DOI:
10.1039/D3FO03816E
(Paper)
Food Funct., 2023,
14, 10868-10881
In vitro digestion of high-lipid emulsions: towards a critical interpretation of lipolysis†
Received
8th September 2023
, Accepted 13th November 2023
First published on 21st November 2023
Abstract
Investigating the gastrointestinal fate of food emulsions is critical to unveil their nutritional relevance. To this end, the protocol standardized by COST INFOGEST 2.0 is meaningful for guiding in vitro digestion experiments. In contrast with studies addressing emulsions with low dispersed phase volume fraction (φ 0.05–0.1), we presently raise some points for a proper interpretation of the digestibility of emulsions with high lipid content using the pH-stat method. Oil-in-water high internal phase emulsions (HIPEs) were submitted to gastric pre-lipolysis with the addition of rabbit gastric lipase (RGE). Commercial mayonnaise (φ 0.76) was systematically diluted (φ 0.025, 0.05, 0.1, 0.15, 0.25, 0.4, and 0.76) to cover a wide range of enzyme-to-lipid ratios (8.5–0.3 U per µmol for RGE and 565.1–18.6 U per µmol for pancreatin, in the gastric and intestinal phases, respectively). Lipolysis was tracked either by fatty acid titration (NaOH titration) or completed by analysis of lipid classes and fatty acid composition. Gastric lipase resulted in substantial lipid hydrolysis, reaching 20 wt% at low lipid fractions (φ 0.025 and 0.05). Likewise, the kinetics and extent of lipolysis during intestinal digestion were modulated by the enzyme-to-substrate ratio. A logarithmic relationship between lipid hydrolysis and lipid concentration was observed, with a very limited extent at the highest lipid content (φ 0.76). A holistic interpretation relying on FFA titration and further evaluation of all lipolytic products appears of great relevance to capture the complexity of the effects involved. Overall, this work contributes to rationally and critically evaluating the outcomes of static in vitro experiments of lipid digestion.
1. Introduction
Studies dedicated to unveiling the digestive fate of foods are paramount for the development of healthy products. For instance, in the case of emulsions – widely recognized as generic models of food systems – colloidal and interfacial aspects can be engineered to attempt at controlling how they evolve during digestion while achieving functionalities such as increasing the bioavailability of bioactive compounds or modulating satiety.1–3
Among macronutrients, lipids have the highest caloric density and draw attention due to their biological functions as structural constituents of cell membranes, energy storehouses, and signaling molecules.4 Intake of dietary lipids can be either as bulk oils or fats (which will later be emulsified during digestion) or, mostly, in the form of colloidal structures (emulsified lipids). Even though lipids are generally combined with other macronutrients and with water in most foods and meals, there are specific situations where they represent a highly dominant part of an oral intake. For instance, if you were a child in the 20–30's, your mother would probably have given you a full teaspoon of cod liver oil, or, nowadays, it is easy to fall for diet indulgences such as mayonnaise or chocolate. It is noteworthy to mention that the consumption of high-lipid systems is very relevant considering their role as carriers for nutritionally relevant lipids and lipophilic bioactive compounds, or even as excipient foods.5
Lipid digestion is a process in which numerous substrate–enzyme interactions occur in a cascade of subsequent hydrolysis. The pathway from macronutrient to the formation of intermediate digestion products, which may be further converted into absorbable metabolites (e.g., sn-2 monoacylglycerols (MAGs), free fatty acids (FFAs)), is guided by multiple interfacial phenomena.6 Lipid digestion takes place during passage through the gastric and intestinal compartments. Although limited (10–25% of the total lipid digestion extent),7–9 gastric lipid digestion has been suggested to further stimulate intestinal lipid lipolysis, because of its effects on droplet disruption, solubilization of digestion products, hormone release, and adjustment of colipase–lipase binding capacity.
Lipolysis in the small intestine occurs with the adsorption of pancreatic lipase and colipase onto the lipid droplet surface.7,10,11 The action of these enzymes leads to the conversion of triacylglycerol (TAG) into FFAs and MAGs that remain at the interface.12,13 Finally, bile salts are essential for intestinal digestion as they promote the formation of colloidal structures after the displacement of lipolytic products, FFAs and MAGs, from the interface (mixed micelles), and thereby transport the solubilized lipolytic products into the intestinal mucosa for absorption.10,11
The well-recognized approaches to gaining insights into the mechanistic events of food digestion are the static or (semi)dynamic in vitro analyses.14 In a review by Duijsens et al. (2022)15 on strategic choices for in vitro food digestion methodologies, the authors acknowledge the recent shift towards more complex in vitro methodologies (i.e., dynamic). However, they also highlight that the simpler ones (static experiments) should not be overlooked, especially for the purposes of mechanistic understanding and/or samples screening. In this regard, the pH-stat method has been very popular because it is a quick and easy method of tracking overall digestion and its kinetics. This method is based on the fact that TAG hydrolysis leads to the formation of two FFAs and one sn-2 MAG. In theory, these two FFAs decrease the pH of the sample, triggering the counter-titration with NaOH. The pH-stat method does not provide details about the actual lipid digestion products formed.15,16 Nevertheless, the pH-stat method is still widely used, and the conditions generally follow the standardized INFOGEST 2.0 protocol. This international consensus has largely contributed to research on in vitro food digestion, as it has allowed the repeatability, representability and comparison of gathered data.15 The related INFOGEST action also guides the assessment of gastric and pancreatic lipases’ activities prior to in vitro digestion studies.17 In this approach, physiologically relevant conditions are established in a way to maintain constant meal to digestive fluid ratios and a constant pH for each digestion step.18,19 Moreover, it recommends the inclusion of a relevant substitute for human gastric lipase, i.e., do not neglect gastric lipolysis. The importance of gastric lipase inclusion in digestion assays relies on its unique combination of biochemical properties, such as activity over a wide pH range (2 to 7); high interfacial activity unleashing resistance to bile salts and penetration into phospholipid layers in TAG droplets’ surroundings; sn-3 stereospecificity; and resistance to pepsin.20
In general, when lipids are the targeted macronutrient, most of the studies published so far have been limited to emulsions with a relatively small lipid fraction (φ ≤ 0.1) (Fig. S1†). Only very few have focused on the role of lipid content on in vitro digestibility outputs. For instance, Martínez and co-workers (2022)21 investigated the digestion of cellulose ether-based emulsions with low- or high oil concentrations (from 5 to 47 wt%). Although cellulose retards lipolysis (attributed to the formation of thick, dense layers on the surface of oil droplets, preventing physical access of lipase molecules to lipids) compared to a control protein-based emulsion, the ratio of lipid mass fraction and NaOH titration volume was linear for cellulose-stabilized systems, whereas it looked logarithmic for the control. In another study, a decrease in lipid digestion was found by increasing the oil content from 10 to 20 wt%, in emulsions composed of corn oil, Tween 20 and β-carotene, which was associated with a limited amount of lipase, bile salts and calcium present in the medium.22
When shifting towards the evaluation of in vitro digestion of systems consisting mainly of oil, such as high internal phase O/W emulsions, oleogels or even bulk oils, some particularities should be considered. In fact, to accommodate large amounts of lipids, the INFOGEST protocol needs to be adapted. Sabet et al. (2022)23 proposed some modifications for the specific case of oleogels, such as: the amount of oleogel added to be digested (or oil per se) should be 20 times smaller than for emulsions with an oil mass fraction of 0.05 (250 mg instead of 5 g); the shear must be high enough to homogenize the sample well with bile salts and enzymes (emulsification); and the results are reliable when the percentage of FFAs released in oil (as a reference) is at least 80% at the end of the intestinal phase. Additionally, in the case of bulk oil containing a certain content of medium-chain fatty acids (MCFAs), gastric lipolysis has been reported to play a substantial role in MCFA digestion, impacting the extent of lipolysis in the GI tract.24
Based on these gaps in knowledge about the digestion patterns of high internal phase emulsions, we systematically evaluated in vitro static digestion of mayonnaise as a model of commercial food HIPE diluted to different initial oil mass fractions (φ 0.025 to 0.76) and included a human gastric lipase surrogate (RGE) to evaluate gastric preduodenal lipolysis. The kinetics of in vitro intestinal digestion was assessed by the pH-stat method. Moreover, quantification of the lipid species present at the endpoints of both the gastric and intestinal phases provided a comprehensive picture of lipid digestion.
2. Experimental
2.1 Materials
Commercial mayonnaise Amora® (Unilever, France) with a lipid content of 76 wt% was bought in the local market. Rabbit gastric extract (RGE, >15 U per mg, RGE 15-1G) was obtained from Lipolytech (France). α-Amylase from porcine pancreas (Type VI-B, ≥5 units per mg solid, A3176-1MU), pepsin from porcine gastric mucosa (lyophilized powder, ≥3200 units per mg protein, P6887-1G), pancreatin from porcine pancreas (8× USP, P7545-25G) and bile bovine (ox gall powder, dried unfractionated, B3883-100G) were purchased from Sigma-Aldrich (France). Likewise, chemicals for the preparation of simulated digestive fluids (potassium chloride, KCl; potassium phosphate monobasic, KH2PO4; sodium chloride, NaCl; magnesium chloride hexahydrate, MgCl2(H2O)6; calcium chloride, CaCl2(H2O)2) and enzyme inhibitors (Pefabloc SC-4-(2-aminoethyl)benzenesulfonyl fluoride and 4-bromophenylboronic acid were from Sigma-Aldrich (France)). Since we applied the pH-stat approach, sodium bicarbonate (NaHCO3) was replaced by NaCl at the same molar ratio to avoid the drawback of pH drift in open vessel.18
The solvents n-hexane, chloroform (ethanol-stabilized, HPLC) and isopropanol were purchased from Biosolve Chimie SARL (the Netherlands), and toluene and cyclohexane from Carlo Erba Reagents (France). Boron trifluoride–methanol solution (14%) was provided by Sigma-Aldrich (France). All the materials were used without further purification.
2.2 Methods
2.2.1
In vitro static digestion.
The in vitro digestion behavior of the samples was evaluated according to the relevant physiological conditions suggested in the standardized protocol of the international INFOGEST 2.0 network.18,19 Using a static approach means that all physiological parameters are adjusted at the beginning of each phase. Initially, pepsin activity (hemoglobin-based) of RGE and pepsin, as well as lipase activity (tributyrin-based) of RGE and pancreatin (added in the gastric and intestinal phases, respectively) were also determined according to the international consensus.18,19 The focus on lipid digestion makes it particularly critical to properly determine lipase activity, which is the subject of a dedicated INFOGEST recommendation.17 The experimental design for evaluating lipid digestion in oil-in-water (O/W) emulsions is presented in Fig. 1.
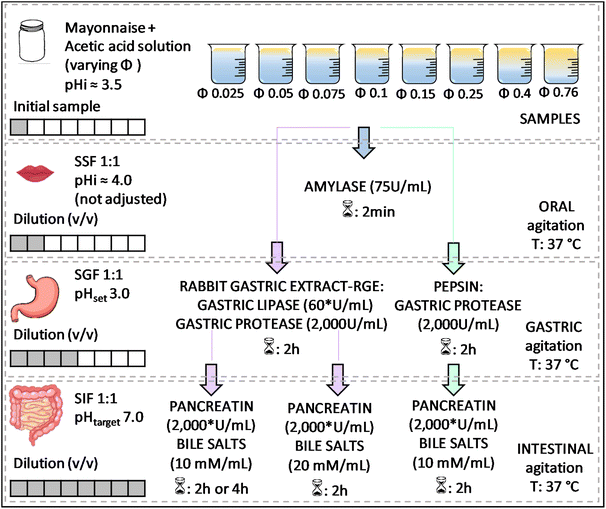 |
| Fig. 1 Experimental design for studying the effect of the lipid content of emulsions on their in vitro digestion. * Based on lipase activity in the final mixture. | |
A static approach was applied to evaluate the in vitro digestion of emulsions using a pH stat (Metrohm, 905 Titrando) coupled with a dosing system (Metrohm, dosino 20 mL or 2 mL for FFA titration or determination of enzymatic activity, respectively). Sequential digestion commands were set using Tiamo 2.5 software. Temperature was controlled by a double-walled beaker connected to a thermostated bath (Lauda Ecoline, RE 104). For the in vitro digestion of emulsions, different mayonnaise dilutions were tested to consider the effect of the initial lipid mass fraction (φ), from highly diluted to pure mayonnaise (φ 0.025; 0.05; 0.1; 0.15; 0.25; 0.4 and 0.76) by mixing mayonnaise with acetic acid 10 mM (pH adjusted to ≈3.44 with NaOH). Modification of the initial oil mass fraction led to further changes in enzyme-to-substrate ratio throughout digestion phases as shown in Table 1. Accordingly, with increasing initial lipid mass fraction, the lipase–lipid ratio in the gastric phase ranged from 8.5 to 0.3 U per µmol (RGE), whereas in the intestinal phase it ranged from 565.1 to 18.6 U per µmol for pancreatin.
Table 1 Lipid mass fraction and enzyme-to-substrate ratio found by screening different sample dilutions
Lipid mass fraction (φ) |
Oral |
Gastric |
Intestinal |
[Lipid] (µmol mL−1) |
[Lipid] (µmol mL−1) |
Enza : lipid ratio (U per µmol) |
[Lipid] (µmol mL−1) |
Enzb : lipid ratio (U per µmol) |
M
w lipid considered: 883 g mol−1 (average molecular weight based on the fatty acids composition determined by gas chromatography); lipolytic activity determined in the a rabbit gastric extract – RGE (gastric) and b pancreatin (intestinal), presented as U, where 1 U = 1 µmol butyric acid released per minute at 37 °C and at pH 8.0 for pancreatin and pH 5.5 for RGE. |
0.025 |
14.16 |
7.08 |
8.48 |
3.54 |
565.13 |
0.05 |
28.31 |
14.16 |
4.24 |
7.08 |
282.56 |
0.075 |
42.47 |
21.23 |
2.83 |
10.62 |
188.38 |
0.1 |
56.62 |
28.31 |
2.12 |
14.16 |
141.28 |
0.15 |
84.94 |
42.47 |
1.41 |
21.23 |
94.19 |
0.25 |
141.56 |
70.78 |
0.85 |
35.39 |
56.51 |
0.4 |
226.50 |
113.25 |
0.53 |
56.62 |
35.32 |
0.76 |
430.35 |
215.17 |
0.28 |
107.59 |
18.59 |
Initially, 5 mL of sample was added to the thermostated vessel. After temperature equilibration (37 °C), oral phase was simulated. As lipids were the target macronutrient, RGE was added to the gastric phase to deliver 60 U gastric lipase activity per mL of reactive medium and 2000 U per mL of pepsin activity. The added amount of pancreatin in the intestinal phase was calculated to reach 2000 U per mL based on lipase activity in the final mixture also meeting the required trypsin activity (100 U per mL). The entire volume of the digestive medium was collected at the end of oral–gastric or oral–gastric–intestinal phases to prevent any sampling error due, for example, to lack of digesta homogeneity. For the sake of further digestion inhibition, after the gastric step, the pH was raised to 7.0 and stored in an ice bath for temperature decrease. Likewise, at the end of intestinal phase, boronic acid and Pefabloc were added for lipase and protease inhibition, respectively. Then, samples were collected and lipid extraction of digesta and micellar phase separation, and microscopy were carried out right after the end of the in vitro simulation.
At a fixed lipid mass fraction (φ 0.25), a set of different conditions was tested to investigate intestinal lipolysis based on the premises that (i) increasing bile salt concentration (while still consistent with physiological conditions) may increase the rate and extent of lipid digestion by removing digestion products that accumulate at the oil–water interface; (ii) extending the duration of the intestinal phase to 4 h could allow for a greater extent of lipid digestion because of the longer time available for digestive interfacial events to take place, and (iii) adding pepsin instead of RGE keeping the same pepsin activity in the medium (2000 U per mL) in both cases during the gastric phase would limit the overall lipid digestion (Fig. 1).
2.2.2 Microstructure.
2.2.2.1 Bright field microscopy.
The microstructure of emulsions at different digestion steps and times was analyzed using an optical microscope (BX51, Olympus, Germany) at 10× magnification. Then a drop of sample was placed on a glass microscope slide, covered with a cover slip and analyzed at room temperature. A representative number of images (n ≥ 6) was evaluated.
2.2.2.2 Confocal laser scanning microscopy (CLSM).
The changes in emulsion microstructure throughout digestion were further examined using a confocal microscope (NIKON Eclipse-TE2000-A1si, France). For acquiring images, a ×20 lens (Plan APO 20×; numerical aperture: 0.75) and a water immersion lens ×40 (Plan APO 40×; numerical aperture: 1.25) were used. The fluorescent dyes Nile Red (lipids) and Alexa 488 (proteins) were dissolved in dimethylformamide (1 mg mL−1) and methanol (0.4 mg mL−1), respectively. Nile Red (maximum excitation 552 nm and maximum emission 636 nm) was excited by a diode laser. Alexa 488 was excited by an argon laser with a maximum excitation 490 nm and a maximum emission 525 nm. Fresh emulsions, oral, gastric and intestinal samples were prepared by mixing 1 mL of sample with 2 μL of Nile Red and 5 μL of Alexa 488. Images from fresh emulsion samples and after oral, gastric and intestinal simulation were taken with the NIS Element software. Images were analyzed using the FIJI software.
2.2.3 Lipid recovery.
The lipids from the digesta samples were immediately extracted at the end of the in vitro digestion protocol. Digesta sample (both gastric and intestinal) was added to a mixture of hexane and isopropanol (3
:
2 (v/v) ratio). Briefly, 1 mL of digesta was transferred to a tube containing 10 mL of hexane–isopropanol and 200 µL of 150 mM NaCl solution. For most samples, 50 µL of sulfuric acid 2.5 M was also added, which is helpful to ensure FFA protonation and hence complete lipid extraction.16 Digesta-solvent mixtures were vortexed for 1 min followed by centrifugation during 5 min at 1811g. Then, the upper hexane phase was collected. The bottom phase was washed with 6 mL of fresh hexane, and vortexing and centrifugation steps were repeated under the same conditions as above. Finally, both collected hexane phases were pooled to be evaporated under a stream of nitrogen for total removal of solvent. The lipid amount was determined by weight difference.
2.2.4 Lipolysis determination.
Lipolysis kinetics (Section 2.2.4.1) was assessed with successive quantification of FFAs by monitoring the NaOH release throughout the intestinal phase. At the end of 2 h of intestinal digestion simulation, the named overall lipolysis extent (Section 2.2.4.2) was assessed by different methodologies either considering the total NaOH volume required to compensate for all FFAs released (% molar of FFAs) during intestinal digestion (t = 2 h) or performed by the HPLC method (determining the lipid species present at both the gastric and intestinal endpoints). In the latter, the degree of lipolysis was estimated considering FFA versus the total acyl chains present by lipid class (TAGs, DAGs, MAGs and FFAs).
2.2.4.1 Kinetics & mathematical modeling.
The measurement of NaOH volume required to neutralize the FFAs being released during intestinal digestion was recorded every 2 s during the intestinal phase unveiling kinetics of lipolysis. The monitored NaOH volume was considered to obtain relative rates of lipolysis and converting these results to FFAs amount.25 This means that we make the hypothesis that the volume of NaOH added corresponds overwhelmingly to the neutralization of deprotonated free fatty acids (COO−) released by lipases, as commonly done with pH-stat in vitro digestion experiments.
The raw data resulting from the pH-stat experiments, both regarding the titration kinetics, and the final NaOH volume were sequentially corrected considering (i) blank digestion (sample: 10 mM acetic acid solution, pH 3.5–3.8) to correct for the endogenous lipid contributions of enzyme extracts and bile salts; and (ii) pH 7.0 as a starting point to count the cumulative increments of NaOH volume. This last correction was performed because during the analytical procedure, even if previously adjusted to pH 7.0, upon addition of pancreatic extract and bile salts, the starting pH recorded by the software was slightly reduced (≈6.8–6.9). To standardize the acquired data, therefore, the titration volume was counted from the moment the system reached pH 7.0 for the first time. Although this is systematic practice, it should be noted that it hampers initial information gathering (30–60 s), because during this period of pH adjustment, small amounts of FFAs can be released and are therefore not considered.
After applying the required correction, mathematical model fittings using KaleidaGraph (Synergy Software) to the kinetic datasets were obtained. After an initial screening with different models proposed by the literature, eqn (1) and (2) showed best fitting.6,26Eqn (2) model extends first order kinetics (eqn (1)) to two rate constants, assuming there are two mechanisms controlling lipolysis, where f is the fraction associated with k1.
| FFA(t) = FFAfinal − FFAfinal × exp(−k1 × t) | (1) |
|  | (2) |
where FFA
t represents the FFAs concentration at time
t in the digestion simulation. FFA
final is the FFAs concentration in the digesta at the final time considering that FFAs is the only lipid species estimated by the model. The rate constant
k1 in (
eqn (1)) is theoretically related to the experimental kinetic curve as
k1 = ln(2)/
t1/2 where
t1/2 is the time at half kinetics that is at FFA
final/2.
2.2.4.2 Lipolysis extent.
(i) NaOH titration – intestinal lipolysis.
As aforementioned, during the intestinal phase, pH drops mainly as a consequence of FFAs release upon the action of pancreatin. Thus, by titrating ionized FFAs with an alkaline solution (e.g., NaOH), pH is maintained at a constant value (e.g., pH 7.0). The number of moles of NaOH required to neutralize FFAs produced from TAGs, if the latter were completely digested (assuming the generation of 2 FFAs per TAG molecule by the action of lipase), is taken to calculate FFA release using eqn (3).25 |  | (3) |
where VNaOH is the volume (L) of NaOH added, MNaOH is the molarity of NaOH, Mw
lipid is the average molecular weight of triglyceride (883 g mol−1) based on the experimental fatty acid composition and Wlipid is the mass of triglyceride initially present in the reaction vessel (g).
(ii) HPLC – gastric and intestinal lipolysis.
The extracted lipids (at the endpoint of gastric and gastric–intestinal sequences) were dissolved in chloroform to reach a final concentration around 0.5–0.6 mg lipid per mL for HPLC injection. Lipid classes were separated, identified and quantified using a modular UltiMate 3000 RS HPLC (Dionex, France) paired with an evaporative light scattering detector (ELSD) Sedex 85 (Sedere S.A., Alfortville, France) as described elsewhere27 and analytical column was packed with a silica normal-phase Uptisphere CS Evolution SI: 150 mm × 4.6 mm, 2.6 μm (Interchim, Montluçon, France). Briefly, chromatographic separation was performed applying a linear gradient (t0: 0% B, t8
min: 50% B, t12
min: 100% B, and isocratic conditions with 100% B for 3 min) using chloroform and a mixture comprised of CH3OH/CHCl3/NH3OH (460/5/35; v/v/v), as eluent A and B, respectively. The percentage of each lipid class was calculated applying the calibration curve considering a quadratic fit between the peak area and the injected lipid amount. Afterwards this percentage was converted into molar concentration. Thus, for the lipolysis degree calculation, the molar fractions of FFAs, MAGs, DAGs and TAGs (with molecular weights of 281.7, 355.7, 619.3 and 883 g mol−1, respectively) were considered. The lipid classes molecular weights were based on the fatty acid composition of extracted rapeseed oil from the commercial mayonnaise.28 Typical chromatogram obtained for both standard and after gastric and intestinal step were presented on Fig. S3.†
The lipolysis degree (LD) was calculated as shown in eqn (4).29
|  | (4) |
where [FFA], [MAG], [DAG], and [TAG] are the concentrations in the digestive media (μmol mL
−1), and LD the lipolysis degree (% mol per mol total). In general, it is expected that the pancreatic lipases hydrolyzed the fatty acids at the
sn-1 and
sn-3 positions, leading to a theoretical maximum lipolysis degree of 67%.
2.2.5 Determination of absorbable lipids.
Assessment of bioaccessibility, namely lipids solubilized in mixed micelles that are potentially absorbable, was carried out considering the fatty acid composition of the micellar phase compared to that of the lipid extracted from the total intestinal digesta medium.
The fatty acids composition and concentration in the lipids recovered from the digesta at the endpoint of intestinal phase (i.e., total lipid extracts) were analyzed as FA methyl esters (FAMEs) after transmethylation with boron trifluoride–methanol solution.30 FAMEs separation and quantification were carried out as previously described.31 Briefly, FAMEs analysis was performed using a gas chromatograph (PerkinElmer, Clarus 680) equipped with a flame ionization and a capillary column (DB 225, 30 m × 0.32 mm, film thickness 0.25 µm, Agilent, J&W). Results were taken considering the relative proportion of the total area of peaks according to the fatty acid methyl esters (%). FA amount was assessed in one mL of digesta (mg mL−1) using heptadecanoid acid (C17:0) as internal standard.
The micellar phase (made up of mixed micelles and vesicles) containing lipolytic products (i.e., FFA and MAG) was recovered from the intestinal digesta by centrifugation as previously described.32 At the end of the intestinal step, a 1.5 mL aliquot of digesta was centrifuged in Eppendorf tubes for 45 min at 21 × 103g (Micro Ultracentrifuge Hettich Universal 320R, Germany). The micellar fraction, which corresponds to the aqueous phase below the upper oil layer (volume varying from 50 to 500 μL according to the lipid content), was collected using an eVol® XR Dispensing Electronic System Syringes (Thermo Scientific™). An aliquot of the micellar phase, typically 100 μL, was directly methylated without prior lipid extraction.33 Briefly, 100 μL internal standard (heptadecanoic acid: ≈1 mg mL−1 in acetone/methanol (2
:
1 (v/v))), 2 mL of methanol and 400 μL sulfuric acid were mixed to the sampled micellar phase. The tubes were shaken for 30 s and heated at 100 °C for 60 min. After cooling to room temperature, 1 mL of water and then 2 mL of cyclohexane were added. After mixing, FAMEs were recovered in the upper cyclohexane phase (2 mL). The FAs analysis (≈1 mL) was performed in the same way as for total lipids extract previously described, then accounting for FAs in the micellar lipid phase (FFAs + MAGs). Thus, the so-called lipid bioaccessibility was defined as the proportion of each fatty acid in the micellar phase (lipids solubilized in mixed micelles) in relation to the lipids recovered from the total intestinal digesta.
2.3 Data analysis
At least two independent digestion experiments were performed for each lipid fraction. HPLC and GC analyses were carried out at least in duplicate for each independent repetition of the process (n = 4). Mean values are reported with standard deviations. Analysis of variance (ANOVA) was carried out using Minitab 16.1.1 software from Minitab Inc. (USA). Statistically significant differences (p < 0.05) among treatments were assessed pairwise by Tukey test.
3. Results & discussion
3.1 Microstructure
Different samples (i.e., whole mayonnaise or the acetic acid solution dilution series) were evaluated during in vitro digestion following the INFOGEST 2.0 protocol. Fig. S2† (bright field) and Fig. 2 (confocal) depict colloidal morphology of the initial samples (“meals”) and after passing through the oral, gastric and intestinal phases, respectively. Overall, the initial degree of droplet packing (related to sample dilution) drives the pattern for further digestion phases (i.e., gastric and intestinal). In contrast to the decrease in mean lipid droplet size as intestinal digestion progresses, some larger droplets were also observed, indicating incomplete digestion. Highly diluted samples (lipid-poor condition) had few droplets remaining after the intestinal phase. These large droplets that remain visible at the end of intestinal digestion (Fig. S2† and Fig. 2) may be related to the creaming effect taking place at higher lipid contents.34 Conversely, the initially undiluted sample had highly packed droplets and ended up with many undigested droplets. A progressive increase in undigested droplets remaining at the endpoint of the intestinal phase was observed from the lowest to the highest oil mass fractions.
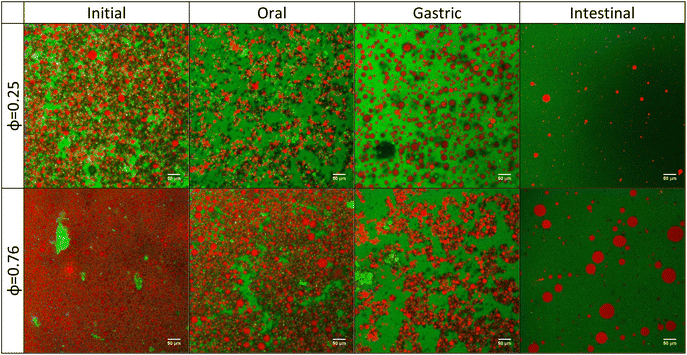 |
| Fig. 2 CLSM images of emulsions with 0.25- and 0.76-oil mass fraction (i) before starting digestion and after (ii) oral, (iii) gastric and (iv) intestinal phases, respectively. The red color depicts the oil droplets and the green color depicts the proteins. The scale bar is 50 µm. (For interpretation of the color reference in this figure legend, the reader is referred to the web version of this manuscript.) | |
Emulsions underwent some flocculation and coalescence after the oral and gastric phases, which may be driven by egg yolk proteins (at their isoelectric point) present in the commercial mayonnaise (Fig. 2). In the stomach phase, the high ionic strength (≈100 mM) combined with a rapid acidification of the medium (from pH 7.0 in the oral phase to pH 3.0 in the early gastric phase) may be responsible for such changes. During pH adjustment, the pH corresponding to the isoelectric point of the proteins (around 4.6)35 was reached. In this condition, the protein layer no longer has sufficient repulsive forces to prevent droplet aggregation.36 In addition, these large droplets can be formed due to protein hydrolysis at the droplet interface by pepsin.37
3.2 Lipid digestion
3.2.1 Gastric phase.
This study focuses on lipids as the main target macronutrient. Therefore, rabbit gastric lipase (RGE) was added to the gastric content as a substitute for human gastric lipase.18 After the gastric phase, the proportion of lipid classes (Fig. 3 – column chart) was monitored and the extent of lipid hydrolysis (Fig. 3 – secondary y-axis) expressed as a percentage using eqn (4).
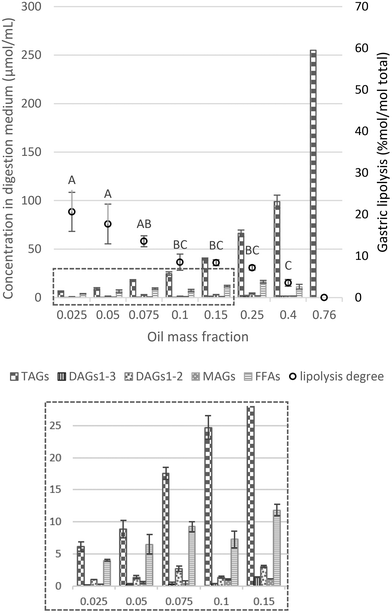 |
| Fig. 3 Proportions of lipid species (concentration of TAGs, DAGs1–3, DAGs1–2, MAGs and FFAs) in the digestive medium (μmol mL−1) and lipolysis degree (% mol per mol total) assessed by HPLC analysis (eqn (4)) as a function of the oil mass fraction in the initial emulsion and at the endpoint of the gastric phase. Different capital letters show a significant difference in gastric lipolysis (% mol per mol total) among lipid mass fractions at p < 0.05 according to the Tukey test (n = 4). The insert at the bottom shows a magnification of the values of the concentration of the lipid species in the digestion medium (μmol mL−1), for oil mass fractions from 0.025 to 0.15. | |
In our experimental approach, gastric lipolysis reached about 20% at lower lipid mass fraction tested (φ 0.025, 0.05 and 0.075) with no significant difference. On the other hand, it was very limited (less than 5%) at high lipid content (φ 0.4–0.76). These results point out to the fact that lipid hydrolysis in the gastric phase is modulated by the initial lipid mass fraction oriented by the [RGE]/[TAG] ratio (Table 1), in which the amount of gastric lipase available to the low-lipid system is much higher than for the high-lipid emulsion. In fact, it has been reported that a range of 5–37% of the total lipids can be cleaved during gastric passage.38 Moreover, when looking at the lipid classes profile, TAGs were mostly hydrolyzed into DAGs and FFAs rather than MAGs (Fig. 3 and S4a†), consistent with RGE activity that is a potential substitute to human gastric lipase, in line with previous findings in the literature.38
Another factor that may play a role is the reduction of the surface area caused by coalescence/flocculation of the droplets observed in the micrographs. This has been shown to impact hydrolysis in the interfacial layer for emulsions stabilized by proteins, digestible carbohydrates (e.g., modified starches), and/or digestible lipids (e.g., lecithin, mono- and diglycerides).39 Therefore, reduction in the available surface area due to coalescence/flocculation should be considered as an additional factor for the reduction of lipolysis at higher lipid mass fraction.
Overall, the extent of gastric lipolysis (% mol per mol total) showed a decay with increasing lipid fraction as there was a decrease in the lipase-to-substrate ratio. In the range of φ 0.025–0.1, it is likely that, as under physiological conditions, lipase concentration is sufficient or even excessive in relation to the substrate, allowing a non-limiting lipase binding on the surface of lipid droplets. For higher oil mass fractions (φ 0.1–0.76), in addition to the [RGE]/[TAG] ratio (Table 1), another relevant point is that gastric lipolysis can be hindered by the protonated FFAs arising from digestion, which progressively accumulate on the surface of lipid droplets, thereby hampering the action of gastric lipase.5 Some authors previously proposed that gastric lipase would be blocked due to the formation of FFA clusters on the droplet surface, limiting the access of lipase to the TAG core.11 Such accumulation of FFA clusters would occur due to the absence of bile salts in the stomach, necessary for the removal of lipid digestion products from the surface of the droplets.5
3.2.2 Intestinal phase.
3.2.2.1 Effect of the initial oil mass fraction on the extent of intestinal lipolysis.
After the gastric phase, emulsions were exposed to in vitro intestinal physiological conditions. Quantification of lipolysis products by HPLC was used to determine the proportion of TAGs, DAGs, MAGs and FFAs and the final extent of lipolysis in the intestinal digesta (Fig. 4). Compared to gastric digesta, the concentration of total lipids (μmol mL−1) was lower due to the dilution (1
:
1) with the intestinal fluid (Fig. 1), and the continuous addition of NaOH to maintain the pH at 7.0 during intestinal phase. Overall, compared to the gastric phase, TAG and FFA profiles were largely altered with a much more marked predominance of FFAs at the end of the intestinal phase (Fig. 4 and S4b†). This is due to the fact that TAG hydrolysis occurred in the duodenal phase, because of the synergistic actions of gastric and colipase-dependent pancreatic lipases, and the presence of bile salts that allow the removal of FFAs from the surface of lipid droplets. MAGs and FFAs are expected to be predominantly present at the end of the intestinal phase, as an indication that the majority of DAGs and TAGs have been hydrolyzed (Fig. 4).
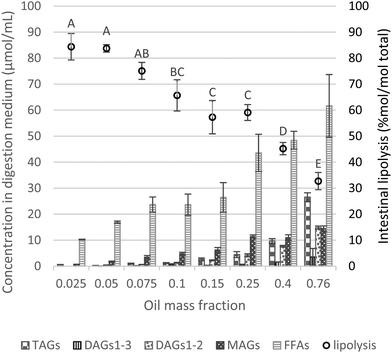 |
| Fig. 4 Proportions of lipid species (concentration of TAGs, DAGs1–3, DAGs1–2, MAGs and FFAs) in the digestive medium (μmol mL−1) and lipolysis degree (% mol per mol total) assessed by HPLC analysis (eqn (4)) as a function of the oil mass fraction in the initial emulsion and at the endpoint of intestinal phase. Different capital letters show a significant difference in intestinal lipolysis (% mol per mol total) among lipid mass fractions at p < 0.05 according to the Tukey test (n = 4). | |
Substantial formation of MAGs occurred during the intestinal phase (i.e., MAGs were produced to a higher extent compared to DAGs), whereas after the gastric phase, DAGs predominated at the expense of MAGs (trace amounts), as depicted in Fig. 3, 4 and S4.† However, upon intestinal digestion of samples with higher lipid mass fractions (φ 0.25–0.76), the formation of MAGs was similar, compensated by a greater presence of DAG1–2 and reduction of DAG1–3. At a very high lipid fraction, the lower formation of FFAs can be related to intermediate products (DAGs and MAGs), in which the digestion could not progress to the final stage (conversion to FFAs).
Similar to the gastric lipolysis behavior, limited intestinal lipolysis at high lipid mass fractions can be related to the absolute amount of lipids (lower dilutions) resulting in enzyme shortage and excess of substrate. Table 2 reports the values of intestinal lipolysis extent estimated by two different approaches. HPLC results are determined based on all classes of lipids present during lipid digestion, i.e. TAGs, DAGs, MAGs and FFAs, whereas the pH-stat approach only accounts for FFAs formation.
Table 2 Lipolysis extent (%) estimated by the ratio of hydrolyzed bonds over the total number of hydrolysable bonds (HPLC) to the final volume of NaOH (VNaOH) consumed to maintain pH 7.0 during intestinal digestion corrected by the blank (minus blank) and by the starting point at pH 7.0 (minus blank and from pH 7.0)
Lipid mass fraction (φ) |
HPLCa (%) |
V
NaOH b (minus blank) (%) |
V
NaOH b (from pH 7.0) (%) |
Values calculated assuming a eqn (4) (n = 4) or b eqn (3) (n = 2). Different capital letters within one column show a significant difference among lipid mass fractions at p < 0.05 according to the Tukey test. Different lowercase letters within alternate pairs show a significant difference between lipolysis as measured by HPLC, NaOH titration (minus blank), and NaOH titration (from pH) at p < 0.05 according to the Tukey test. |
0.025 |
84.4 ± 6.1A,a |
80.4 ± 6.6A,ab |
63.2 ± 4.7A,b |
0.05 |
83.8 ± 1.4A,a |
75.3 ± 0.2A,b |
58.6 ± 0.8AB,c |
0.075 |
75.1 ± 3.3AB,a |
61.6 ± 4.6B,b |
50.0 ± 6.6BC,b |
0.1 |
65.7 ± 6.0BC,a |
50.6 ± 0.2BC,b |
43.5 ± 1.8CD,b |
0.15 |
57.3 ± 6.4C,a |
42.4 ± 2.7CD,b |
38.3 ± 1.5CD,b |
0.25 |
56.0 ± 2.9C,a |
37.5 ± 2.9DE,b |
33.3 ± 1.2DE,b |
0.4 |
45.2 ± 2.4D,a |
28.4 ± 1.7EF,b |
24.1 ± 0.3EF,b |
0.76 |
31.8 ± 4.3E,a |
18.1 ± 1.3F,b |
16.1 ± 1.4F,b |
In general, both methods showed a similar trend in lipolysis as a function of the lipid fraction in the initial emulsion sample (as φ increased, the extent of lipolysis decreased). However, the degree of lipolysis calculated by NaOH titration was always significantly lower (p < 0.05) than the values obtained by HPLC (Table 2), suggesting an underestimation of lipolysis when FFAs are calculated based on titration. Such an underestimation of lipolysis can be related to the physicochemical properties of the medium such as pH, ionic strength, bile salt and calcium concentrations. In fact, all these parameters affect the apparent pKa value of the FFA, which can be important, as the assay pH must be higher or equal to the pKa of FFAs.16 Moreover, the pH-stat approach does not provide any information about the actual digestion products formed (i.e., MAGs and DAGs), which are not accounted in the quantification of lipolysis extent. Furthermore, these considerations are even more relevant when the digested food contains a significant proportion of protein, in which proteolytic products are also titrated by NaOH.40,41 Thus, we believe that the best picture is obtained by combining HPLC and pH-titration interpretations for a comprehensive evaluation of lipolysis.
Interestingly, in the intestinal phase, the final extent of lipolysis decreased with increasing oil concentration with a logarithmic trend (Fig. S5†) that may be related to enzyme saturation.21 The group of Sabet et al. (2022)23 raised this potential pitfall related to the INFOGEST static in vitro digestion protocol applied to oleogels, which are, like HIPEs, matrices containing very high lipid fractions. The authors proposed that the amount of oil in the oleogel to be sampled should be 250 mg, which is much lower when compared to the 5 g of food sample (i.e. how lipid is usually digested in emulsion) recommended by the protocol.23 This is in agreement with our outcomes for φ 0.05, which show satisfactory lipolysis extent (Table 1), endorsing the role of dilution for proper experimental conditions when dealing with high-lipid samples. It can be argued that, in the presence of large amounts of lipids, the shortage of enzymes in the intestinal environment could be mitigated by adding more enzymes. However, from a practical perspective, it is difficult to increase pancreatin concentration due to its limited solubility in simulated intestinal fluid (highly viscous and presence of filaments). It is true that the protocol targets a realistic food consumption scenario, in which diluting or suspending food in an appropriate amount of water should be considered. For instance, aiming to simulate an oil capsule intake with a mouthful of water, a starting sample to in vitro digestion was prepared with an oil-to-water ratio of 1
:
10.42 Nevertheless, the changes caused by dilution or suspension in the enzyme-to-substrate ratio should not be overlooked.
3.2.2.2 Absorbable lipids.
Fig. 5 illustrates the fatty acid composition of the micellar phase, total digesta and accordingly, the calculated absorbable lipids. This shows that up to φ 0.25, comparable values of lipid bioaccessibility were observed, demonstrating no clear effect of the lipid content over this fraction range (φ 0.025–0.25) (Fig. 5c). Beyond this threshold, the bioaccessibility of FAs was governed by the degree of lipolysis, with limited values for the higher lipid mass fractions (φ 0.4–0.76), which indicates that other factors besides lipid content become limiting for the incorporation of lipid digestion products into the mixed micelles.
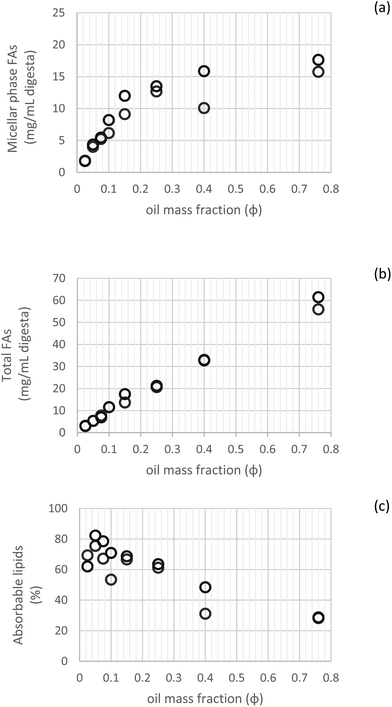 |
| Fig. 5 Fatty acid content in the micellar phase (a), total digesta (b) and their comparison expressed as absorbable lipids (c) (n = 2). | |
A similar trend was observed by other authors when investigating β-carotene bioaccessibility using different oil droplets concentrations. In this case, bioaccessibility was improved by increasing the lipid concentration from φ 0.025 to 0.1, related to the greater solubilization capacity of mixed micelles. However, further increase in lipid content (φ 0.2) caused a reduction in bioaccessibility. These outcomes point out to the fact that the lipid fraction in emulsion influences bioaccessibility by affecting hydrolysis and solubilization in digestion.22
3.2.2.3 Effect of adding RGE addition in the gastric phase on subsequent intestinal lipolysis.
The physiological relevance of gastric lipase is enormous as it notably triggers further action of pancreatic enzymes which determine the digestive fate of lipids.20 It has been reported in a clinical trial that the gastric lipase contributes about 7.5% to small intestinal lipolysis due to the synergistic activity of gastric and pancreatic lipases.7 To determine whether the addition of RGE during the gastric step would impact or not on further pancreatic in vitro lipid digestion, we performed experiments with the addition of pepsin only instead of RGE, keeping the same pepsin activity in both cases (2000 U per mL). When performing the experiment with pepsin, as expected, no gastric lipolysis was observed (100% TAGs), depicting a clear difference in gastric lipolysis caused by the presence of RGE in the gastric phase. In the subsequent intestinal phase for the sample with 0.25 as lipid mass fraction, the final lipolysis extent assessed by HPLC or NaOH titration was comparable (RGE vs. pepsin), as well as the kinetic behavior (Fig. S6†) and the absorbable lipid fraction (Table 3).
Table 3 Lipid hydrolysis extent and bioaccessibility comparing the addition of gastric lipase (RGE) or only pepsin (PEP)
φ
|
Gastric |
Intestinal |
Enzyme |
Lipolysisa (%) |
Lipolysisa (%) |
FFAsb (%) |
Absorbable lipidsc (%) |
n.d. not detectable. Different capital letters within one column show a significant difference between enzyme type (RGE or PEP) in the same oil mass fraction at p < 0.05 according to Tukey test. Different lowercase letters within alternate pairs show a significant difference between lipolysis measured by HPLC (lipolysis) and NaOH titration (FFAs) at p < 0.05 according to Tukey test. HPLC – eqn (4) (n = 4). NaOH volume from pH 7.0 – eqn (3) (n = 2). Determined according to Section 2.2.5 – Determination of absorbable lipids. |
0.25 |
RGE |
7.2 ± 0.5 |
56.0 ± 2.9A,a |
33.3 ± 1.2A,b |
62.4 ± 1.7A |
PEP |
n.d. |
56.4 ± 1.1A,a |
37.0 ± 2.7A,b |
60.2 ± 5.0A |
0.05 |
RGE |
17.7 ± 4.8 |
83.8 ± 1.8A,a |
58.6 ± 0.8A,b |
78.9 ± 4.8A |
PEP |
n.d. |
81.5 ± 1.4A,a |
53.9 ± 0.2B,b |
54.0 ± 4.2B |
As discussed earlier, at a lipid mass fraction of 0.25, a shortage of digestive enzymes may have occurred. Thus, to circumvent the enzyme-to-substrate ratio limitation, a smaller lipid mass fraction (φ 0.05) was also investigated. Here again, no gastric lipolysis was detected for digestion with pepsin and no clear effect of the occurrence or lack of gastric lipolysis on the subsequent intestinal lipolysis extent could be found. Nonetheless, a clear drop in the fraction of absorbable lipids was noticed (Table 3). This could be interpreted as a hint to the role of RGE in lipid digestion. The gastric lipolysis reached with RGE (17.7 ± 4.8%) aligns with the reported levels in literature7–9 and corroborates the relevance of the recommended addition of RGE in the INFOGEST protocol.18 Moreover, the initiated gastric lipolysis in the presence of RGE might be linked to a higher level of absorbable lipids compared to conditions where no gastric lipolysis occurs (i.e., with pepsin), for the 0.05 lipid mass fraction. In a similar fashion, a study by Iddir et al.9 showed that the gastric lipase addition, expected to foster emulsification of lipophilic constituents before their incorporation into mixed micelles, could not only significantly increase lipolysis but also positively affected the carotenoid bioaccessibility in plant food matrices. However, more efforts must be done to unveil the impact of gastric lipase on the overall lipid digestion and metabolic fate in different food matrices.
Overall, for both studied samples (0.05 and 0.25 oil mass fractions), there was a remarkable difference in gastric lipolysis caused by the presence of RGE. However, gastric pre-lipolysis appears to be evened during intestinal digestion for relatively high lipid fractions (in that case, 0.25), which is not true for the 0.05 lipid mass fraction, especially when considering the absorbable lipid content in the digesta (Table 3).
Likewise, a study verifying gastric pre-lipolysis on in vitro lipid digestion in the small intestine using triolein as the lipid phase (φ 0.05) with different interfacial compositions (sodium taurodeoxycholate, citrus pectin, soy protein isolate, soy lecithin, and Tween 80) inferred that gastric pre-lipolysis affected the formation and degradation of intermediate products of lipid digestion in the small intestine phase.5 Interestingly, the different extents of gastric lipolysis observed in these systems did not significantly affect subsequent duodenal lipolysis.5 Moreover, another investigation highlighted that the nature of the emulsifier mainly affected gastric lipolysis, whereas duodenal lipolysis was only marginally affected.29 However, these studies did not make a direct comparison without the presence of gastric lipase in their experiments.
3.2.2.4 Effect of time-dependent behavior and bile salt concentration on digestion assays.
In the previous sections, the effect of initial oil mass fraction, ranging from 0.025 to 0.76 (screening different lipid-to-enzyme ratios), was evaluated on the digestion fate of emulsions and the final extent of lipolysis clearly decreased as the lipid fraction increased. Herein, we took a step forward to understand other parameters that could have limited lipolysis in the in vitro static approach at a lipid mass fraction of 0.25. The following hypotheses were evaluated: (i) the shortage of bile salts in the intestinal medium could cause saturation of the oil–water interface by continuous accumulation of digestion products (i.e., MAGs and FFAs), hindering further digestion by digestive enzymes; (ii) by increasing the time of intestinal digestion, more lipolysis could take place as it does not reach a plateau after 2 h.
The key role of bile salts in continuously removing lipid digestion products from the droplet surface and incorporating them in the form of mixed micelles to transport lipids towards intestinal mucosa is widely recognized.12 Furthermore, from previous experience in our group, it was observed that a greater amount of bile salts was required to incorporate DHA – a long chain polyunsaturated fatty acid (unpublished results) – in mixed micelles. In the INFOGEST protocol, 10 mM bile salts are recommended. Thus, to check whether lipolysis was hindered by a possible shortage of bile salts, we doubled the added bile salt concentration (i.e., 20 mM). Fig. 6 shows that such addition of a larger amount of bile salts affected the rate of lipolysis (kinetic behavior). However, it is likely that bile salts did not limit lipolysis at this lipid mass fraction, allowing lipid digestion to continue at the interface, yielding a rather close lipolysis degree after 2 h compared to the INFOGEST protocol (FFAs = 33.3 ± 1.2 and 37.9 ± 3.0 for INFOGEST and doubled bile salt concentration, respectively measured by NaOH titration) and with no significant different for lipolysis assessed by HPLC (Fig. 6).
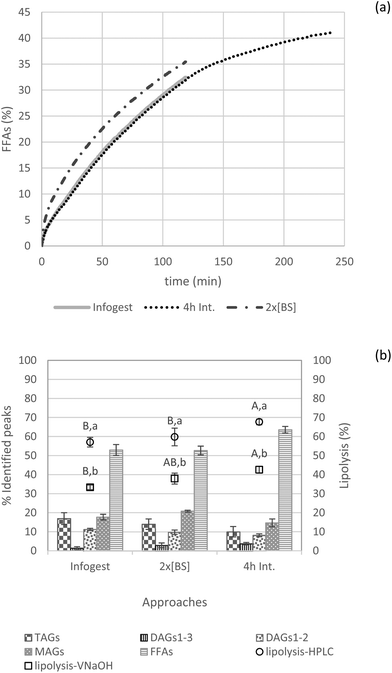 |
| Fig. 6 Representative FFA release curves from NaOH titration (a); lipid species (TAGs, DAGs1–3, DAGs1–2, MAGs and FFAs) present in the digestive media (% of identified peaks) assessed by HPLC analysis and lipolysis degree estimated by HPLC and final NaOH volume (b) for 0.25 oil mass fraction applying the INFOGEST 2.0 protocol, as well as variations of this protocol with doubled bile salts (2× [BS]) and a 4 h intestinal phase. Different capital letters show a significant difference in intestinal lipolysis among approaches (Infogest, 2× [BS] and 4 h Int.) and, different lowercase letters show a significant difference in lipolysis estimated either by HPLC or NaOH titration at p < 0.05 according to the Tukey test at p < 0.05 according to the Tukey test (n = 4). | |
Another study also reported comparable findings, but from a different perspective. Verkempinck and collaborators investigated whether the gradual addition of lipases and bile salts would impact lipid digestion in emulsions. In their systems with a lipid fraction of 0.2, the gradual addition of bile salts significantly reduced the lipolysis rate, but the lipolysis extent after 2 h was not significantly influenced by this alternative digestion procedure.43
Next, to check the time-dependent behavior of in vitro lipolysis, the intestinal phase was set to 4 h instead of the 2 h recommended in the INFOGEST protocol. Indeed, from a physiological point of view, there is evidence that gastrointestinal functions may adapt in response to a high-fat meals or diet.44 Accordingly, slower gastric emptying and longer intestinal transit time are expected as an acute response to a high-fat meal.45
As depicted in Fig. 6, intestinal lipid hydrolysis continues to take place after 2 h increasing the extent of final lipolysis to significant higher degree (for both NaOH and HPLC lipolysis values) when reaching 4 h compared to the 2 h-digestion. Furthermore, this procedure results in the formation of more FFAs than MAGs compared to the other approaches (i.e., INFOGEST and 2× [BS]).
In general, trends caused by increased bile salts in the digestive medium or by prolonged intestinal digestion time (4 h) were confirmed by the lipolysis degree estimated by HPLC and NaOH volume consumption. Herein, it was observed again that the degree of lipolysis calculated by NaOH titration was significantly lower than the values obtained by HPLC.
The fraction of absorbable lipids was not affected for the three evaluated conditions (Fig. S7†).
3.2.2.5 Kinetics – effect of the oil mass fraction.
All these results were gathered in an attempt to understand the possible limitations of in vitro approaches to lipid digestion. To further analyze our experimental outcomes in terms of kinetic rate constants, mathematical empirical models derived from the literature were fitted to experimental data.6,26 In general, both selected models (eqn (1) and (2)) provided good fits of our experimental dataset (Fig. 7). Interestingly, both models predicted the observed data very well at the lowest and highest lipid contents. Conversely, in the intermediate range (φ of 0.15 and 0.25), more deviations were observed. At low lipid fractions (φ 0.025; 0.05 and 0.075), eqn (2) was more suitable, as the rate constant k2 made a significant contribution, with (1 − f) of 0.40, 0.15, and 0.10, respectively (1 − f value of 0.05 for all the other lipid fractions). For these low lipid fractions, k2 rate constant indicates t1/2 values of about 140 s (t1/2 values are about 30 s for all the other lipid fractions). This is likely related to the diffusion of lipase to the oil–water interface, as theorized by Sarkar et al. (2016).46 As the lipid fraction increases, there are fewer lipase molecules per interfacial area and they are physically closer to the interfaces, making their diffusion to the interfaces faster. Authors used experimental data from emulsions formulated with φ 0.05 to establish most of the models. This again points out to the importance of considering the lipid fraction when designing experiments and defining suitable dilutions for HIPEs or concentrated emulsions for in vitro digestion experiments. When considering a longer digestion kinetics for φ 0.25, it was observed that a longer kinetic curve was better fitted, predicting values of maximum FFA released and rate constant k1 closer to the experimental ones (Fig. S8†), probably because the digestion processes were closer to their true endpoint. This result shows that correct parameters derived from long digestion kinetics can also be predicted from shorter digestion kinetics.
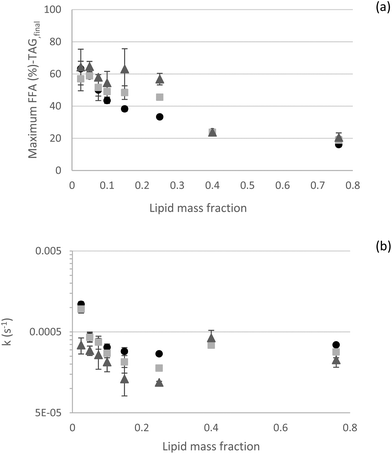 |
| Fig. 7 Experimental (●) and fitting parameters (■ – eqn (1); ▲ – eqn (2)) describing the maximum FFA released (a) and kinetic constant (b). R1 and R2 are the replicates of independent experiments. | |
4. Conclusion
Investigating in vitro lipid digestion is meaningful to evaluate the fate of matrices such as emulsions acting as carriers of nutritionally relevant lipids and lipophilic bioactive compounds. The static pH-stat approach can provide a quick and robust overview of both kinetics and extent of lipolysis. However, several points of attention should be considered when interpreting pH-stat outcomes. Some of them may sound simple enough, yet are not always included and/or reported in literature, which sometimes hampers comparison between studies. For instance, correction with proper blank samples and onset titration according to target intestinal pH must be considered. We also strongly recommend combining it with HPLC analysis (or alternatively, thin layer chromatography) of gastric and intestinal digesta to take into account the concentrations of the lipolysis substrates and products (TAGs, DAGs, MAGs and FFAs). Our results exemplify that the different lipolysis values found with both approaches points towards an underestimation of the lipolysis extent by the titration method, which can be explained by the different underlying principle – the pH-stat method considering only the FFA formation, whereas HPLC quantification addresses the whole picture of the different lipid classes involved. Another important message to convey is the importance of complying with the recommendation pertaining to the addition of RGE in the gastric phase when dealing with in vitro lipid digestion. Increasing the bile salts’ concentration increased the lipolysis rate and, to a smaller degree, the lipolysis extent; and longer digestion times led to increased lipolysis for relatively diluted systems (φ 0.25). Finally, lipid concentration largely influences the outcomes of INFOGEST 2.0 protocol regarding extent (in both gastric and intestinal compartments) and kinetics of lipolysis and, consequently, lipid bioaccessibility. This parameter should therefore be carefully considered – and if necessary, adjusted – in related experimental studies.
Author contributions
Paula K. Okuro: conceptualization, investigation, formal analysis, methodology, visualization, writing – original draft, writing – review & editing; Michèle Viau: investigation, formal analysis, writing – review & editing; Sébastien Marze: investigation, methodology, writing – review & editing; Sophie Laurent: investigation, formal analysis; Rosiane L. Cunha: funding acquisition, writing – review & editing, supervision; Claire Berton-Carabin: funding acquisition, writing – review & editing, supervision, project administration; Anne Meynier: conceptualization, methodology, writing – review & editing, supervision, project administration.
Conflicts of interest
There are no conflicts to declare.
Acknowledgements
The authors would like to thank Perrine Gélébart for her help with confocal microscopy analysis. The authors thank the financial support of FAPESP (grant # 2018/20308-3, 2019/27354-3, and 2019/26046-3, São Paulo Research Foundation – FAPESP), the National Council for Scientific and Technological Development – CNPq (grant 307094/2021-9). C. B.-C. would like to acknowledge Nantes Métropole and Région Pays de la Loire for financial support of this research through her Connect Talent grant (VESTA, grant 2020_12667).
References
- I. Katouzian, A. Faridi Esfanjani, S. M. Jafari and S. Akhavan, Formulation and application of a new generation of lipid nano-carriers for the food bioactive ingredients, Trends Food Sci. Technol., 2017, 68, 14–25 CrossRef CAS.
- M. C. Michalski, C. Genot, C. Gayet, C. Lopez, F. Fine, F. Joffre, J. L. Vendeuvre, J. Bouvier, J. M. Chardigny and K. Raynal-Ljutovac, Multiscale structures of lipids in foods as parameters affecting fatty acid bioavailability and lipid metabolism, Prog. Lipid Res., 2013, 52, 354–373 CrossRef CAS.
- M. N. Corstens, C. C. Berton-Carabin, R. de Vries, F. J. Troost, A. A. M. Masclee and K. Schroën, Food-grade micro-encapsulation systems that may induce satiety via delayed lipolysis: A review, Crit. Rev. Food Sci. Nutr., 2017, 57, 2218–2244 CrossRef CAS.
- C. Mas-Bargues, C. Escrivá, M. Dromant, C. Borrás and J. Viña, Lipid peroxidation as measured by chromatographic determination of malondialdehyde. Human plasma reference values in health and disease, Arch. Biochem. Biophys., 2021, 709, 108941 CrossRef CAS.
- M. R. Infantes-Garcia, S. H. E. Verkempinck, P. G. Gonzalez-Fuentes, M. E. Hendrickx and T. Grauwet, Lipolysis products formation during in vitro gastric digestion is affected by the emulsion interfacial composition, Food Hydrocolloids, 2021, 110, 106163 CrossRef CAS.
- S. H. E. Verkempinck, L. Salvia-Trujillo, M. R. Infantes Garcia, M. E. Hendrickx and T. Grauwet, From single to multiresponse modelling of food digestion kinetics: The case of lipid digestion, J. Food Eng., 2019, 260, 40–49 CrossRef CAS.
- F. Carriere, J. A. Barrowman, R. Verger and L. René, Secretion and contribution to lipolysis of gastric and pancreatic lipases during a test meal in humans, Gastroenterology, 1993, 105, 876–888 CrossRef CAS.
- Y. Gargouri, G. Pieroni, C. Rivière, P. A. Lowe, J.-F. Saunière, L. Sarda and R. Verger, Importance of human gastric lipase for intestinal lipolysis: an in vitro study, Biochim. Biophys. Acta, Lipids Lipid Metab., 1986, 879, 419–423 CrossRef CAS.
- M. Iddir, J. F. Porras Yaruro, Y. Larondelle and T. Bohn, Gastric lipase can significantly increase lipolysis and carotenoid bioaccessibility from plant food matrices in the harmonized INFOGEST static in vitro digestion model, Food Funct., 2021, 12, 9043–9053 RSC.
- D. J. McClements, E. A. Decker and Y. Park, Controlling Lipid Bioavailability through Physicochemical and Structural Approaches, Crit. Rev. Food Sci. Nutr., 2008, 49, 48–67 CrossRef.
- Y. Pafumi, D. Lairon, P. L. de la Porte, C. Juhel, J. Storch, M. Hamosh and M. Armand, Mechanisms of Inhibition of Triacylglycerol Hydrolysis by Human Gastric Lipase, J. Biol. Chem., 2002, 277, 28070–28079 CrossRef CAS.
- A. Macierzanka, A. Torcello-Gómez, C. Jungnickel and J. Maldonado-Valderrama, Bile salts in digestion and transport of lipids, Adv. Colloid Interface Sci., 2019, 274, 102045 CrossRef CAS.
- J. Maldonado-Valderrama, P. Wilde, A. Macierzanka and A. Mackie, The role of bile salts in digestion, Adv. Colloid Interface Sci., 2011, 165, 36–46 CrossRef CAS.
- A.-I. Mulet-Cabero, L. Egger, R. Portmann, O. Ménard, S. Marze, M. Minekus, S. Le Feunteun, A. Sarkar, M. M.-L. Grundy, F. Carrière, M. Golding, D. Dupont, I. Recio, A. Brodkorb and A. Mackie, A standardised semi-dynamic in vitro digestion method suitable for food – an international consensus, Food Funct., 2020, 11, 1702–1720 RSC.
- D. Duijsens, K. Pälchen, J. M. Guevara-Zambrano, S. H. E. Verkempinck, M. R. Infantes-Garcia, M. E. Hendrickx, A. M. Van Loey and T. Grauwet, Strategic choices for in vitro food digestion methodologies enabling food digestion design, Trends Food Sci. Technol., 2022, 126, 61–72 CrossRef CAS.
- A. Helbig, E. Silletti, E. Timmerman, R. J. Hamer and H. Gruppen, In vitro study of intestinal lipolysis using pH-stat and gas chromatography, Food Hydrocolloids, 2012, 28, 10–19 CrossRef CAS.
- M. M. L. Grundy, E. Abrahamse, A. Almgren, M. Alminger, A. Andres, R. M. C. Ariëns, S. Bastiaan-Net, C. Bourlieu-Lacanal, A. Brodkorb, M. R. Bronze, I. Comi, L. Couëdelo, D. Dupont, A. Durand, S. N. El, T. Grauwet, C. Heerup, A. Heredia, M. R. Infantes Garcia, C. Jungnickel, I. E. Kłosowska-Chomiczewska, M. Létisse, A. Macierzanka, A. R. Mackie, D. J. McClements, O. Menard, A. Meynier, M.-C. Michalski, A.-I. Mulet-Cabero, A. Mullertz, F. M. Payeras Perelló, I. Peinado, M. Robert, S. Secouard, A. T. Serra, S. D. Silva, G. Thomassen, C. Tullberg, I. Undeland, C. Vaysse, G. E. Vegarud, S. H. E. Verkempinck, M. Viau, M. Zahir, R. Zhang and F. Carrière, INFOGEST inter-laboratory recommendations for assaying gastric and pancreatic lipases activities prior to in vitro digestion studies, J. Funct. Foods, 2021, 82, 104497 CrossRef CAS.
- A. Brodkorb, L. Egger, M. Alminger, P. Alvito, R. Assunção, S. Ballance, T. Bohn, C. Bourlieu-Lacanal, R. Boutrou, F. Carrière, A. Clemente, M. Corredig, D. Dupont, C. Dufour, C. Edwards, M. Golding, S. Karakaya, B. Kirkhus, S. Le Feunteun, U. Lesmes, A. Macierzanka, A. R. Mackie, C. Martins, S. Marze, D. J. McClements, O. Ménard, M. Minekus, R. Portmann, C. N. Santos, I. Souchon, R. P. Singh, G. E. Vegarud, M. S. J. Wickham, W. Weitschies and I. Recio, INFOGEST static in vitro simulation of gastrointestinal food digestion, Nat. Protoc., 2019, 14, 991–1014 CrossRef CAS.
- M. Minekus, M. Alminger, P. Alvito, S. Ballance, T. Bohn, C. Bourlieu, F. Carrière, R. Boutrou, M. Corredig, D. Dupont, C. Dufour, L. Egger, M. Golding, S. Karakaya, B. Kirkhus, S. Le Feunteun, U. Lesmes, A. MacIerzanka, A. MacKie, S. Marze, D. J. McClements, O. Ménard, I. Recio, C. N. Santos, R. P. Singh, G. E. Vegarud, M. S. J. Wickham, W. Weitschies and A. Brodkorb, A standardised static in vitro digestion method suitable for food-an international consensus, Food Funct., 2014, 5, 1113–1124 RSC.
- L. Sams, J. Paume, J. Giallo and F. Carrière, Relevant pH and lipase for in vitro models of gastric digestion, Food Funct., 2016, 7, 30–45 RSC.
- S. Martínez, M. Espert, A. Salvador and T. Sanz, The role of oil concentration on the rheological properties, microstructure, and in vitro digestion of cellulose ether emulsions, Food Hydrocolloids, 2022, 131, 107793 CrossRef.
- Y. Tan, Z. Zhang, H. Zhou, H. Xiao and D. J. McClements, Factors impacting lipid digestion and β-carotene bioaccessibility assessed by standardized gastrointestinal model (INFOGEST): oil droplet concentration, Food Funct., 2020, 11, 7126–7137 RSC.
- S. Sabet, S. J. Kirjoranta, A.-M. Lampi, M. Lehtonen, E. Pulkkinen and F. Valoppi, Addressing criticalities in the INFOGEST static in vitro digestion protocol for oleogel analysis, Food Res. Int., 2022, 160, 111633 CrossRef CAS.
- E. Pereira, J.-M. Fernandes, R. Gonçalves, A. C. Pinheiro, M. Salomé Duarte, M. Madalena Alves, A. J. A. Meirelles, G. J. Maximo and A. A. Vicente, Evaluating the in vitro digestion of lipids rich in medium-chain fatty acids (MCFAs) using dynamic and static protocols, Food Chem., 2023, 406, 135080 CrossRef CAS.
- Y. Li and D. J. McClements, New Mathematical Model for Interpreting pH-Stat Digestion Profiles: Impact of Lipid Droplet Characteristics on in Vitro Digestibility, J. Agric. Food Chem., 2010, 58, 8085–8092 CrossRef CAS.
- S. Marze and M. Choimet, In vitro digestion of emulsions: mechanistic and experimental models, Soft Matter, 2012, 8, 10982–10993 RSC.
- H. B. Kenmogne-Domguia, A. Meynier, M. Viau, G. Llamas and C. Genot, Gastric conditions control both the evolution of the organization of protein-stabilized emulsions and the kinetic of lipolysis during in vitro digestion, Food Funct., 2012, 3, 1302–1309 RSC.
- P. K. Okuro, M. Viau, A. Kermarrec, R. L. Cunha, A. Meynier and C. Berton-Carabin, Lipid Recovery from Concentrated Emulsions by Freezing–Thawing as an Alternative to Solvent-Based Extraction: A Case Study on Mayonnaise, Eur. J. Lipid Sci. Technol., 2022, 124, 2200101 CrossRef CAS.
- L. Couëdelo, S. Amara, M. Lecomte, E. Meugnier, J. Monteil, L. Fonseca, G. Pineau, M. Cansell, F. Carrière, M. C. Michalski and C. Vaysse, Impact of various emulsifiers on ALA bioavailability and chylomicron synthesis through changes in gastrointestinal lipolysis, Food Funct., 2015, 6, 1726–1735 RSC.
- W. R. Morrison and L. M. Smith, Preparation of fatty acid methyl esters and dimethylacetals from lipids with boron fluoride–methanol, J. Lipid Res., 1964, 5, 600–608 CrossRef CAS.
- A. Meynier, C. Leborgne, M. Viau, P. Schuck, M. Guichardant, C. Rannou and M. Anton, n-3 fatty acid enriched eggs and production of egg yolk powders: An increased risk of lipid oxidation?, Food Chem., 2014, 153, 94–100 CrossRef CAS.
- M. N. Corstens, C. C. Berton-Carabin, K. Schroën, M. Viau and A. Meynier, Emulsion encapsulation in calcium-alginate beads delays lipolysis during dynamic in vitro digestion, J. Funct. Foods, 2018, 46, 394–402 CrossRef CAS.
- V. M. Pizones Ruiz-Henestrosa, L. Ribourg, A. Kermarrec, M. Anton, A. Pilosof, M. Viau and A. Meynier, Emulsifiers modulate the extent of gastric lipolysis during the dynamic in vitro digestion of submicron chia oil/water emulsions with limited impact on the final extent of intestinal lipolysis, Food Hydrocolloids, 2022, 124, 107336 CrossRef CAS.
- J. Calvo-Lerma, V. Fornés-Ferrer, A. Heredia and A. Andrés, In vitro digestion models to assess lipolysis: The impact of the simulated conditions of gastric and intestinal pH, bile salts and digestive fluids, Food Res. Int., 2019, 125, 108511 CrossRef CAS.
- M. Bautista Villarreal, C. T. Gallardo Rivera, E. García Márquez, J. Rodríguez Rodríguez, M. A. Núñez González, A. Chávez Montes and J. G. Báez González, Comparative Reduction of Egg Yolk Cholesterol Using Anionic Chelating Agents, Molecules, 2018, 23, 3204 CrossRef.
- H. Singh and A. Ye, Structural and biochemical factors affecting the digestion of protein-stabilized emulsions, Curr. Opin. Colloid Interface Sci., 2013, 18, 360–370 CrossRef CAS.
- L. Cheng, A. Ye, Y. Hemar and H. Singh, Modification of the interfacial structure of droplet-stabilised emulsions during in vitro dynamic gastric digestion: Impact on in vitro intestinal lipid digestion, J. Colloid Interface Sci., 2022, 608, 1286–1296 CrossRef CAS.
- M. Armand, Lipases and lipolysis in the human digestive tract: where do we stand?, Curr. Opin. Clin. Nutr. Metab. Care, 2007, 10, 156–164 CrossRef CAS.
- M. R. Infantes-Garcia, S. H. E. Verkempinck, M. E. Hendrickx and T. Grauwet, Kinetic Modeling of In Vitro Small Intestinal Lipid Digestion as Affected by the Emulsion Interfacial Composition and Gastric Prelipolysis, J. Agric. Food Chem., 2021, 69, 4708–4719 CrossRef CAS.
- D. J. L. Mat, I. Souchon, C. Michon and S. Le Feunteun, Gastro-intestinal in vitro digestions of protein emulsions monitored by pH-stat: Influence of structural properties and interplay between proteolysis and lipolysis, Food Chem., 2020, 311, 125946 CrossRef CAS.
- D. J. L. Mat, S. Le Feunteun, C. Michon and I. Souchon, In vitro digestion of foods using pH-stat and the INFOGEST protocol: Impact of matrix structure on digestion kinetics of macronutrients, proteins and lipids, Food Res. Int., 2016, 88, 226–233 CrossRef CAS.
- C. Tullberg, G. Vegarud and I. Undeland, Oxidation of marine oils during in vitro gastrointestinal digestion with human digestive fluids – Role of oil origin, added tocopherols and lipolytic activity, Food Chem., 2019, 270, 527–537 CrossRef CAS.
- S. H. E. Verkempinck, J. M. Guevara-Zambrano, M. R. Infantes-Garcia, M. C. Naranjo, R. Soliva-Fortuny, P. Elez-Martínez and T. Grauwet, Gastric and small intestinal lipid digestion kinetics as affected by the gradual addition of lipases and bile salts, Food Biosci., 2022, 46, 101595 CrossRef CAS.
- F. A. Duca, Y. Sakar and M. Covasa, The modulatory role of high fat feeding on gastrointestinal signals in obesity, J. Nutr. Biochem., 2013, 24, 1663–1677 CrossRef CAS.
- C. Dirksen, J. Graff, S. Fuglsang, J. F. Rehfeld, J. J. Holst and J. L. Madsen, Energy intake, gastrointestinal transit, and gut hormone release in response to oral triglycerides and fatty acids in men with and without severe obesity, Am. J. Physiol.: Gastrointest. Liver Physiol., 2018, 316, G332–G337 CrossRef.
- A. Sarkar, B. Murray, M. Holmes, R. Ettelaie, A. Abdalla and X. Yang, In vitro digestion of Pickering emulsions stabilized by soft whey protein microgel particles: influence of thermal treatment, Soft Matter, 2016, 12, 3558–3569 RSC.
|
This journal is © The Royal Society of Chemistry 2023 |