DOI:
10.1039/D3FO01651J
(Paper)
Food Funct., 2023,
14, 10855-10867
Pediococcus acidilactici (pA1c®) alleviates obesity-related dyslipidemia and inflammation in Wistar rats by activating beta-oxidation and modulating the gut microbiota†
Received
24th April 2023
, Accepted 6th November 2023
First published on 9th November 2023
Abstract
Due to the importance of the gut microbiota in the regulation of energy homeostasis, probiotics have emerged as an alternative therapy to ameliorate obesity-related disturbances, including cholesterol metabolism dysregulation, dyslipidemia and inflammation. Therefore, the objectives of this study were to evaluate the effect of the probiotic strain Pediococcus acidilactici (pA1c®) on the regulation of adiposity, cholesterol and lipid metabolism, inflammatory markers and gut microbiota composition in diet-induced obese rats. Twenty-nine four-week-old male Wistar rats were divided into three groups: rats fed a control diet (CNT group, n = 8), rats fed a high fat/high sucrose diet (HFS group, n = 11), and rats fed a HFS diet supplemented with pA1c® (pA1c®group, n = 10). Organs and fat depots were weighed, and different biochemical parameters were analysed in serum. Gene expression analyses in the adipose tissue were conducted using real-time quantitative-PCR. Faecal microbiota composition was evaluated using 16S metagenomics. Animals supplemented with pA1c® exhibited a lower proportion of visceral adiposity, a higher proportion of muscle, an improvement in the total-cholesterol/HDL-cholesterol ratio and a decrease in the total cholesterol, triglyceride and aspartate aminotransaminase (AST) serum levels, together with a decrease in several inflammation-related molecules. The expression of key genes related to adipose (Adipoq, Cebpa and Pparg) and glucose (Slc2a1 and Slc2a4) metabolism in the adipose tissue was normalized by pA1c®. Moreover, it was demonstrated that pA1c® supplementation activated fatty acid β-oxidation in the adipose tissue and the liver. Metagenomics demonstrated the presence of pA1c® in the faecal samples, an increase in alpha diversity, an increase in the abundance of beneficial bacteria, and a decrease in the abundance of harmful micro-organisms, including the Streptococcus genus. Thus, our data suggest the potential of pA1c® in the prevention of obesity-related disturbances including hypercholesterolemia, hypertriglyceridemia, inflammation and gut microbiota dysbiosis.
1. Introduction
Obesity corresponds to a worldwide chronic disease of multifaceted aetiology, characterized by excessive accumulation and unusual distribution of the adipose tissue that lead to physiopathological dysfunction of the human organism.1,2 Being a complex disease, besides the affectation of the adipose tissue, other factors can accompany this pathology, such as dyslipidemia,3,4 cholesterol dysregulation,5 and inflammation (cytokines),6–9 among others. Furthermore, the prevalence of obesity has been rising gradually over the last few years and is currently at unprecedented levels: more than 68% of adults in the United States are considered overweight, and 35% are obese.10,11 This problem also has an impact on the children and adolescent population, with rising obesity rates in almost all nations in the last four decades.12 For all these reasons, it has become vital, crucial and compulsory to develop novel and alternative therapies and treatments to reduce the progression of this pathology and deal with obesity-related disturbances, including dyslipidemia and inflammation.
In recent times, numerous works have shown a strong link between obesity and dysbiosis.6,13–16 Chronic low-grade inflammation and gut microbiota dysbiosis are two of the most common obesity-related disorders, being defined as long-term inflammation lasting several months to years17 and an imbalance in the gut microbial community that is associated with disease,18 respectively. The gut microbiota has been considered a superorganism within our bodies that can modulate many physiological functions, including immunity development19,20 and inflammation regulation.21,22 For this reason, it is important to identify strategies that are able to modulate intestinal microbiota composition, to turn back the dysbiosis and to help alleviate the problems caused by obesity. Thereby, supplementation with beneficial microorganisms (probiotics) and bioactive compounds that have anti-obesogenic properties may be a good alternative to existing conventional and more invasive treatments for obesity.
Previous studies of our group demonstrated that pA1c® was able to regulate the lipid and carbohydrate metabolism in C. elegans through the modulation of the insulin signalling pathway.23 Moreover, the probiotic strain pA1c® was able to regulate glucose metabolism in diabetic mice.24 Bearing this in mind, and with the goal of continuing to explore the metabolic activities of pA1c®, we administered this probiotic strain to male Wistar rats fed an obesogenic diet, which mimics three important obesity-related disturbances, namely, fat accumulation, dyslipidemia and inflammation. We propose that pA1c®, apart from glucose metabolism regulation in diabetic mice,24 may also be involved in the regulation of lipid metabolism and inflammation. Hence, the objectives of this study were: (1) the evaluation of the effects of the supplementation with pA1c® on the regulation of adiposity and dyslipidemia in diet-induced obese rats and (2) the investigation of the mechanism of action of pA1c® and its impact on the gut microbiota composition in diet-induced obese rats.
2. Materials and methods
2.1. Bacterial strain
Pediococcus acidilactici strain pA1c® was deposited, according to the Budapest Treaty, in the Spanish Collection of Type Cultures (CECT) with identification reference CECT 9879 from the proprietary strain collection of Genbioma Aplicaciones S.L. (Polígono industrial Noain-Esquiroz, S Street, Nave 4, Navarra, 31191, Spain) backed by an international patent “Probiotics for regulating blood glucose [PCT/EP2020/087284; WO2021/123355A1]”. This bacterium was grown in deMan-Rogosa-Sharpe Agar (MRS) medium at 37 °C (facultative anaerobe). This species meets the criteria of qualified presumption of safety (QPS) by the European Food Safety Authority (EFSA) and GRAS status (FDA).
2.2. Experimental design
Twenty-nine four-week-old male Wistar rats (Charles River) were used in this study. The rats were kept in an isolated room with controlled temperature (21–23 °C) and humidity (50% ± 10%), and a 12 h
:
12 h artificial light/dark cycle. All rats were acclimatized to the experimental facility for two weeks, where all animals were fed a control diet. Following the acclimation period, the animals were randomly divided and allocated into three groups until the end of the study. One group (control diet group, CNT; n = 8) was still given the control diet (2014, Teklad Global 14% Protein Rodent Maintenance Diet), and the other two groups received a high fat/high sucrose (HFS) diet (D12451, Research Diets, 20 Jules Lane; New Brunswick, NJ) for nine weeks. One of the HFS groups, named HFS (n = 11), was fed only the HFS diet, while the other group named pA1c® (n = 10) was supplemented with the probiotic strain pA1c® in addition to the HFS diet. The rats were housed in individual cages with ad libitum access to water and controlled food intake. Body weight was checked weekly and food intake was recorded two times per week.
All animals were euthanized by decapitation at week nine of supplementation, trunk blood was collected, and serum and plasma samples were obtained for biochemical analysis. Tissue samples from the liver, kidneys, spleen, gastrocnemius muscle, and white adipose tissue (WAT) depots (mesenteric, retroperitoneal, epididymal, and subcutaneous) were isolated, weighed and immediately stored at −80 °C. All procedures were performed following the national and institutional ethical guidelines of the Care and Use of Laboratory Animals, with the consent of the Food Safety and Environmental Health Service of the Government of Navarra, Spain. The protocol was approved by the Ethics Committee for Animal Experimentation of the University of Navarra (protocol reference 038-21).
2.3. Experimental diets
The control diet contained 14% of protein. The commercial HFS diet had the following energy distribution: 20% of kcal corresponding to protein, 35% of kcal corresponding to carbohydrates and 45% of kcal corresponding to fat (ESI Table 1†). In the pA1c® group, the lyophilized probiotic strain pA1c® (1010 CFU per day per rat) was mixed with the HFS diet and given to the animals during the whole experiment. The diet mixed with the probiotic was changed every 3 days to avoid rancidity and oxidation. The lactic acid bacteria (LAB) counts in the HFS diet + pA1c® were used as viability confirmation of the strain and were performed by plate counting on MRS agar (Scharlau, Barcelona, Spain), incubated for 48 h at 37 °C under a CO2 atmosphere (5%).
2.4. Biochemical analyses
Total cholesterol, HDL-cholesterol (HDL-Chol), triglycerides (TG), glucose, aspartate aminotransaminase (AST), and alanine aminotransaminase (ALT) were quantified with an HK-CP kit (ABX Pentra, Montpellier, France) adapted for a Pentra C200 analyser (HORIBA ABX, Montpellier, France). Monocyte chemotactic protein-1 (MCP-1), oxidized low-density lipoprotein (ox-LDL) and interleukin-6 (IL-6) serum concentrations were quantified using specific ELISA kits (Thermo Fisher Scientific Inc., Waltham, MA, and MyBiosource, San Diego, CA). Serum insulin was quantified with a specific ELISA kit (Mercodia AB, Uppsala, Sweden). The log (TG/HDL-Chol) ratio was calculated to determine the atherogenic index of plasma (AIP).25
2.5. RNA extraction and quantitative PCR analysis
Total RNA from 200 mg of mesenteric fat and liver samples from the control, HFS and pA1c® samples was extracted using TRIzol® RNA isolation reagent (Invitrogen Life Technologies, Paisley, UK). The concentration and purity of RNA were determined at 260/280 nm using a NanoDrop ND-1000 spectrophotometer (Thermo Fisher Scientific, Wilmington, DE). Then, 1 μg of RNA was treated with DNAse I (DNase I-RNase free, Invitrogen Life Technologies) and reverse-transcribed into cDNA using 200 IU of M-MLV-RT (Invitrogen Life Technologies) in the presence of 40 IU of recombinant RNAsin® Ribonuclease inhibitor (Promega, Madison, WI), with incubation for 10 min at 25 °C, 50 min at 37 °C and 15 min at 70 °C. Gene expression analyses were performed by quantitative-real time PCR (qPCR) using TaqMan Universal PCR master mix and specific probes from Life Technologies: Adipoq, Cebpa, Fasn, Slc2a1, Slc2a4, Pparg, Srebf, Acot-8, Acox-1, Cpt-1a, Cpt-2, Hsd-17b4, Plin-1, Scp-2, Scd-1, Aaca-2, Hmgcl and Hmgcs-2 (TaqManTM Gene Expression Assays) in triplicate using a CFX384 TouchTM Real-Time PCR Detection System (BioRad Laboratories, Hercules, CA). Gene expression levels were normalized compared to TATA box binding protein (Tbp) gene expression as a housekeeping control. Gene expression differences between the pA1c®-supplemented and non-supplemented samples were estimated using the relative quantification 2−ΔΔCt method.
2.6. Fecal sample collection and metagenomic analyses
Feces of the different groups of the study were collected on week nine of supplementation and immediately stored at −80 °C. DNA isolation and bacterial DNA sequencing analyses were performed at the CIMA LAB Diagnostics, Genomics Unit of the Centre for Applied Medical Research (Pamplona, Spain). dsDNA characterization was performed using Qubit (Thermo Fisher). The regions V3 and V4 of the ribosomal 16S gene were sequenced by using Illumina protocols explained elsewhere.26 Briefly, sequencing consists of two polymerase chain reactions (PCRs), in which the V3 and V4 regions of the 16S rRNA gene were amplified. It required the use of 16S-forward and 16S-reverse specific primers (16S Amplicon PCR forward primer = 5 0 TCGTCGGCAGCGTCAGATGTGTA TAAGAGACAGCCTACGGGNGGCWGCAG; 16S Amplicon PCR Reverse Primer = 5 0 GTCTCGTGGGCTCGGAGATGTGTATAAGAGACAGGACTACHVGGGTATCTAATCC; Nextera XT DNA Index Kit FC-131-1002 Illumina; San Diego, CA). The protocol followed for the first PCR was at 95 °C for 3 min and 25 cycles of 95 °C for 30 s, 55 °C for 30 s, 72 °C for 30 s, and 72 °C for 5 min. After the cleansing process, 5 μl was taken from the first PCR sample to be used for the second PCR. For the second PCR, the protocol followed was 95 °C for 3 min and 8 cycles of 95 °C for 30 s, 55 °C for 30 s, 72 °C for 30 s, and 72 °C for 5 min. A cleansing process was performed after each PCR to remove the primers from the sample. Afterwards, the samples were loaded into an MiSeq equipment for sequencing and quantification. The operational taxonomic unit (OTU) grouping methods were used to analyze the gut microbiome. Taxonomy was assigned using BLAST and HITdb. The alignments of the different OTUs (sequences) were conducted using the workflow of the 16S Metagenomics of Illumina database, which allows the classification at the phylum, class, order, family, genus or species level. The abundance matrices were filtered and then normalized at each level of classification. Bioinformatic analyses of the differences in the gut microbiota of the different groups were performed using MicrobiomeAnalyst. Finally, a predictive analysis of the metabolic function of the intestinal microbiota was conducted by Tax4Fun using the program MicrobiomeAnalyst.
2.7. Statistical analyses
Body related tissues and biochemical parameters, qPCR analyses and ELISAs were evaluated using the ordinary one-way ANOVA test followed by Fisher's LSD test when significant differences were obtained. 16S metagenomics (alpha-diversity and the differential abundance of families, genera and species) were analysed using Metagenome-seq of MicrobiomeAnalyst. Correlation analyses were performed using Spearman's rank correlation coefficient.
3. Results and discussion
3.1. pA1c® reduces adipose tissue accumulation and increases lean muscle in rats
No differences in the total body weight were observed between the pA1c® group and the HFS group, although there was a non-significant downward trend in the probiotic-supplemented rats (Table 1). No differences were also found in the weights of the liver, spleen and kidneys between the CNT, HFS and pA1c® groups. However, the animals supplemented with pA1c® exhibited a higher proportion of lean muscle tissue than the HFS rats. Compared with the HFS group, the rats that received the probiotic had lower weights of all the fat depots, and this weight reduction was statistically significant in the mesenteric fat (Table 1). These results confirm the findings of our previous work in which we demonstrated the fat accumulation- and lipid droplet-reducing properties of the probiotic pA1c® in C. elegans.23 The tendency of the probiotic to reduce all types of visceral fat led us to think that a fattening phase (weight gain) before the experiment or a longer duration of the supplementation phase could have revealed more pronounced significant differences in the adipose tissue between the pA1c® and HFS groups. Numerous studies have linked high mesenteric fat with obesity and inflammation.27–29 Therefore, a possible mechanism of action of the probiotic could be the reduction of mesenteric fat and thus inflammation.
Table 1 Tissue weights in Wistar rats under normal and experimental conditionsa
|
CNT (n = 8) |
HFS (n = 11) |
pA1c® (n = 10) |
ANOVA (p) |
Data are expressed as mean ± SEM. Statistical analyses were performed using the one-way ANOVA test followed by the Fisher's LSD test when statistical significance (p < 0.05) was reached in the ANOVA test. (Fisher's LSD test, *p < 0.05 and **p < 0.01 with respect to the HFS group; and ##p < 0.01 and ###p < 0.001 with respect to the CNT group.) CNT, control; HFS, high-fat-sucrose; pA1c®, Pediococcus acidilactici; and WAT, white adipose tissue. Visceral fat WAT corresponds to the sum of mesenteric, epididymal and retroperitoneal fat depots; and total WAT corresponds to the sum of visceral WAT and subcutaneous fat.
|
Weight (g)
|
409.83 ± 7.95 |
474.09 ± 7.40### |
460.16 ± 8.92### |
<0.001 |
Tissue weights (weight proportion related to total body weight)
|
Liver |
2.37 ± 0.06 |
2.48 ± 0.09 |
2.42 ± 0.06 |
ns |
Spleen |
0.18 ± 0.01 |
0.17 ± 0.01 |
0.18 ± 0.01 |
ns |
Kidney |
0.27 ± 0.01 |
0.27 ± 0.01 |
0.28 ± 0.01 |
ns |
Gastrocnemius muscle |
0.55 ± 0.01 |
0.47 ± 0.01### |
0.51 ± 0.01*,## |
<0.001 |
WAT depot weight (weight proportion related to total body weight)
|
Mesenteric fat |
0.59 ± 0.10 |
1.27 ± 0.07### |
0.97 ± 0.05**,## |
<0.001 |
Epididymal fat |
1.78 ± 0.15 |
3.36 ± 0.26### |
3.18 ± 0.20### |
<0.001 |
Subcutaneous fat |
1.38 ± 0.08 |
2.33 ± 0.13### |
2.18 ± 0.16### |
<0.001 |
Retroperitoneal fat |
1.44 ± 0.15 |
3.02 ± 0.24### |
2.95 ± 0.21### |
<0.001 |
Visceral WAT |
3.81 ± 0.37 |
7.66 ± 0.42### |
7.17 ± 0.45### |
<0.001 |
Total WAT |
5.19 ± 0.44 |
9.98 ± 0.48### |
9.35 ± 0.58### |
<0.001 |
3.2. pA1c® reduces cholesterol and triglyceride contents, and enhances inflammatory response in rats
pA1c® supplementation significantly reduced total cholesterol and triglyceride levels when compared with the HFS group. In fact, although without statistical significance, the pA1c® rats showed even lower values than the control group, normalizing the HFS-induced dyslipidemia (Table 2): the pA1c®-supplemented animals showed a lower ratio between total cholesterol and HDL-cholesterol than the HFS rats, indicating an amelioration of the cholesterol profile. In addition, recent studies have shown that the species Pediococcus acidilactici could be used as a cholesterol-reducing probiotic for preventing or managing hypercholesterolemia and dyslipidemia.30–32 Taking all these data together, we suggest that supplementation with pA1c® could be a potential alternative or complementary strategy to traditional hypercholesterolemia treatments. However, it is necessary to go deeper into the study of the mechanism of action of the probiotic in cholesterol metabolism.
Table 2 Biochemical variables in C57BL/6J mice under normal and experimental conditionsa
|
CNT (n = 8) |
HFS (n = 11) |
pA1c® (n = 10) |
ANOVA (p) |
Data are expressed as mean ± SEM. Statistical analyses were performed using the one-way ANOVA test followed by the Fisher's LSD test when statistical significance (p < 0.05) was reached in the ANOVA test. (Fisher's LSD test, *p < 0.05 and **p < 0.01 with respect to the HFS group; and ##p < 0.01 and ###p < 0.001 with respect to the CNT group.) CNT, control; HFS, high-fat-sucrose; pA1c®, Pediococcus acidilactici; TG, triglycerides; AIP, atherogenic index of plasma; ALT, alanine aminotransferase; and AST, aspartate aminotransferase.
|
Cholesterol metabolism biomarkers
|
Cholesterol (mg dL−1) |
89.66 ± 4.89 |
105.7 ± 5.58 |
82.88 ± 5.20** |
<0.05 |
HDL-Chol (mg dL−1) |
24.11 ± 0.80 |
23.63 ± 0.90 |
21.29 ± 1.06 |
ns |
Total Chol/HDL |
3.71 ± 0.13 |
4.46 ± 0.12### |
3.88 ± 0.10** |
<0.001 |
TG (mg dL−1) |
56.50 ± 2.86 |
68.00 ± 6.51 |
48.86 ± 3.76* |
<0.05 |
AIP |
0.37 ± 0.02 |
0.45 ± 0.05 |
0.36 ± 0.01 |
ns |
Hepatic biomarkers
|
ALT (U L−1) |
43.33 ± 1.22 |
47.43 ± 1.25 |
47.44 ± 1.16 |
ns |
AST (U L−1) |
172.16 ± 5.10 |
204.22 ± 5.35## |
181.35 ± 6.67* |
<0.05 |
Moreover, although no statistically significant difference was found in the atherogenic index of plasma (AIP), the pA1c® group showed lower levels than the control and HFS rats. Furthermore, a significant decrease in one of the most important hepatic biomarkers of liver damage (AST) was found in rats supplemented with the probiotic in comparison with the HFS group.
Regarding inflammation, a decrease in the circulating levels of three pro-inflammatory biomarkers (MCP-1, ox-LDL and IL-6) was observed in the pA1c®-supplemented group when compared with the HFS group (Fig. 1A–C). MCP-1 is one of the key chemokines that regulate migration and infiltration of monocytes/macrophages.33 Likewise, ox-LDL has been found to have antigenic potential and contribute heavily to atherosclerosis-associated inflammation, activating both innate and adaptive immunity.34 In the same way, IL-6 is a pro-inflammatory cytokine closely related to obesity, inflammation and immunity.35 Besides, it is more than proven that cytokines that are released by inflammatory cells infiltrating the obese adipose tissue, such as IL-6 and MCP-1, can act on immune cells leading to local and generalized inflammation.36 Furthermore, the white adipose tissue of obese people is characterized by increased production and secretion of a wide range of inflammatory molecules including IL-6, which may have local effects on the same white adipose tissue physiology but also systemic effects on other organs such as the liver.37 Hence, and in conjunction with the above results, we can corroborate the strong anti-inflammatory effect of pA1c®, and at the same time, we can complement the mechanism of action proposed with the reported findings. Therefore, the molecular mechanism of action by which this probiotic exerts its anti-obesogenic effect is by reducing visceral adiposity (mesenteric fat), TG, cholesterol, hepatic biomarker (AST), and the serum levels of three important pro-inflammatory molecules (MCP-1, ox-LDL and IL-6) that are usually increased in obese people.
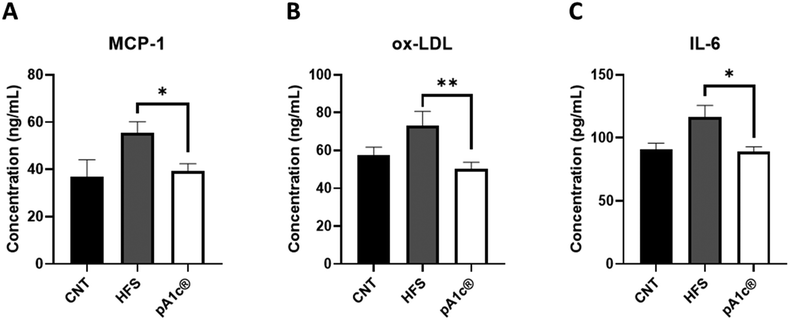 |
| Fig. 1 The MCP-1 (A), ox-LDL (B) and IL-6 (C) levels of the control, HFS and probiotic-treated rats quantified by ELISA. Statistical analyses were performed using the one-way ANOVA test followed by the Fisher's LSD test when statistical significance (p < 0.05) was reached in the ANOVA test (Fisher's LSD test, *p < 0.05 with respect to the HFS group). | |
3.3. The anti-obesogenic activities of pA1c® are mediated by anti-inflammatory pathways, adipocyte differentiation, and the activation of the insulin signalling pathway, the fatty acid beta-oxidation pathway and the lipolysis process
Gene expression analyses of the mesenteric fat were performed due to the probiotic-induced reduction of this fat depot, and of liver tissue (Fig. 2). Adiponectin (Adipoq) is the most abundant peptide secreted by adipocytes. Its regulation plays a key role in obesity-related diseases, regulating inflammation and energy metabolism, stimulating fatty acid oxidation, reducing plasma triglycerides and improving glucose metabolism by increasing insulin sensitivity.38,39 Thus, its significant upregulation in the pA1c®-supplemented group compared with the HFS and CNT groups (Fig. 2A) may explain the biomolecular pathway by which the probiotic increases the anti-inflammatory response and reduces the TG serum levels. Moreover, a downregulation of Cebpa was found in the pA1c®-supplemented group in comparison with the HFS rats in both the mesenteric fat and liver (Fig. 2A and C). The members of the C/EBP family of transcription factors participate as critical regulators throughout the development of adipocyte differentiation where C/EBPα proteins were found to play a central role in later stages of adipocyte maturation.40–42 This reduction in Cebpa is in consonance with similar obesogenic studies that have been carried out, where the authors conclude that the decreased weight gain and adipose tissue mass and the reduction of TG after a treatment were caused by the inhibition of Cebpa.42,43 Furthermore, it has also been demonstrated that the reducing levels of Cebpa in adipose tissue could reduce insulin resistance and pro-inflammatory cytokines such as IL-6.42,44 Thus, it is suggested that Cebpa may be an important mediator in the fat-reducing and inflammatory-normalizing activities of the probiotic, although further studies are needed. On the other hand, an upregulation of two important genes that govern glucose metabolism (Slc2a1 and Slc2a4) was observed in the pA1c®-supplemented group when compared with the HFS group. In the case of Slc2a1, a significant increase was also observed when compared with the CNT group. GLUT proteins are encoded by the Slc2 genes and are members of the major facilitator superfamily of membrane transporters. GLUTs 1–5 are the most thoroughly studied and all have well-established roles as glucose and/or fructose transporters in various tissues and cell types.45 Inhibition of these genes appears to be closely related to insulin resistance, so their overexpression, together with that of adiponectin, may help to combat obesity and the related disturbances arising from it. Furthermore, a strong upregulation of the peroxisome proliferator-activated receptor gamma (Pparg) in the pA1c® group was noticed. Several studies linked the overexpression of Pparg with an insulin resistance- and dyslipidemia-alleviating effect and an anti-inflammatory activity,46–48 so this finding supports the anti-inflammatory and dyslipidemia-alleviating role of pA1c® in obesity. No differences were found in Fasn and Srebf expression in mesenteric fat. Interestingly, these 2 genes, together with Scd-1, were found significantly downregulated in the liver with respect to the HFS group, and even with the control in the case of Fasn and Scd-1 (Fig. 2C). Srebf, Scd-1 and Fasn are well-known lipogenic genes, and are involved in the synthesis of fatty acids and adipogenesis.49–51 In addition, they are highly interconnected with each other, since upregulated Srebf levels are capable of activating Fasn.52 Beysen et al. demonstrated the importance of Fasn in the process of lipogenesis, which inhibited alleviated hepatic steatosis, non-alcoholic fatty liver disease (NAFLD) and obesity.53 Moreover, Qin et al. reported that dietary fibre alleviates hepatic fat deposition via inhibition of lipogenic gene expression of Srebf.54 Hence, these findings support the anti-lipogenic role of pA1c® in the liver of individuals with obesity and are in agreement with previous studies conducted by our group on C. elegans and mice.23,55
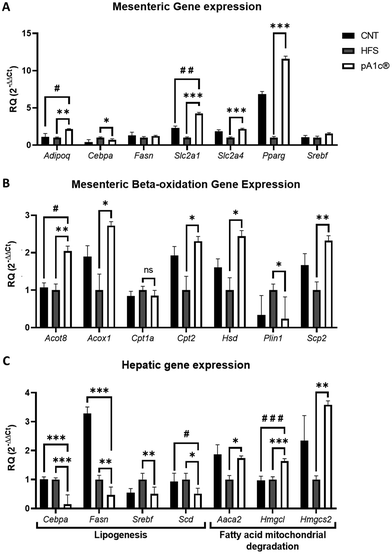 |
| Fig. 2 Mesenteric fat gene (A), fatty acid beta-oxidation and lipolysis (B) and liver (C) gene expression quantified by real-time PCR (qPCR) in Wistar rats. The gene expression levels were normalized to the housekeeping gene (Tbp-1). Data are expressed using the 2−ΔΔCt method. Statistical analyses were performed using the one-way ANOVA test followed by the Fisher's LSD test when statistical significance (p < 0.05) was reached in the ANOVA test (Fisher's LSD test, nsp > 0.05, *p < 0.05, **p < 0.01, and ***p < 0.001 with respect to the HFS group; #p < 0.05 and ###p < 0.001 with respect to the CNT group). | |
The expression analyses of beta-oxidation genes in mesenteric fat showed an upregulation of the fatty acid degradation process in the pA1c®-supplemented group, through the activation of the peroxisomal (by the upregulation of the Acot-8, Acox-1, Hsd-17b4 and Scp-2 genes in mesenteric fat) and mitochondrial (by the upregulation of Cpt-2) fatty acid beta-oxidation, in comparison with the HFS group (Fig. 2B). This increase in fatty acid oxidation would be confirmed again in liver tissue, where we observed a significant upregulation of the Aaca-2, Hmgcl and Hmgcs-2 genes (Fig. 2C). The triggering of fatty acid beta-oxidation in adipose and liver tissues after the probiotic supplementation could be one of the mechanisms by which mesenteric and visceral fat reduction occurred. Importantly, this rise in beta-oxidation, together with the overexpression of the glucose metabolism genes Slc2a1 and Slc2a4, is in concordance with our previous studies on C. elegans and mice, where we demonstrated that the mechanism of action of pA1c® affected the insulin signalling and beta-oxidation pathways.23,55 Looking more closely at Fig. 2B reveals a downregulation of perilipin-1 (Plin-1), which is a gene that encodes lipid droplet surface proteins named perilipins, in the pA1c® rats compared with the HFS rats. Perilipins sequester lipids by protecting lipid droplets from a lipase action modulating and governing the lipolysis process.56 Associated studies have established a strong relationship between high levels of Plin-1 in the white adipose tissue and excessive fat accumulation and adiposity, and the development of chronic inflammation and obesity.57,58 Therefore, the reduction of Plin-1 by the probiotic activated the lipolysis process, which, combined with the increase in beta-oxidation, could be a promising alternative approach for therapy against obesity. Probably, if we had extended the study a few more weeks, we would have been able to see these changes in gene expression reflected at the phenotypic level (significant reduction in the total weight of the pA1c®-supplemented rats compared with the HFS rats not supplemented with pA1c®).
3.4. pA1c® modulates increasing alpha diversity and the abundance of beneficial bacteria and decreasing harmful micro-organisms in the gut microbiota
In recent years, numerous works have established a strong correlation between the gut microbiota dysbiosis and the appearance of obesity, and its associated health problems such as dyslipidemia, metabolic syndrome, cardiovascular disease, atherosclerosis or the development of chronic inflammation.16,59,60 Hence, one alternative viewpoint of obesity treatment might be the modulation of the intestinal microbiota with bioactive compounds or live organisms (probiotics), with the main objective of restoring gut microbiota balance and neutralization of intestinal dysbiosis.
The metagenomic study showed a statistically significant increase in the alpha-diversity (Chao-1 diversity index, p = 0.028; and observed sample diversity index, p = 0.009) in the pA1c®-supplemented group compared with the HFS group (Fig. 3A). There is evidence that supports a relationship between lower alpha-diversity and higher body mass index,61 and between high alpha-diversity and weight loss.62 Furthermore, there are studies that have linked an increase in alpha-diversity with an improvement in cholesterol regulation and the prevention of hyperlipidemia and dyslipidemia.63 Therefore, we can assume that the probiotic supplementation, apart from increasing and improving the species richness of the intestinal microbiota, may directly contribute to a reduction of body weight and the normalization of the cholesterol profile, thus contributing to avoid the onset of dyslipidemia.
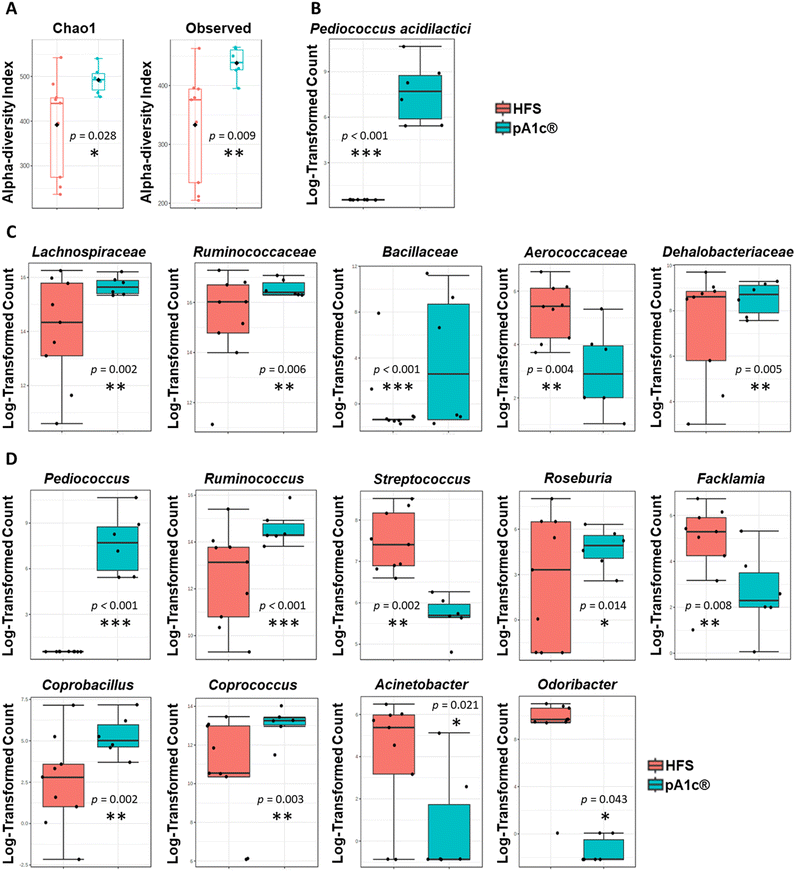 |
| Fig. 3 Alpha-diversity analyses (A), presence of the supplemented probiotic Pediococcus acidilactici pA1c® (B), and statistically significant differences in families (C) and genera (D), between the pA1c®- and HFS-supplemented groups. Metagenomic statistical analyses and the abundance of the bacteria were calculated using Metagenome-seq, with log-transformed bacterial 16S rRNA gene copy counts (*p < 0.05; **p < 0.01; and ***p < 0.001 with respect to the HFS group). | |
A significant increase in the abundance of Pediococcus acidilactici (species to which the probiotic strain pA1c® administered to the supplemented rats belongs) was detected in the pA1c® group compared to the HFS group (Fig. 3B). This species appeared in the feces of all the pA1c®-supplemented rats. Hence, these findings support the idea that pA1c® is able to colonize the colon and settle in the intestine with 100% effectiveness in only nine weeks of supplementation. Microbiota analyses performed through 16S evidenced significant differences in the abundance of five families between the pA1c®- and HFS-supplemented groups. pA1c® increased the abundance of the Lachnospiraceae, Ruminococcaceae, Bacillaceae and Dehalobacteriaceae families and decreased that of Aerococcaceae (Fig. 3C). It is well established that the Lachnospiraceae and Ruminococcaceae families are butyrate-producing bacteria and have been negatively correlated with obesity.64,65 Moreover, different studies correlate increased abundance of these bacterial families with an alleviation of obesity in high fat rodents by enhancing gut barrier function, reducing inflammation, ameliorating weight gain, mitigating liver function damage and alleviating insulin resistance.64,66–68 On the other hand, it is still unclear whether the Bacillaceae family plays a role in obesity and, if it does, what function it exerts. However, in recent years there has been a boom in the use of probiotics from the Bacillaceae family as an alternative treatment for obesity.69–72 Therefore, an increase in the abundance of this family might be beneficial for obesity-related disturbances. Furthermore, obesity has been related with a decreased abundance of several groups within the class Clostridia, including Dehalobacteriaceae.73 Additionally, previous studies of our group have revealed that the family Dehalobacteriaceae was involved in inflammation, being one of the main overexpressed families in subjects with low-inflammatory index compared with individuals with high-inflammatory index,74 so its increase after pA1c® supplementation would evidence the anti-obesogenic and anti-inflammatory capacity of pA1c® against chronic inflammation and obesity. Other scientific investigations have reported Aerococcaceae as a pathogenic micro-organism that may be involved in human infections;75 thus, its abundance decline after pA1c®-supplementation should be understood as a protective mechanism of defence of the gut microbiota against external infections.
Regarding genus, the nine-week pA1c®-supplementation increased the abundance of Pediococcus, Ruminococcus, Roseburia, Coprobacillus and Coprococcus, and decreased the levels of the Streptococcus, Facklamia, Acinetobacter and Odoribacter genera when compared with the HFS group (Fig. 3D). Over the last few years, probiotics derived from the Pediococcus genus, including the Pediococcus acidilactici strains, have been studied in the context of obesity. Numerous studies have reported that an increase in the abundance of Pediococcus was associated with lower fat accumulation, lower serum TG and cholesterol levels, reduced liver damage, lower inflammation and ameliorated obesity-related dyslipidemia through the modulation of the gut microbiota.23,30–32,76–79 Besides, butyrate has emerged with vast beneficial effects on energy metabolism, intestinal homeostasis and immune response regulation that seems to play an important role in the development and alleviation of obesity. Butyrate is a small-chain fatty acid (SCFA) organic metabolite produced by the fermentation of dietary fibre and resistant starch. Several studies have provided a protective role of butyrate against inflammation, hypercholesterolemia, obesity and obesity-related diseases.80,81 Thus, the overexpression of butyrate-producing bacteria, such as the genera Ruminococcus, Roseburia and Coprococcus,82–84 could be beneficial in relation to dyslipidemia, inflammation and obesity. Moreover, aside from the butyrate-producing effect, the literature describes Roseburia as a genus that is negatively correlated with obesity and liver steatosis85 while Coprococcus, together with Coprobacillus, have been reported as bacteria that correlate negatively with serum biochemical and cholesterol metabolic parameters,86 shedding some light on the hepatic biomarkers, TG and cholesterol parameter reduction observed above. In contrast, Streptococcus is positively correlated with obesity, inflammation and weight gain, and is found to be decreased in the HFS diet-induced obese mice through the effect of SCFA,87,88 while Facklamia is an opportunistic genus that tends to increase in abundance in obesity-related problems such as type-2 diabetes and insulin resistance.89 Furthermore, different authors have established a positive relationship between the abnormal increase in body weight, visceral fat, fat mass, and the abundance of Acinetobacter.88,90 Additional authors have reported that a Sanghuangporus vaninii mixture ameliorated obesity related type-2 diabetes and altered intestinal microbiota by reducing the abundance of Odoribacter.91
Finally, a predictive analysis of the metabolic function of the intestinal microbiota was performed using Tax4Fun (ESI Table 2†). According to our gene expression analysis data, the prediction confirmed differences in the signalling pathways related to lipid and carbohydrate metabolism.
3.5. pA1c® correlates negatively with Streptococcus, cholesterol parameters, mesenteric fat, triglycerides and liver damage
In order to demonstrate whether the presence of Pediococcus acidilactici in animal feces could be related to the improvements observed in biochemical and physiological parameters, as well as to the presence or absence of other bacteria in the gut, we carried out different correlation analyses (ESI Fig. 1†). As expected, a statistically significant negative correlation was observed between pA1c® and cholesterol metabolism-related parameters: between pA1c® and total cholesterol (Spearman Rho: −0.7516, p value = 0.008), between pA1c® and total chol/HDL ratio (Spearman Rho: −0.7227, p value = 0.012), between pA1c® and TG levels (Spearman Rho: −0.7585, p value = 0.007), and between pA1c® and AIP (Spearman Rho: −0.6880, p value = 0.019). Moreover, a negative correlation was also observed between pA1c® and AST (Spearman Rho: −0.6302, p value = 0.039), and mesenteric fat (Spearman Rho: −0.7400, p value = 0.009). In contrast, a positive correlation was found between pA1c® and the gastrocnemius muscle (Spearman Rho: −0.7400, p value = 0.009). Therefore, these results confirm once again the involvement of pA1c® in cholesterol metabolism, lipid regulation (fat accumulation in mesenteric fat) and metabolic diseases such as obesity and NAFLD (characterized by high TG content and liver damage).
Interestingly, we found a strong negative correlation between pA1c® and the genus Streptococcus (Spearman Rho: −0.8018, p value = 0.0003, Fig. 4A). Curiously, Streptococcus demonstrated a significant positive correlation with total cholesterol (Fig. 4B), total chol/HDL (Fig. 4C), and TG levels (Fig. 4D), just the opposite of pA1c®. Similar to our findings, recent studies have positively associated the genus Streptococcus with hypercholesterolemia,92 high TG level,93 dyslipidemia94 and obesity.92–94 Furthermore, certain works have revealed the potential use of Pediococcus acidilactici in the food industry, since it is able, through the bacteriocins it produces, to inhibit the growth of pathogenic microorganisms such as Streptococcus pyogenes95 and Streptococcus mutants,96 highlighting once again the negative association between both. Interestingly, Renye et al. have reported the contrary effect. They have found that one Streptococcus species produced a bacteriocin with anti-pediococcal activity, inhibiting the growth of Pediococcus.97 Therefore, it was hypothesized that there may be a competition between the species Pediococcus acidilactici (already described its anti-obesogenic, anti-inflammatory and anti-hypercholesterolemic activity) and the Streptococcus genus in the intestine; and depending on which bacterium is predominant, the host will have more (Streptococcus is predominant) or less (Pediococcus acidilactici is predominant) risk of developing metabolic diseases, such as obesity, hypercholesterolemia or hypertriglyceridemia.
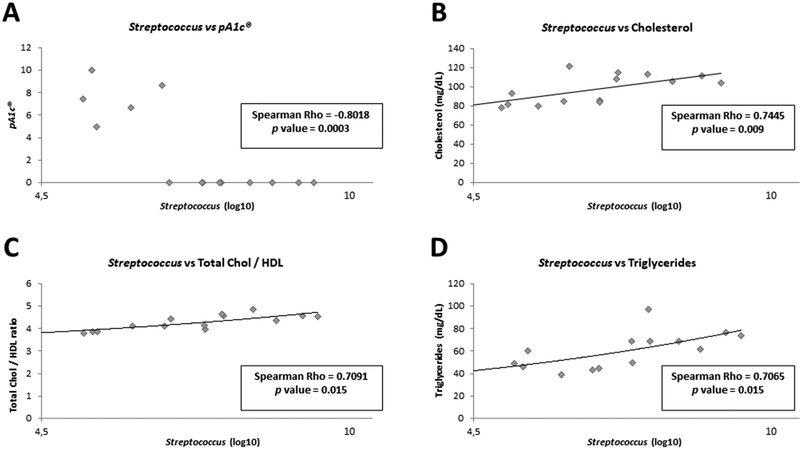 |
| Fig. 4 Spearman correlations between Streptococcus and pA1c® (A), between Streptococcus and cholesterol (B), between Streptococcus and total chol/HDL ratio (C), and between Streptococcus and triglycerides (D). | |
4. Conclusions
We have demonstrated that the administration of the probiotic strain pA1c® has beneficial effects on TG and cholesterol metabolism parameters, visceral adiposity, biomarkers of liver damage, and inflammatory markers in high-fat diet-induced obese rats. In addition, we have shown that the main metabolic pathways by which pA1c® acts on obesity are the activation of the peroxisomal- and mitochondrial-fatty acid beta-oxidation process and inhibition of lipogenesis, which led to a reduction in inflammation. Furthermore, pA1c® would modulate the gut microbiota by increasing the microbial diversity, favouring SCFA-producing bacteria and competing with obesity and dyslipidemia-associated bacteria, such as species of the genus Streptococcus. Although further research is necessary, our data suggest pA1c® as a potential agent for the management of obesity and related comorbidities such as chronic inflammation, dyslipidemia, and dysbiosis.
Author contributions
Conceptualization: Deyan Yavorov-Dayliev, Fermín I. Milagro, Miguel López-Yoldi, and Paula Aranaz; data curation: Deyan Yavorov-Dayliev, Miguel López-Yoldi, and Paula Aranaz; formal analysis: Deyan Yavorov-Dayliev; funding acquisition: Fermín I. Milagro; investigation: Deyan Yavorov-Dayliev, Fermín I. Milagro, Miguel López-Yoldi, Josune Ayo, María Oneca, and Paula Aranaz; methodology: Deyan Yavorov-Dayliev, Fermín I. Milagro, Miguel López-Yoldi, and Paula Aranaz; project administration: Fermín I. Milagro; resources: Deyan Yavorov-Dayliev, Fermín I. Milagro, Miguel López-Yoldi, Iñigo Clemente, José Ignacio Riezu-Boj, Josune Ayo, María Oneca, and Paula Aranaz; software: Deyan Yavorov-Dayliev, Iñigo Clemente, and José Ignacio Riezu-Boj; supervision: Fermín I. Milagro, Josune Ayo, and Paula Aranaz; validation: Fermín I. Milagro and Paula Aranaz; visualization: Fermín I. Milagro and Paula Aranaz; writing – original draft: Deyan Yavorov-Dayliev; writing – review & editing: Fermín I. Milagro, Josune Ayo, María Oneca, and Paula Aranaz.
Conflicts of interest
Josune Ayo is a shareholder of the company Genbioma Aplicaciones S.L., and Josune Ayo and María Oneca are co-authors of the patent “Probiotics for regulating blood glucose [WO2021/123355A1]”. The rest of the authors declare no conflicts of interest. Deyan Yavorov received a predoctoral grant from the Government of Navarra (Ayudas para la contratación de doctorandos y doctorandas “Doctorados industriales 2020”) [Reference: 0011-1408-2020-000010].
Acknowledgements
The authors thank Goyo Sanzol and Pentabiol S.L for the deposit of the P. acidilactici pA1c® strain. The authors acknowledge the partners of Genbioma Aplicaciones S.L. for their constructive criticism in the development of this manuscript, as well as Luís Gosálbez (Sandwalk BioVentures S.L.).
References
- B. Conway and A. Rene, Obesity as a disease: no lightweight matter, Obes. Rev., 2004, 5, 145–151 CrossRef CAS
.
- C. M. Apovian,
et al., Obesity: definition, comorbidities, causes, and burden, Am. J. Manag. Care, 2016, 22, s176–s185 Search PubMed
.
- J. Vekic, A. Zeljkovic, A. Stefanovic, Z. Jelic-Ivanovic and V. Spasojevic-Kalimanovska,
et al., Obesity and dyslipidemia, Metabolism, 2019, 92, 71–81 CrossRef CAS
.
- R. Franssen, H. Monajemi, E. S. G. Stroes and J. J. P. Kastelein,
et al., Obesity and Dyslipidemia, Med. Clin. North Am., 2011, 95, 893–902 CrossRef CAS
.
- M. Auley and M. T,
et al., Effects of obesity on cholesterol metabolism and its implications for healthy ageing, Nutr. Res. Rev., 2020, 33, 121–133 CrossRef
.
- A. J. Cox, N. P. West and A. W. Cripps, Obesity, inflammation, and the gut microbiota, Lancet Diabetes Endocrinol., 2015, 3, 207–215 CrossRef CAS
.
- T. Kawai, M. V. Autieri and R. Scalia, Inflammation: From Cellular Mechanisms to Immune Cell Education: Adipose tissue inflammation and metabolic dysfunction in obesity, Am. J. Physiol.: Cell Physiol., 2021, 320, C375 CrossRef CAS
.
- O. Izaola, D. de Luis, I. Sajoux, J. C. Domingo and M. Vidal, Inflammation and obesity (lipoinflammation), Nutr. Hosp., 2015, 31, 2352–2358 CAS
.
- R. Monteiro and I. Azevedo, Chronic Inflammation in Obesity and the Metabolic Syndrome, Mediators Inflammation, 2010, 2010 DOI:10.1155/2010/289645
.
- S. M. Wright and L. J. Aronne, Causes of obesity, Abdom. Imaging, 2012, 37, 730–732 CrossRef
.
- K. M. Flegal, M. D. Carroll, C. L. Ogden and L. R. Curtin, Prevalence and trends in obesity among US adults, 1999–2008, J. Am. Med. Assoc., 2010, 303, 235–241 CrossRef CAS
.
- G. Nittari,
et al., Fighting obesity in children from European World Health Organization member states. Epidemiological data, medical-social aspects, and prevention programs, Clin. Term., 2019, 170, E223–E230 CAS
.
- L. Abenavoli,
et al., Gut Microbiota and Obesity: A Role for Probiotics, Nutrients, 2019, 11 DOI:10.3390/nu11112690
.
- A. C. Gomes, C. Hoffmann and J. F. Mota, The human gut microbiota: Metabolism and perspective in obesity, Gut Microbes, 2018, 9, 308 CAS
.
- J. Debédat, K. Clément and J. Aron-Wisnewsky, Gut Microbiota Dysbiosis in Human Obesity: Impact of Bariatric Surgery, Curr. Obes. Rep., 2019, 8, 229–242 CrossRef
.
- E. Amabebe, F. O. Robert, T. Agbalalah and E. S. F. Orubu, Microbial dysbiosis-induced obesity: role of gut microbiota in homoeostasis of energy metabolism, Br. J. Nutr., 2020, 123, 1127–1137 CrossRef CAS
.
-
R. Pahwa, A. Goyal and I. Jialal, Chronic Inflammation, in Pathobiology of Human Disease: A Dynamic Encyclopedia of Disease Mechanisms, 2022, pp. 300–314. DOI:10.1016/B978-0-12-386456-7.01808-6
.
-
J. S. Messer and E. B. Chang, Microbial Physiology of the Digestive Tract and Its Role in Inflammatory Bowel Diseases, in Physiology of the Gastrointestinal Tract, 6th edn, 2018, vol. 1, pp. 795–810 Search PubMed
.
- E. Ansaldo, T. K. Farley and Y. Belkaid, Control of Immunity by the Microbiota, Annu. Rev. Immunol., 2021, 39, 449–479 CrossRef CAS
.
- Y. Belkaid and O. J. Harrison, Homeostatic Immunity and the Microbiota, Immunity, 2017, 46, 562–576 CrossRef CAS
.
- Z. A. Bander, M. D. Nitert, A. Mousa and N. Naderpoor, The Gut Microbiota and Inflammation: An Overview, Int. J. Environ. Res. Public Health, 2020, 17, 1–22 Search PubMed
.
- G. Barbara,
et al., Inflammatory and Microbiota-Related Regulation of the Intestinal Epithelial Barrier, Front. Nutr., 2021, 8 DOI:10.3389/fnut.2021.718356
.
- D. Yavorov-dayliev, F. I. Milagro, J. Ayo, M. Oneca and P. Aranaz, Pediococcus acidilactici CECT9879 (pA1c) Counteracts the Effect of a High-Glucose Exposure in C. elegans by Affecting the Insulin Signaling Pathway (IIS), Int. J. Mol. Sci., 2022, 23 DOI:10.3390/ijms23052689
.
- M. Cabello-Olmo,
et al., Antidiabetic Effects of Pediococcus acidilactici pA1c on HFD-Induced Mice, Nutrients, 2022, 14 DOI:10.3390/nu14030692
.
- J. C. Fernández-Macías, A. C. Ochoa-Martínez, J. A. Varela-Silva and I. N. Pérez-Maldonado, Atherogenic Index of Plasma: Novel Predictive Biomarker for Cardiovascular Illnesses, Arch. Med. Res., 2019, 50, 285–294 CrossRef
.
- B. de Cuevillas,
et al., Possible metabolic interplay between quality of life and fecal microbiota in a presenior population: Preliminary results, Nutrition, 2022, 103–104, 111841 CrossRef CAS
.
- C. H. L. Dinh,
et al., Bardoxolone Methyl Prevents Mesenteric Fat Deposition and Inflammation in High-Fat Diet Mice, Sci. World J., 2015, 2015 DOI:10.1155/2015/549352
.
- E. Karaskova,
et al., Role of Adipose Tissue in Inflammatory Bowel Disease, Int. J. Mol. Sci., 2021, 22 DOI:10.3390/ijms22084226
.
- L. I. Kredel and B. Siegmund, Adipose-tissue and intestinal inflammation - visceral obesity and creeping fat, Front. Immunol., 2014, 5 DOI:10.3389/fimmu.2014.00462
.
- F. T. Lim, S. M. Lim and K. Ramasamy, Cholesterol lowering by Pediococcus acidilactici LAB4 and Lactobacillus plantarum LAB12 in adult zebrafish is associated with improved memory and involves an interplay between npc1l1 and abca1, Food Funct., 2017, 8, 2817–2828 RSC
.
- F. T. Lim, S. M. Lim and K. Ramasamy, Pediococcus acidilactici LAB4 and Lactobacillus plantarum LAB12 assimilate cholesterol and modulate ABCA1, CD36, NPC1L1 and SCARB1 in vitro, Benefic. Microbes, 2017, 8, 97–109 CrossRef CAS
.
- E. B. M. Daliri, Y. Kim, Y. Do, R. Chelliah and D. H. Oh, In Vitro and In Vivo Cholesterol Reducing Ability and Safety of Probiotic Candidates Isolated from Korean Fermented Soya Beans, Probiotics Antimicrob. Proteins, 2022, 14, 87–98 CrossRef CAS
.
- S. L. Deshmane, S. Kremlev, S. Amini and B. E. Sawaya, Monocyte Chemoattractant Protein-1 (MCP-1): An Overview, J. Interferon Cytokine Res., 2009, 29, 313 CrossRef CAS
.
- J. P. Rhoads and A. S. Major, How Oxidized Low-Density Lipoprotein Activates Inflammatory Responses, Crit. Rev. Immunol., 2018, 38, 333–342 CrossRef
.
- T. Tanaka, M. Narazaki and T. Kishimoto, IL-6 in inflammation, immunity, and disease, Cold Spring Harbor Perspect. Biol., 2014, 6 DOI:10.1101/cshperspect.a016295
.
- A. Engin, The Pathogenesis of Obesity-Associated Adipose Tissue Inflammation, Adv. Exp. Med. Biol., 2017, 960, 221–245 CrossRef CAS
.
- J.-P. Bastard,
et al., Recent advances in the relationship between obesity, inflammation, and insulin resistance, Eur. Cytokine Network, 2006, 17, 4–12 CAS
.
- H. Fang and R. L. Judd, Adiponectin Regulation and Function, Compr. Physiol., 2018, 8, 1031–1063 Search PubMed
.
- A. E. Achari and S. K. Jain, Adiponectin, a Therapeutic Target for Obesity, Diabetes, and Endothelial Dysfunction, Int. J. Mol. Sci., 2017, 18 DOI:10.3390/ijms18061321
.
- L. V. Vasileva, M. S. Savova, D. Tews, M. Wabitsch and M. I. Georgiev, Rosmarinic acid attenuates obesity and obesity-related inflammation in human adipocytes, Food Chem. Toxicol., 2021, 149, 112002 CrossRef CAS
.
- T. Y. Lin,
et al., IL-29 promoted obesity-induced inflammation and insulin resistance, Cell. Mol. Immunol., 2019, 17, 369–379 CrossRef
.
- L. V. Vasileva, A. S. Marchev and M. I. Georgiev, Causes and solutions to “globesity”: The new fa(s)t alarming global epidemic, Food Chem. Toxicol., 2018, 121, 173–193 CrossRef CAS
.
- Y. H. Han,
et al., Anti-obesity effects of Arctii Fructus (Arctium lappa) in white/brown adipocytes and high-fat diet-induced obese mice, Food Funct., 2016, 7, 5025–5033 RSC
.
- J. B. Seo,
et al., Anti-obesity effects of Lysimachia foenum-graecum characterized by decreased adipogenesis and regulated lipid metabolism, Exp. Mol. Med., 2011, 43, 205–215 CrossRef CAS
.
- M. Mueckler and B. Thorens, The SLC2 (GLUT) Family of Membrane Transporters, Mol. Aspects Med., 2013, 34, 121 CrossRef CAS
.
- B. Liu, C. Deng and P. Tan, Ombuin ameliorates diabetic nephropathy in rats by anti-inflammation and antifibrosis involving Notch 1 and PPAR γ signaling pathways, Drug Dev. Res., 2022, 83, 1270–1280 CrossRef CAS
.
- B. Li,
et al., Luteolin alleviates inflammation and modulates gut microbiota in ulcerative colitis rats, Life Sci., 2021, 269 DOI:10.1016/j.lfs.2020.119008
.
- D. A. Youssef, H. M. El-Fayoumi and M. F. Mahmoud, Beta-caryophyllene protects against diet-induced dyslipidemia and vascular inflammation in rats: Involvement of CB2 and PPAR-γ receptors, Chem.-Biol. Interact., 2019, 297, 16–24 CrossRef CAS
.
- D. Eberlé,
et al., SREBF-1 gene polymorphisms are associated with obesity and type 2 diabetes in French obese and diabetic cohorts, Diabetes, 2004, 53, 2153–2157 CrossRef
.
- D. Gonzalez-Bohorquez,
et al., FASN-dependent de novo lipogenesis is required for brain development, Proc. Natl. Acad. Sci. U. S. A., 2022, 119 DOI:10.1073/pnas.2112040119
.
- G. Costabile,
et al., Reduction of De Novo Lipogenesis Mediates Beneficial Effects of Isoenergetic Diets on Fatty Liver: Mechanistic Insights from the MEDEA Randomized Clinical Trial, Nutrients, 2022, 14 DOI:10.3390/nu14102178
.
- F. W. B. Sanders and J. L. Griffin, De novo lipogenesis in the liver in health and disease: More than just a shunting yard for glucose, Biol. Rev., 2016, 91, 452–468 CrossRef
.
- C. Beysen,
et al., Inhibition of fatty acid synthase with FT-4101 safely reduces hepatic de novo lipogenesis and steatosis in obese subjects with non-alcoholic fatty liver disease: Results from two early-phase randomized trials, Diabetes, Obes. Metab., 2021, 23, 700–710 CrossRef CAS
.
- S. Qin,
et al., Dietary fibre alleviates hepatic fat deposition via inhibiting lipogenic gene expression in meat ducks, J. Anim. Physiol. Anim. Nutr., 2018, 102, e736–e745 CrossRef CAS
.
- D. Yavorov-Dayliev,
et al., Glucose-lowering effects of a synbiotic combination containing Pediococcus acidilactici in C. elegans and mice, Diabetologia, 2023 DOI:10.1007/s00125-023-05981-w
.
- C. Sztalryd and D. L. Brasaemle, The perilipin family of lipid droplet proteins: Gatekeepers of intracellular lipolysis, Biochim. Biophys. Acta, Mol. Cell Biol. Lipids, 2017, 1862, 1221–1232 CrossRef CAS
.
- B. K. S. Hirata,
et al., Potential anti-obesogenic effects of Ginkgo biloba observed in epididymal white adipose tissue of obese rats, Front. Endocrinol., 2019, 10, 284 CrossRef
.
- I. Warnke,
et al., Combinations of bio-active dietary constituents affect human white adipocyte function in vitro, Nutr. Metab., 2016, 13, 1–10 CrossRef
.
- I. J. Malesza,
et al., High-Fat, Western-Style Diet, Systemic Inflammation, and Gut Microbiota: A Narrative Review, Cells, 2021, 10 DOI:10.3390/cells10113164
.
- M. Green, K. Arora and S. Prakash, Microbial Medicine: Prebiotic and Probiotic Functional Foods to Target Obesity and Metabolic Syndrome, Int. J. Mol. Sci., 2020, 21 DOI:10.3390/ijms21082890
.
- M. A. Stanislawski, D. Dabelea, L. A. Lange, B. D. Wagner and C. A. Lozupone, Gut microbiota phenotypes of obesity, npj Biofilms Microbiomes, 2019, 5 DOI:10.1038/s41522-019-0091-8
.
- D. A. Koutoukidis,
et al., The association of weight loss with changes in the gut microbiota diversity, composition, and intestinal permeability: a systematic review and meta-analysis, Gut Microbes, 2022, 14 DOI:10.1080/19490976.2021.2020068
.
- M. Xiao,
et al., Effects of Torreya grandis Kernel Oil on Lipid Metabolism and Intestinal Flora in C57BL/6J Mice, Oxid. Med. Cell. Longevity, 2022, 2022 DOI:10.1155/2022/4472751
.
- J. Ye,
et al., Pu-erh tea ameliorates obesity and modulates gut microbiota in high fat diet fed mice, Food Res. Int., 2021, 144 DOI:10.1016/j.foodres.2021.110360
.
- L. Costantini, R. Molinari, B. Farinon and N. Merendino, Impact of Omega-3 Fatty Acids on the Gut Microbiota, Int. J. Mol. Sci., 2017, 18 DOI:10.3390/ijms18122645
.
- L. Ma,
et al., Spermidine improves gut barrier integrity and gut microbiota function in diet-induced obese mice, Gut Microbes, 2020, 12, 1–19 Search PubMed
.
- Q. Zhao,
et al., Adzuki Bean Alleviates Obesity and Insulin Resistance Induced by a High-Fat Diet and Modulates Gut Microbiota in Mice, Nutrients, 2021, 13 DOI:10.3390/nu13093240
.
- D. Zhao,
et al., Beneficial impacts of fermented celery (Apium graveolens L.) juice on obesity prevention and gut microbiota modulation in high-fat diet fed mice, Food Funct., 2021, 12, 9151–9164 RSC
.
- X. Lu,
et al., Bacillus licheniformis Zhengchangsheng® Inhibits Obesity by Regulating the AMP-Activated Protein Kinase Signaling Pathway, Probiotics Antimicrob. Proteins, 2021, 13, 1658–1667 CrossRef CAS
.
- Z. Zeng,
et al., Bacillus amyloliquefaciens SC06 alleviates the obesity of ob/ob mice and improves their intestinal microbiota and bile acid metabolism, Food Funct., 2022, 13 10.1039/d1fo03170h
.
- J. Huang,
et al., Enterococcus faecium R0026 Combined with Bacillus subtilis R0179 Prevent Obesity-Associated Hyperlipidemia and Modulate Gut Microbiota in C57BL/6 Mice, J. Microbiol. Biotechnol., 2021, 31, 181–188 CrossRef
.
- K. D. Lee,
et al., Novel Bacillus ginsengihumi CMRO6 Inhibits Adipogenesis via p38MAPK/Erk44/42 and Stimulates Glucose Uptake in 3T3-L1 Pre-Adipocytes through Akt/AS160 Signaling, Int. J. Mol. Sci., 2022, 23 DOI:10.3390/ijms23094727
.
- B. A. Peters,
et al., A taxonomic signature of obesity in a large study of American adults, Sci. Rep., 2018, 8 DOI:10.1038/s41598-018-28126-1
.
- P. Aranaz,
et al., A predictive regression model of the obesity-related inflammatory status based on gut microbiota composition, Int. J. Obes., 2021, 45, 2261–2268 CrossRef CAS
.
-
E. Stackebrandt, The family aerococcaceae, in The Prokaryotes: Firmicutes and Tenericutes, 2014, pp. 3–6, ISBN 9783642301209 Search PubMed
.
- K. Barathikannan,
et al., Anti-Obesity Efficacy of Pediococcus acidilactici MNL5 in Canorhabditis elegans Gut Model, Int. J. Mol. Sci., 2022, 23 DOI:10.3390/ijms23031276
.
- X. Zhao,
et al., The obesity and fatty liver are reduced by plant-derived Pediococcus pentosaceus LP28 in high fat diet-induced obese mice, PLoS One, 2012, 7 DOI:10.1371/journal.pone.0030696
.
- Y. Wang,
et al., Pediococcus pentosaceus PP04 improves high-fat diet-induced liver injury by the modulation of gut inflammation and intestinal microbiota in C57BL/6N mice, Food Funct., 2021, 12, 6851–6862 RSC
.
- T. Ueda,
et al., Effects of Pediococcus acidilactici R037 on Serum Triglyceride Levels in Mice and Rats after Oral Administration, J. Nutr. Sci. Vitaminol., 2018, 64, 41–47 CrossRef CAS
.
- S. Coppola, C. Avagliano, A. Calignano and R. Berni Canani, The Protective Role of Butyrate against Obesity and Obesity-Related Diseases, Molecules, 2021, 26 DOI:10.3390/molecules26030682
.
- S. M. McNabney and T. M. Henagan, Short Chain Fatty Acids in the Colon and Peripheral Tissues: A Focus on Butyrate, Colon Cancer, Obesity and Insulin Resistance, Nutrients, 2017, 9 DOI:10.3390/nu9121348
.
- F. Liu,
et al., Fructooligosaccharide (FOS) and Galactooligosaccharide (GOS) Increase Bifidobacterium but Reduce Butyrate Producing Bacteria with Adverse Glycemic Metabolism in healthy young population, Sci. Rep., 2017, 7 DOI:10.1038/s41598-017-10722-2
.
- P. Louis and H. J. Flint, Formation of propionate and butyrate by the human colonic microbiota, Environ. Microbiol., 2017, 19, 29–41 CrossRef CAS
.
- W. Zhang, J. H. Xu, T. Yu and Q. K. Chen, Effects of berberine and metformin on intestinal inflammation and gut microbiome composition in db/db mice, Biomed. Pharmacother., 2019, 118, 109131 CrossRef CAS
.
- G. Yuan, M. Tan and X. Chen, Punicic acid ameliorates obesity and liver steatosis by regulating gut microbiota composition in mice, Food Funct., 2021, 12, 7897–7908 RSC
.
- H. Wang,
et al., Dietary wood pulp-derived sterols modulation of cholesterol metabolism and gut microbiota in high-fat-diet-fed hamsters, Food Funct., 2019, 10, 775–785 RSC
.
- Y. Lan,
et al., Seabuckthorn polysaccharide ameliorates high-fat diet-induced obesity by gut microbiota-SCFAs-liver axis, Food Funct., 2022, 13, 2925–2937 RSC
.
- J. J. Y. Sung,
et al., Gastric microbes associated with gastric inflammation, atrophy and intestinal metaplasia 1 year after Helicobacter pylori eradication, Gut, 2020, 69, 1572–1580 CrossRef CAS
.
- A. U. Rehman,
et al., Morchella esculenta mushroom polysaccharide attenuates diabetes and modulates intestinal permeability and gut microbiota in a type 2 diabetic mice model, Front. Nutr., 2022, 9 DOI:10.3389/fnut.2022.984695
.
- Z. Miao,
et al., Lacticaseibacillus paracasei K56 Attenuates High-Fat Diet-Induced Obesity by Modulating the Gut Microbiota in Mice, Probiotics Antimicrob. Proteins, 2022 DOI:10.1007/S12602-022-09911-X
.
- Z. Huang,
et al., Sanghuangporus vaninii mixture ameliorated type 2 diabetes mellitus and altered intestinal microbiota in mice, Food Funct., 2022, 13, 11758–11769 RSC
.
- L. Ding,
et al., Modulation of gut microbiota and fecal metabolites by corn silk among high-fat diet-induced hypercholesterolemia mice, Front. Nutr., 2022, 9 DOI:10.3389/fnut.2022.935612
.
- Y. Lan,
et al., Seabuckthorn polysaccharide ameliorates high-fat diet-induced obesity by gut microbiota-SCFAs-liver axis, Food Funct., 2022, 13, 2925–2937 RSC
.
- Q. Deng,
et al., Gougunao tea polysaccharides ameliorate high-fat diet-induced hyperlipidemia and modulate gut microbiota, Food Funct., 2023, 14 10.1039/d2fo01828d
.
- S. Abbasiliasi,
et al., In vitro assessment of Pediococcus acidilactici Kp10 for its potential use in the food industry, BMC Microbiol., 2017, 17 DOI:10.1186/s12866-017-1000-z
.
- A. Sharma,
et al., Biomedical applications of L-alanine produced by Pediococcus acidilactici BD16 (alaD+), Appl. Microbiol. Biotechnol., 2022, 106, 1435–1446 CrossRef CAS
.
- J. A. Renye and G. A. Somkuti, BlpC-regulated bacteriocin production in Streptococcus thermophilus, Biotechnol. Lett., 2013, 35, 407–412 CrossRef CAS
.
|
This journal is © The Royal Society of Chemistry 2023 |
Click here to see how this site uses Cookies. View our privacy policy here.