the synthetic methylotrophic Y. lipolytica showed passable average carbon labeling levels in Thr with 5.5% in strain Yl-003 and 11.2% in strain Yl-004. It was also found that 13.5% and 4.2% of the Thr pool contained M + 1 and M + 2 labeling in strain Yl-003, and 14.7%, 8.2% and 4.6% of the Thr pool contained M + 1, M + 2 and M + 3 labeling in strain Yl-004, respectively. These results in terms of 13C-methanol labeling analysis validated that the construction of the Xu5P recycle pathway in the peroxisome could efficiently improve the methanol assimilation in synthetic methylotrophic Y. lipolytica.
It has been reported that the conversion of methanol to several essential amino acids was severely hindered in E. coli, which was regarded as an important limitation to achieving autonomous methylotrophy.42 However, due to the differences in metabolic networks, the synthetic methylotrophic Y. lipolytica did not exhibit this defect, no matter whether in the previous study or this study.19 This phenomenon indicated that further engineering efforts and adaptive laboratory evolution (ALE) will be required to realize autonomous methylotrophy.
Transcriptome analysis to reveal metabolic regulation
To better elaborate the global regulation responses when cells were exposed to methanol, transcriptome analysis of strain Yl-004 grown in SD medium containing 20 g L−1 of xylose with or without 20 g L−1 of methanol was carried out. A total of 315 genes showed a significant variation at the genome-wide transcriptional level (223 upregulated and 92 downregulated genes). KEGG pathway enrichment analysis showed that differentially expressed genes mainly get involved in the biosynthesis and degradation of amino acids, carbohydrate metabolism, and energy metabolism (ESI Fig. 3†). To further analyze the differential gene expression, we first focused on genes directly related to xylose and methanol metabolism. The significant upregulation of xyr, xdh and dak corresponded well with the result of RT-PCR (Fig. 3E), further verifying the synergistic effect between methanol and xylose. In terms of the methanol dissimilation pathway, S-formylglutathione hydrolase (Frm) can catalyze the conversion of S-formylglutathione (S-FG) to formate, which was further metabolized to CO2, leading to the loss of carbon atoms. The upregulation of frm indicated that partial methanol was assigned to the dissimilation pathway in strain Yl-004. Knocking out frm was usually used as an effective strategy to improve the methanol assimilation in methylotrophic E. coli.8,12,22,24 Interestingly, the upregulation of shm1 (encoding serine hydroxymethyltransferase) and sda (encoding serine deaminase) involved in rGlyP showed potential to improve the methanol assimilation by recirculating the carbon atoms into the central carbon cycle. This strategy has also been successfully applied to E. coli and S. cerevisiae instead of knocking out frm.27,28 Specifically, the central metabolic and glycolysis pathways showed downregulation with the addition of methanol. This phenomenon has demonstrated that the blockage of glycolysis was regulated by cells in order to allocate more carbon sources for the regeneration of formaldehyde acceptors, and thus drive the methanol assimilation efficiency.29,30 The upregulation of the TCA cycle was expected to fulfill the bioenergetic and biosynthetic requirements of methylotrophic Y. lipolytica. It was speculated that the upregulation of succinyl-CoA synthetase, malate dehydrogenase and ATP citrate lyase involved in the TCA cycle was to provide GTP and balance the NAD+/NADH ratio for microbial growth. Previous studies on synthetic methylotrophs indicated that the downregulation or inactivation of malate dehydrogenase could promote the cells to maintain a high NAD+/NADH ratio, which was beneficial for methanol oxidation, since methanol dehydrogenases depend on NAD+ as the electron acceptor.30–32 However, several NAD-dependent dehydrogenases showed significant upregulation in strain Yl-004 with the addition of methanol, and consume NAD+ to produce NADH. This may be due to the methanol oxidation in methylotrophic Y. lipolytica depending on oxygen as the electron acceptor instead of NAD+.
As mentioned above, amino acids play crucial roles in cell growth. However, for synthetic methylotrophs, the biosynthesis of several amino acids from methanol is challenging, as their own metabolic network is not adapted to this unnatural or xenobiotic substrate.33 Several genes involved in amino acid metabolism were upregulated, suggesting that the amino acid biosynthesis was reprogramed to facilitate cell growth on methanol. For the biosynthesis of aromatic amino acids, E4P and phosphoenolpyruvate obtained from PPP and the glycolysis pathway are precursors of chorismite. In particular, the related genes of aroF, aroC, trp3, trpD, and trpA for the biosynthesis of L-tryptophan showed varying degrees of upregulation. In addition, the upstream genes responsible for the synthesis of branched-chain amino acids including L-leucine and L-valine were all enhanced, as well as the L-aspartate and L-glutamate family of amino acids (Fig. 6).
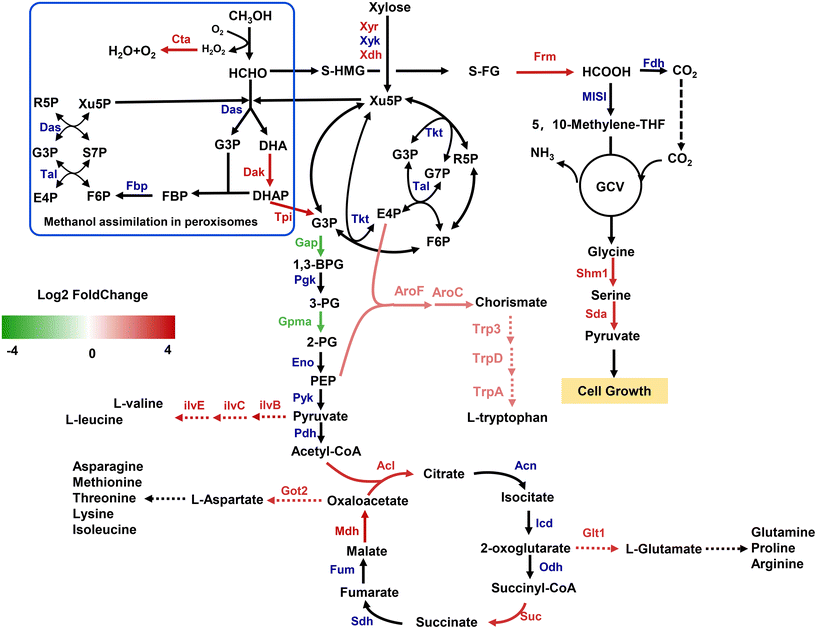 |
| Fig. 6 Transcriptome analysis of strain Yl-004 cultivated in SD medium supplemented with 20 g L−1 of xylose with or without 20 g L−1 of methanol. Only significant changes (log 2 (fold change) >0.5 or <−0.5, FDR <0.05) are shown. Upregulated and downregulated enzymes are indicated in red and green. | |
Promotion of Hsp70 on cell growth from methanol
Based on the functional analysis of differentially expressed genes for cell growth and metabolism, several significantly upregulated genes were selected for more detailed analysis including phosphate transporter (ptp), 6-phosphogluconate dehydrogenase (gnd), triose phosphate isomerase (tpi), SHM1 combined with sda and heat shock protein 70 (hsp70), which may have effects on methanol metabolism (Fig. 7A). Single overexpressed strains of the aforementioned genes were constructed in strain Yl-004 and tested by spotting strains in SD medium containing 20 g L−1 of methanol and 20 g L−1 of xylose.
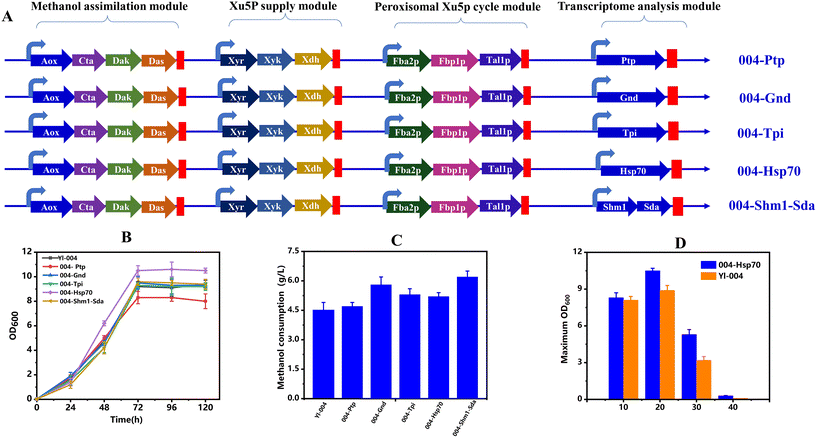 |
| Fig. 7 Performance of different strain Yl-004-overexpressed genes. (A) Schematic diagram of constructing recombinant strains based on strain Yl-004. (B) Cell growth of recombinant strains cultivated in SD medium supplemented with 20 g L−1 of xylose and 20 g L−1 of methanol. (C) Methanol consumption of recombinant strains cultivated in SD medium supplemented with 20 g L−1 of xylose and 20 g L−1 of methanol at 72 h. The methanol consumption has been corrected by subtracting the evaporation of methanol in the empty control. (D) Cell growth of strains Yl-004 and 004-Hsp70 cultivated in SD medium supplemented with 20 g L−1 of xylose and different concentrations of methanol. The data represent the means ± standard deviations (n = 3). | |
First, the ptp was selected to confirm whether it can help to transport Xu5P from cytoplasm into the peroxisome. Fermentation results showed that the overexpression of ptp cannot improve methanol consumption, and even caused the inhibition of cell growth. It is speculated that the overexpression of ptp broke the PPP balance, thus affecting cell growth. Besides, gnd was selected as it can promote the cell growth by converting formaldehyde to CO2 and NADH for detoxification. As shown in Fig. 7B and C, the methanol consumption of strain Yl-004 was increased, while the cell growth was not improved, which may be due to the overexpression of gnd driving more methanol into the dissimilation pathway. In addition, strengthening tpi and shm1 combined with sda showed a similar phenomenon to strengthening gnd, which is responsible for the driving of the carbon flow from the methanol assimilation module into the glycolysis pathway. It is worth mentioning that both cell growth and methanol consumption were increased by 14% and 15%, respectively, after the overexpression of hsp70, although hsp70 was not directly involved in methanol metabolism. It was reported that the presence of hsp70 could enable cells to better respond to the stresses of high alcohol concentrations and hydrogen peroxide, which are the by-products during methanol oxidation.28,33,34 Subsequently, in order to investigate the effect of hsp70 on methanol tolerance, we cultured strain 004-Hsp70 and Yl-004 in SD medium supplemented with 20 g L−1 of xylose and different concentrations of methanol (10, 20, 30 and 40 g L−1). It was found that the most obvious difference occurred when the methanol concentration was 30 g L−1, in which the maximum OD600 reached 5.3, which was improved by 1.66-fold compared to that of strain Yl-004 (Fig. 7D). These results demonstrated the importance of hsp70 in methanol utilization and cell growth.
Previously, the improvement of methanol tolerance mainly relied on the non-rational ALE, which allows the occurrence and selection of beneficial mutations.43 However, it was difficult for this non-rational strategy to provide clear guidance for other cases. Here, definite metabolic engineering by overexpressing hsp70 to improve methanol tolerance was implemented, which may be applicable for other strains. The stress factors, such as formaldehyde and hydrogen peroxide, generated in the process of methanol assimilation will increase the levels of denatured proteins, which can be harmful to the cell owing to changes in their biological activities.44 Therefore, HSPs such as hsp70 were synthesized to protect cells exposed to stress conditions.44 This may be the reason that the transcription level of hsp70 was greatly increased when methanol was added into the medium.
Bioconversion of methanol into succinic acid
To verify whether the engineered methylotrophic Y. lipolytica was capable of producing industrially relevant chemicals using methanol as the sole carbon source, succinic acid (SA) was selected as a proof-of-concept example, which represents one of the most important platform chemicals. SA is a key intermediate of the tricarboxylic acid (TCA) cycle, whose accumulation requires interfering in the activity of succinate dehydrogenase (Sdh).35 Thus, strain Yl-005 was constructed based on 004-Hsp70 by deleting the fifth subunit sdh5, which is required for sdh activity and stability for SA production (Fig. 8A). After 96 h of fermentation, 0.35 g L−1 of SA was produced by consuming 4.2 g L−1 of methanol as the sole carbon source (Fig. 8A and B). In contrast, no SA was detected in the medium without methanol.
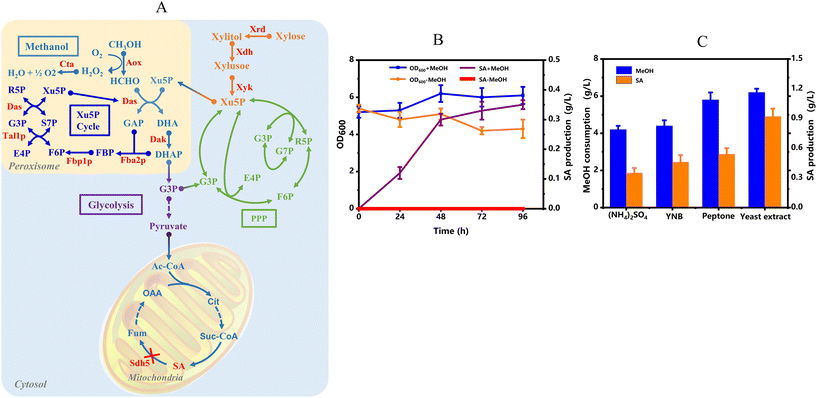 |
| Fig. 8 Production of succinic acid in Yl-005. (A) Scheme of the pathways related to SA production in Y. lipolytica. (B) Cell growth and SA production of Yl-005 in SD medium with or without 20 g L−1 methanol. (C) Effects of different nitrogen sources on the methanol consumption and SA production of strain Yl-005. The data represent the means ± standard deviations (n = 3). | |
Although we have finally achieved the goal of SA production from sole methanol, the titer was still too low. To further improve the carbon flux from methanol to SA, the effect of different additional nitrogen sources on the methanol assimilation and SA production of strain Yl-005 was studied. As shown in Fig. 8B, the additional YNB, peptone, and yeast extract can all effectively promote the methanol consumption compared to initial (NH4)2SO4, in which the yeast extract worked best. On the other hand, YNB and peptone were both able to slightly increase the SA production, and yeast extract can increase the production to 0.92 g L−1 by consuming 6.2 g L−1 of methanol as the sole carbon source. A previous study about methanol labeling experiments has demonstrated that the addition of 1 g L−1 yeast extract can increase the labeled succinate pool from 11% to 37%.9 Besides, an engineered methylotrophic strain of E. coli was observed with a 650% improvement in flavonoid production from M9 minimal medium containing 1 g L−1 of yeast extract and 60 mM methanol when compared to the strain containing the empty vector control in the methanol utilization module. In this study, by adding 2 g L−1 of yeast extract, methanol assimilation and SA production were both enhanced, resulting in a final titer of 0.92 g L−1 with methanol as the sole carbon source, demonstrating the methanol-driven biosynthesis of useful chemicals.
Conclusion
In conclusion, synthetic methylotrophic Y. lipolytica was rationally constructed in this study by employing methanol assimilation and xylose uptake pathways, rewiring the peroxisomal Xu5P recycle pathway and overexpressing hsp70. OD600 and methanol consumption of the recombinant strain could finally reach 10.5 and 5.2 g L−1 at 72 h when using 20 g L−1 methanol and 20 g L−1 xylose as co-substrates. Here, it was the first time highlighting the importance of rewiring the peroxisomal Xu5P recycle pathway for methanol assimilation in synthetic methylotrophic yeasts. In addition, methanol-driven SA production by the engineered Y. lipolytica strain was demonstrated. Given that the engineered Y. lipolytica strain presented in this study has the ability to convert methanol into biomass and high value chemicals, we hypothesize that further adaptive laboratory evolution will likely lead to a strain capable of growing on methanol as the sole carbon source. This work helps pave the way for the production of valuable platform chemicals from methanol by constructing synthetic methylotrophic yeast cell factories.
Author contributions
Shangjie Zhang, Jiangfeng Ma, Wenming Zhang, Fengxue Xin and Min Jiang conceived and initiated the project. Shangjie Zhang and Feng Guo wrote the paper and performed the genetic modifications. Qiao yang and Yujia Jiang performed the fermentation of the engineered strains. Shihui Yang, Tomohisa Hasunuma and Akihiko Kondo reviewed this paper and provided valuable suggestions. All authors read, edited, and approved the final manuscript.
Conflicts of interest
The authors declare no conflict of interest.
Acknowledgements
This work was supported by the National Key R&D Program of China (grant no. 2018YFA0901500), the National Natural Science Foundation of China (22078151 and 21978130), the Jiangsu Synergetic Innovation Center for Advanced Bio-Manufacture (XTD2215), and the Open Funding Project of the State Key Laboratory of Biocatalysis and Enzyme Engineering and Young Elite Scientist Sponsorship Program by CAST (YESS20200174).
References
- X. B. Liu, Biotechnol. Rep., 2020, 27, e00486 CrossRef.
- C. A. Haynes and R. Gonzalez, Nat. Chem. Biol., 2014, 10, 331–339 CrossRef CAS PubMed.
- C. A. Cotton, N. J. Claassens, S. Benito-Vaquerizo and A. Bar-Even, Curr. Opin. Biotechnol., 2020, 62, 168–180 CrossRef CAS.
- W. B. Whitaker, N. R. Sandoval, R. K. Bennett, A. G. Fast and E. T. Papoutsakis, Curr. Opin. Biotechnol., 2015, 33, 165–175 CrossRef CAS PubMed.
- A. Goeppert, M. Czaun, J. P. Jones, G. K. Surya Prakash and G. A. Olah, Chem. Soc. Rev., 2014, 43, 7995–8048 RSC.
- M. R. Antoniewicz, Curr. Opin. Biotechnol., 2019, 59, 165–174 CrossRef CAS.
- R. K. Bennett, L. M. Steinberg, W. Chen and E. T. Papoutsakis, Curr. Opin. Biotechnol., 2018, 50, 81–93 CrossRef CAS PubMed.
- J. D. Windass,
et al.
, Nature, 1980, 287, 396–401 CrossRef CAS.
- W. B. Whitaker, J. A. Jones, K. Bennett, J. E. Gonzalez and E. T. Papoutsakis, Metab. Eng., 2016, 39, 49–59 CrossRef.
- T. Zhu, T. Zhaoa, O. E. Bankef and Y. Li, Biotechnol. Adv., 2020, 39, 107467 CrossRef CAS.
- W. Zhang, T. Zhang, S. Meng, Z. Dai and J. Min, ACS Synth. Biol., 2018, 7, 2803–2811 CrossRef CAS PubMed.
- F. Y. H. Chen, H.-W. Jung, C.-Y. Tsuei and J. C. Liao, Cell, 2020, 182, 933–946 CrossRef CAS PubMed.
- J. T. Fabarius, V. Wegat, A. Roth and V. Sieber, Trends Biotechnol., 2020, 39, 348–358 CrossRef PubMed.
- Z. Dai, H. Gu, S. Zhang, F. Xin, W. Zhang, W. Dong, J. Ma, H. Jia and M. Jiang, Bioresour. Technol., 2017, 1407–1412 CrossRef CAS.
- M. I. Espinosa, R. A. Gonzalez-Garcia, K. Valgepea, M. R. Plan and T. C. Williams, Nat. Commun., 2020, 11, 5564 CrossRef CAS PubMed.
- S. Lajus,
et al.
, Front. Bioeng. Biotechnol, 2020, 8, 954 CrossRef PubMed.
- J. Hambalko,
et al.
, Front. Bioeng. Biotechnol., 2020, 8, 593419 CrossRef PubMed.
- Y. K. Park, T. Dulermo, R. Ledesma-Amaro and J. M. Nicaud, Biotechnol. Biofuels, 2018, 11, 158 CrossRef.
- G. K. Wang,
et al.
, ACS Synth. Biol., 2021, 10, 3537–3550 CrossRef CAS.
- T. W. Brown, V. I. Titorenko and R. A. Rachubinski, Mol. Biol. Cell, 2000, 11, 141–152 CrossRef CAS PubMed.
- C. Chen, F. Y. H. Chen, I. W. Bogorad, T. Wu, R. Zhang, A. S. Lee and J. C. Liao, Metab. Eng., 2018, 49, 257–266 CrossRef CAS PubMed.
- F. A. D. Silveira, T. A. R. Fernandes, C. R. S. Bragana, T. R. Balbino and W. B. D. Silveira, Int. Microbiol., 2019, 9(1), 1508 Search PubMed.
- H. Li and H. S. Alper, Biotechnol. J., 2016, 11, 1230–1240 CrossRef CAS.
- H. Fukuoka, T. Kawase, M. Oku, H. Yurimoto, Y. Sakai, T. Hayakawa and T. Nakagawa,
et al.
, J. Biosci. Bioeng., 2019, 128, 33–38 CrossRef CAS.
- M. W. Benjamin, R. K. Jason, R. Michael, V. H. Bob and S. Gregory, Nat. Commun., 2018, 9, 2387 CrossRef PubMed.
- J. Rohlhill, R. Jie, M. R. Antoniewicz and E. T. Papoutsakis, Metab. Eng., 2020, 57, 247–255 CrossRef CAS PubMed.
- B. Rkba,
et al.
, Metab. Eng., 2020, 61, 1–10 CrossRef PubMed.
- A. Müller,
et al.
, J. Bacteriol., 2013, 195, 2807–2816 CrossRef PubMed.
- Y. Hong and J. C. Liao, Nat. Commun., 2018, 9, 3992 CrossRef PubMed.
- S. Kim, S. N. Lindner, S. Aslan, O. Yishai and A. Bar-Even, Nat. Chem. Biol., 2020, 16, 538–545 CrossRef CAS PubMed.
- F. Meyer, P. Keller and J. Hartl,
et al.
, Nat. Commun., 2018, 9, 1508 CrossRef.
- B. M. Woolston, J. R. King, R. Michael, V. H. Bob and S. Gregory, Nat. Commun., 2018, 9, 2387 CrossRef PubMed.
- K. Zhao, M. Liu and R. Richard, J. Biol. Chem., 2005, 280, 17758–17768 CrossRef CAS PubMed.
- J. V. Price, L. Chen, W. B. Whitaker, E. Papoutsakis and W. Chen, Proc. Natl. Acad. Sci. U. S. A., 2016, 113, 12691–12696 CrossRef CAS.
- C. Gao, X. Yang, H. Wang, C. P. Rivero, C. Li and Z. Cui, Biotechnol. Biofuels, 2016, 9, 179 CrossRef PubMed.
- C. Schwartz, M. Shabbir-Hussain, M. Blenner and I. Wheeldon, ACS Synth. Biol., 2016, 5, 356–359 CrossRef CAS PubMed.
- J. L. Zhou,
et al.
, LWT – Food Sci. Technol., 2022, 163, 113390 CrossRef CAS.
- J. L. Zhou,
et al.
, ACS Sustainable Chem. Eng., 2020, 8(12), 4781–4791 CrossRef CAS.
- G. Guirimand, N. Kulagina, N. Papon, T. Hasunuma and V. Courdavault, Trends Biotechnol., 2021, 39, 488–504 CrossRef CAS PubMed.
- G. J. Gregory, R. K. Bennett and E. T. Papoutsakis, Metab. Eng., 2022, 71, 99–116 CrossRef CAS.
- B. M. Woolston, Trends Biotechnol., 2019, 37, 1273–1276 CrossRef CAS PubMed.
- J. E. Gonzalez, R. K. Bennett, E. T. Papoutsakis and M. R. Antoniewicz, Metab. Eng., 2018, 45, 67–74 CrossRef CAS PubMed.
- Y. Wang,
et al.
, Commun. Biol., 2020, 3, 217 CrossRef CAS PubMed.
- J. L. Rodrigues and L. R. Rodrigues, Trends Biotechnol., 2018, 36, 186–198 CrossRef CAS PubMed.
|
This journal is © The Royal Society of Chemistry 2023 |