DOI:
10.1039/D2FO03338K
(Paper)
Food Funct., 2023,
14, 133-147
Dietary leucine and fish oil cooperatively regulate skeletal myofiber type transformation via the CaMKII signaling pathway of pigs†
Received
4th November 2022
, Accepted 5th December 2022
First published on 9th December 2022
Abstract
The study investigated the effects of dietary leucine (Leu) and fish oil (FO) on skeletal myofiber type transformations in pigs and their potential interactions. The results showed that Leu increased the content of Leu, upregulated myocyte enhancer factor-2C (MEF2C) and activated the CaMKII-AMPK/SIRT1-PGC-1α pathway in the longissimus dorsi (LD) muscle. FO increased adiponectin and fatty acid beta-oxidation of LD muscle. Activation of the adiponectin signaling pathway by FO further enhanced the CaMKII pathway and upregulated the expression of MEF2C. Moreover, we found that Leu increased cyclic AMP and caffeine, and FO increased linoleic acid and glutamine in muscle metabolites, which may be the cause of myofiber conversion. In conclusion, this study demonstrated that dietary Leu and FO co-regulated the transformation from glycolytic to oxidative skeletal myofiber type. It is hypothesized that there is an interaction between amino acids and polyunsaturated fatty acids, possibly via the CaMKII signaling pathway to upregulate MEF2 and mitochondrial biogenesis.
1. Introduction
With the development and progress of the economy, consumers are demanding higher quality pork. The histochemical and biochemical properties of the skeletal muscle, such as myofiber type composition, fiber size, oxidative and glycolytic capacity, glycogen and fatty acid content, are the major aspects that determine the quality of pork.1 Skeletal myofiber types are categorized as type I oxidative and type II enzymatic, in accordance with the different metabolic pathways of ATP production and the corresponding contraction patterns, with the oxidative type being enriched with mitochondria and suitable for sustained contraction, and the enzymatic type mainly depending on anaerobic glycolysis for energy.2 On the other hand, porcine skeletal myofibers with respect to the expression of myosin heavy chain (MyHC) isoforms can be specifically subdivided into I, IIa, IIx and IIb.3 Increased proportions of type I oxidative myofibers may improve meat color, pH and flavor scores of pork, and intramuscular fat content is positively correlated with the proportion of type I myofibers.4 Therefore, augmenting the proportion of oxidative myofibers through the modulation of mitochondrial biogenesis and energy metabolism would be an effective way to improve pork quality. Leucine (Leu) has been extensively demonstrated to boost protein synthesis and the growth of skeletal muscle in humans,5 mice6 and pigs.7,8 Our previous studies have indicated that Leu has an effect on skeletal myofiber type shifting in pigs.9–11 Fish oil (FO) is enriched in omega-3 polyunsaturated fatty acids (PUFA). For porcine production applications, FO is added to feeds to enhance the growth and immunity of weaned piglets or to optimize the fatty acid composition and quality of pork in finishing pigs, and less research has been done on the myofiber type transformation,12,13 while studies in humans have shown that FO-derived omega-3 PUFA stimulates muscle growth and enhances mass.14,15 Furthermore, the primary and secondary fibers appear in pigs at the embryonic and fetal developmental stages, respectively, after which the total number of fibers is fixed, then the piglet stage is a highly efficient and rapid period for muscle fiber type conversion.16,17 Amino acids, most notably branched-chain amino acids (BACC) represented by Leu, and fatty acids, such as PUFA rich in FO, are two important nutrients which are effective at improving the formation of muscle fiber types. Therefore, it is possible to combine two nutrients, such as Leu and FO, at the early age stage to evaluate the impact of skeletal myofiber type transformation of pigs.
2. Materials and methods
2.1 Animals and experimental diets
Forty healthy pigs (Duroc × Landrace × Yorkshire, barrow, 35 days) with an average weight of 7.64 ± 0.13 kg were randomly allocated into four experimental groups following a 2 × 2 factorial arrangement: non-addition of Leu but the addition of 3.5% corn oil (CO) (N-Leu/A-CO), non-addition of Leu but the addition of 3.5% FO (N-Leu/FO), addition of 0.5% Leu and 3.5% CO (A-Leu/CO), and addition of 0.5% Leu and 3.5% FO (A-Leu/FO). The trial was conducted with 10 replicates per treatment, one pig per replicate, for 45 d. The diets (meal) were designed according to the NRC18 and the porcine ideal amino acid model, and the composition and nutritional levels of the protocol are presented in Table S1.† The location of the feeding site was at the Animal Experimental Building of the Institute of Subtropical Agriculture, Chinese Academy of Sciences. Each piglet was housed individually in a cage (1.80 × 1.10 m) and had free access to food and clean drinking water. Feed intake was recorded daily. The protocol for the administration of animals was performed in strict accordance with the Animal Management Rules of the Ministry of Health of the People's Republic of China and approved by the Animal Care and Use Committee of the Institute of Subtropical Agriculture, Chinese Academy of Sciences (Changsha, China).
2.2 Sample collection
At day 45 of the experiment, the final weights were recorded, then all pigs were slaughtered by electro stunning and bloodletting. Before slaughter, blood samples were taken from the jugular vein, centrifuged at 3000g for 15 minutes at 4 °C to generate serum, and then stored at −80 °C until additional analysis. Samples of the longissimus dorsi (LD) muscle tissue were rapidly separated from the right side of the carcass of ribs 6–7 and immediately frozen in liquid nitrogen. Afterwards, all muscle tissue samples were deposited in an ultra-low temperature refrigerator at −80 °C and were held for subsequent analyses. The LD muscle samples were synchronously obtained and fixed in 4% formaldehyde or 2.5% glutaraldehyde fixative for subsequent observation in sections.
2.3 Serum biochemistry and cytokines
The levels of glucose (GLU), total triglycerides (TG), total cholesterol (TC), low-density-lipoprotein cholesterol (LDL) and high-density-lipoprotein cholesterol (HDL) were analyzed. Utilizing commercial kits (Leadman Biotech Limited, Beijing, China) and biochemical analytical instruments (Beckman CX4; Beckman Coulter, Germany), all of these indications were examined. The serum cytokine levels of insulin, insulin-like growth factor 1 (IGF1), myostatin (MSTN), chemerin, fibroblast growth factors 21 (FGF 21), leptin, interleukin 6 and adiponectin were measured by a commercial ELISA kit (Cloud-Clone Corp., Wuhan, China).
2.4 Muscle chemical composition and enzyme activity
Approximately 30 g of fresh LD muscle samples were weighed for an individual pig. After freezing, the muscle was sliced and then re-frozen at −80 °C for 2 hours. The samples were placed in a freeze dryer (Labconco Inc., Kansas City, MO, USA) for 72 h at −50 °C and subsequently re-weighed. The moisture percentage was ascertained by calculating the difference between the initial weight and the weight of the dried muscle samples. Dried muscle samples were powdered and then analyzed for free amino acid composition. Muscle powder was dissolved in 0.01 M hydrochloric acid and 8% sulfosalicylic acid was added to precipitate the protein, which was filtered through a 0.22 μm filter membrane and then placed on an amino acid auto analyzer (Hitachi L8900, Japan) for free amino acid detection. Fresh LD muscle was assayed for lactate, glycogen, glucose, glucose-6-phosphate, creatine phosphate, hexokinase and mATPase, and the kits were purchased from Changsha Aoki Biotechnology Co. (Changsha, Hunan, China). The glycolytic potential (GP) was calculated as glycolytic potential = lactate content + 2 × (glycogen content + glucose content + glucose-6-phosphate content).
2.5 Muscle immunohistochemistry, tissue histology and transmission electron microscopy
The LD muscle sample fixed in 4% formaldehyde was embedded in paraffin and paraffin sections were made using a slicer (Leica, Germany). The paraffin sections were dewaxed in a series of processes using xylene, ethanol and distilled water and then antigenically repaired with EDTA antigen repair buffer (Servicebio, G1206). After the sections were dried for a period of time (approximately 10 minutes), circles were drawn around the slices with a histochemical pen (to prevent antibody run-off), an autofluorescence quencher (Servicebio, G1221) was added to the just-drawn circles for 5 min, and the sections were rinsed under flowing water for 10 min. The circular sections were then incubated for 30 min in BSA. After the removal of the blocking solution, sections were incubated with the FAST MyHC (Abcam, Eugene, OR, USA; CAT) antibody and Slow MyHC (Abcam; cat. Ab11803) antibody (1
:
500) at 4 °C overnight. Following washing, sections were incubated with secondary antibody (1
:
300, Servicebio) for 50 min at room temperature in the dark. After phosphate buffer washing, sections were exposed to DAPI (Servicebio, G1012) for 10 minutes at room temperature while remaining in the dark. After thorough washing, the sections were gently dried, sealed with an anti-fluorescence quenching sealer, and photographs were taken using a fluorescent microscope (Nikon, Tokyo, Japan). After dehydration in a series of ethanol (70–100%), the LD muscle tissue was paraffin embedded and cut into 2 μm slices with a microtome (Leica, Germany) before being stained with hematoxylin and eosin. Tissue sections were photographed using a microscope (Eclise Ci-L, Nikon, Japan) at 200× magnification. Digital images were quantitatively examined using ImageJ software (US National Institutes of Health, USA).
The LD muscle samples were taken out of the 2.5% glutaraldehyde fixative and fixed for 2 h at room temperature (20 °C) in 1% osmium acid–0.1 M phosphate buffer PB (pH 7.4), then gradient dehydrated with acetone, permeabilised with 812 embedding agent overnight, and embedded in resin. After 48 hours in a 60 °C incubator, 60–80 nm ultrathin sections were made using an ultrathin sectioning machine (Leica, Germany), followed by double staining with uranyl acetate–lead citrate. Following washing with water, images were photographed under a transmission electron microscope (Hitachi, Japan), and the length of the muscle segments, the distance between the Z-lines, was measured using ImageJ software.
2.6 Reverse transcription and real-time quantitative PCR
Total RNA of LD muscle was extracted using a TRIzol reagent (15596-026 Invitrogen). We then identified the concentration and quality of RNA with a NanoDrop ND-1000 spectrophotometer (Thermo Scientific, Waltham, MA). Gene sequences were obtained from NCBI and primers were designed using Primer Premier 5 software; the primer sequences are listed in Table 1. All real-time PCR reagents were mixed in LightCycler 480 384-well plates according to the operating protocol of the Takara Real-Time PCR Kit (RR096A; Takara Bio, Tokyo, Japan). There were two replicates for each sample. Relative gene expression was quantified on a Roche LightCycler 480II (Basel, Switzerland) using the real-time qPCR function. According to the 2−ΔΔCt formula, the expression of GAPDH genes was used to standardize the expression of other target genes.
Table 1 Primers used for quantitative real-time PCR
Gene |
Forward |
Reverse |
RefSeq |
MyHC-I, myosin heavy chain I; MyHC-IIa, myosin heavy chain IIa; MyHC-IIb, myosin heavy chain-IIb; MyHC IIx, myosin heavy chain IIx; CaMK2B, calcium/calmodulin-dependent protein kinase II B; AdipoR1, adiponectin receptor 1; AMPKα, AMP-activated protein kinase α; SIRT1, sirtuin 1; PGC-1α, peroxisome proliferator-activated receptor γ coactivator 1 α; MEF2C, myocyte enhancer factor 2 C; GLUT4, glucose transporter type 4; Pdk4, pyruvate dehydrogenase kinase isoform 4; Cs, citrate synthase; Acadm, acyl-CoA dehydrogenase medium-chain; Acox1, acyl-CoA oxidase 1; Cox4, cytochrome-c-oxidase subunit 4; Cycs, cytochrome c; ATP5a1, ATP synthase F1 subunit α. GAPDH, reduced glyceraldehyde-phosphate dehydrogenase. |
MyHC-I
|
5′-GAAGGTGGGCAACGAA-3′ |
5′-CCAGGACCCCTATGAAGTA-3′ |
NM_213855.2 |
MyHC-IIa
|
5′-GATGGAGATCGACGACCTTGCT-3′ |
5′-CTGCTGCTCTTCCTCCTTGGAT-3′ |
NM_214136.1 |
MyHC-IIx
|
5′-CGCCAAGCTACTGAGGCAATAA-3′ |
5′-GTTCCACCATGGCCAGTTGTTC-3′ |
NM_001123141.1 |
MyHC-IIb
|
5′-TTGACTGGGCTGCCATCAAT-3′ |
5′-GCCTCAATGCGCTCCTTTTC-3′ |
NM_001104951.2 |
CAMK2B
|
5′-GACCTCAAGCCCGAGAACCT-3′ |
5′-TGCCAGCGAACCCAAACCAT-3′ |
XM_021078650.1 |
AdipoR1
|
5′-CACCAACCCACCCAAAG-3′ |
5′-TGACCCTCCAGCGACC-3′ |
NM_001007193.1 |
AMPKα
|
5′-AACATGGACGGGTTGAAGAG-3′ |
5′-CGCAGAAACTCACCATCTGA-3′ |
NM_214266.1 |
SIRT1
|
5′-ACTCTCCCTCTTTTAGACCAAGC-3′ |
5′-AAACCTGGACTCTCCATCGG-3′ |
NM_001145750.2 |
MEF2C
|
5′-CAGGTGGTCTGATGGGTG-3′ |
5′-CTGGTGGAATAAGAACTCG-3′ |
NM_001044540.1 |
PGC1-α
|
5′-CCCGAAACAGTAGCAGAGACAAG-3′ |
5′-CTGGGGTCAGAGGAAGAGATAAAG-3′ |
NM_213963.2 |
GLUT4
|
5′-CTTCTCCGCTGTGCTC-3′ |
5′-GCCCAGAGGGTAGTGAG-3′ |
NM_001128433.1 |
Pdk4
|
5′-TCAGACAGAGGAGGTGGT-3′ |
5′-TCCTGGCAAAGAGTAGAG-3′ |
NM_001159306 |
Cs
|
5′-TAAGGAGCAAGCCAGAA-3′ |
5′-ACTGTAGCCTCGGAAACG-3′ |
XM_021091143.1 |
Acadm
|
5′-GGCAGCGATGTTTAGG-3′ |
5′-GCAGCAACTGGGATTA-3′ |
NM_214039 |
Acox1
|
5′-TGCCTTCATTGTCCCTAT-3′ |
5′-GGTAGCCATTATCCATCTCA-3′ |
NM_001101028.1 |
Cox4
|
5′-GTTCTTCATCGGCTTCACC-3′ |
5′-CTTCATGTCCAGCATCCTCT-3′ |
XM_021093705.1 |
Cycs |
5′-ATGGGTGATGTTGAGCAG-3′ |
5′-GGTGATGCCTTTGTTCT-3′ |
NM_001129970.1 |
ATP5a1
|
5′-ATGCCCTTGGTAACGC-3′ |
5′-TGTCGGTCGCCAATAA-3′ |
NM_001185142 |
GAPDH
|
5′-TCGGAGTGAACGGATTT-3′ |
5′-CCGCCTTGACTGTGCC-3′ |
NM_001206359.1 |
2.7 Western blot analysis
The total protein extracted from the LD muscle was used to measure myosin heavy chain I (MyHC-I) (M8421, Sigma), myosin heavy chain IIa (MyHC-IIa) (PA5-120340, Thermo Scientific), myosin heavy chain IIx (MyHC-IIx) (25182-1-AP, Proteintech), myosin heavy chain IIb (MyHC-IIb) (14-6503-82, Thermo Scientific), calcium/calmodulin-dependent protein kinase II B CaMK2B (11533-1-AP, Proteintech), phospho-AMP-activated protein kinase α (p-AMPKα) (2532S, CST), sirtuin 1(SIRT1) (13161-1-AP, Proteintech), peroxisome proliferator-activated receptor γ coactivator 1 α (PGC-1α) (66369-1-Ig, Proteintech), adiponectin receptor 1 (AdipoR1) (14361-1-AP, Proteintech) and myocyte enhancer factor 2 C (MEF2C) (10056-1-AP, Proteintech). Each sample contained 50 μg of protein, which was electrophoresed on sodium dodecyl sulfate–polyacrylamide gel using the western blot method along with a pre-stained protein ladder (Thermo Fisher, Rockford, Illinois). Proteins were therefore electro-transferred onto polyvinylidene fluoride membranes and blocked with 5% (w/v) bovine serum albumin for 1 h at room temperature, then incubated with the appropriate primary antibodies overnight at 4 °C. Primary antibodies were diluted to 1
:
1000 (MyHC-IIx, CaMK2B, p-AMPKα, SIRT1), 1
:
5000 (MyHC-I, PGC-1α) and 1
:
500 (MyHC-IIa), 1 μg ml−1 (MyHC-IIb). Following three washes, the membranes were incubated with HRP-labeled secondary antibodies (goat anti-mouse IgG/HRP goat anti-rabbit IgG, AWS0002, Abiowell) diluted to 1
:
5000. GAPDH (10494-1-AP, Proteintech) diluted to 1
:
5000 was used as an internal control.
2.8 Liquid chromatography–tandem mass spectrometry (LC-MS/MS) analysis
The LD muscle tissue samples were removed from the ultra-low temperature refrigerator at −80 °C and thawed. 25 mg samples were weighed and 500 μL of pre-cooled extractant, methanol
:
acetonitrile
:
water = 2
:
2
:
1, containing an isotopically labeled internal standard mixture, was added. Pre-chilled steel beads were added for homogenization at 35 Hz for 4 min and then sonicated in an ice-water bath for 5 min. The homogenization and sonication cycles were repeated three times. The beads were removed and allowed to stand for 1 h at −40 °C before centrifugation at 12
000 rpm for 15 min at 4 °C. The extracted supernatant was taken into the injection vial liner and used for LC-MS/MS analysis. Chromatographic column: UPLC BEH Amide column (2.1 mm × 100 mm, 1.7 μm); mass spectrometry equipment: Q Exactive HFX mass spectrometer (Orbitrap MS, Thermo). Mobile phase A: ultrapure water (containing 25 mmol L−1 ammonium acetate and 25 mmol L−1 ammonium hydroxide) (pH = 9.75); mobile phase B: acetonitrile. The autosampler temperature of the equipment was 4 °C and the injection volume was 2 μL. All data were analyzed based on the internal database of Shanghai Biotree Technology Company.
2.8 Statistical analyses
All data were expressed as mean ± standard error of the mean (SEM). Furthermore, SPPS 26.0 software (IBM Corp., USA) was used for one-way and two-way analysis of variance (ANOVA) with Tukey's test. P < 0.05 was considered statistically significant.
The raw metabolomics data were converted to mzXML format using ProteoWizard, processed using a proprietary R program package (kernel XCMS), and matched with a self-built secondary mass spectrometry database for substance annotation, with the algorithm scoring cutoff value set to 0.3. The data were then imported into SIMCA 14.1 software (MKS Data Analytics Solutions, Umea, Sweden) for multivariate statistical analysis and differential metabolite screening.
3. Results
3.1 Growth performance and serum biochemistry of pigs
As shown in Fig. 1, we observed that the addition of both 0.5% Leu (A-Leu) and 3.5% FO has no effect on the final body weight, average daily gain, average daily feed intake, carcass weight and liver index, just that FO decreased abdominal fat (P < 0.05). The interaction between A-Leu and FO was not observed. As shown in Fig. 2A, A-Leu reduced GLU (P < 0.01). FO reduced TG (P < 0.01) and LDL (P < 0.01). However, there was no interaction between A-Leu and FO. Afterwards, we tested serum cytokines. As shown in Fig. 2B, both A-Leu and FO had no effects on insulin, IGF1, MSTN, chemerin, FGF 21 and leptin, but FO significantly increased adiponectin (P = 0.01), although there was no interaction between A-Leu and FO for all serum cytokines.
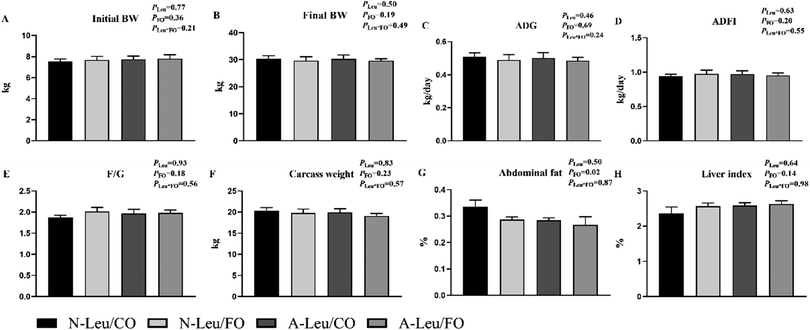 |
| Fig. 1 Effects of Leu and FO on growth performance and carcass characteristics of the pigs. (A) Initial BW, body weight at the start of the trial; (B) final BW, body weight at the end of the trial; (C) ADG, average daily gain; (D) ADFI, average daily feed intake; (E) F/G, feed/gain; (F) carcass weight; (G) abdominal fat;(H) liver index, liver weight/body weight. PLeu, significance of the main effect of A-Leu by two-way ANOVA; PFO, significance of the main effect of FO by two-way ANOVA; PLeu*FO, significance of the interaction effect of A-Leu and FO by two-way ANOVA. Data are expressed as mean ± SE. | |
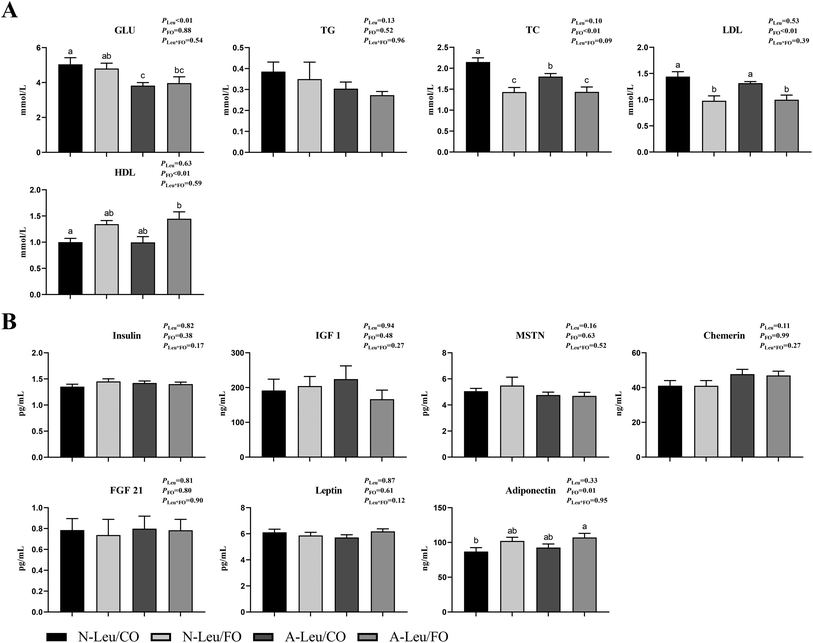 |
| Fig. 2 Effects of A-Leu and FO on serum biochemical indicators and serum cytokines of the pigs. (A) Effects of A-Leu and FO on the levels of glucose (GLU), total triglyceride (TG), total cholesterol (TC), low-density lipoprotein (LDL) and high-density lipoprotein (HDL). (B) Effects of A-Leu and FO on levels of insulin, insulin-like growth factor 1 (IGF 1), myostatin (MSTN), chemerin, fibroblast growth factors 21 (FGF 21), leptin and adiponectin. PLeu, significance of the main effect of A-Leu by two-way ANOVA; PFO, significance of the main effect of FO by two-way ANOVA; PLeu*FO, significance of the interaction effect of A-Leu and FO by two-way ANOVA. Data are expressed as the mean ± SE. Values with different superscript letters are significantly different by one-way ANOVA (P < 0.05). | |
3.2 Myofiber characteristics of the LD muscle of pigs
To investigate the effects of A-Leu and FO on skeletal myofiber types, we examined the myofiber composition of the LD muscle. As shown in Fig. 3, the results of muscle sections revealed that A-Leu increased the fiber number (P < 0.05), sarcomere length (P < 0.01) and slow-fiber proportion (P < 0.01), and decreased the fiber diameter (P < 0.01). FO increased the fiber diameter (P = 0.05), fiber number (P = 0.01), sarcomere length (P < 0.01) and slow-fiber proportion (P < 0.01). Overall, there was a significant interaction between A-Leu and FO for the sarcomere length (P < 0.01) and slow-fiber proportion (P < 0.01).
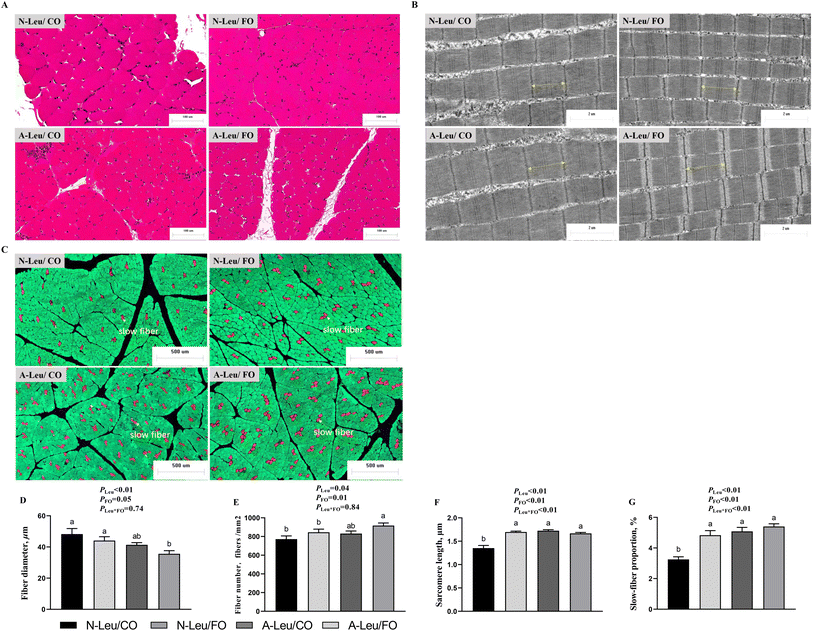 |
| Fig. 3 Effects of A-Leu and FO on fiber characteristics of LD muscle of pigs. (A) Hematoxylin–eosin staining for muscle. (B) Transmission electron microscopy image of the sarcomere of muscle. (C) Immunofluorescence staining for slow-fibers (red), fast-fibers (green) and nuclei (blue). (D) Effects of A-Leu and FO on the fiber diameter. (E) Effects of A-Leu and FO on the fiber number. (F) Effects of A-Leu and FO on the sarcomere length. (G) Effects of A-Leu and FO on slow-fiber proportion. PLeu, significance of the main effect of A-Leu by two-way ANOVA; PFO, significance of the main effect of FO by two-way ANOVA; PLeu*FO, significance of the interaction effect of A-Leu and FO by two-way ANOVA. Data are expressed as the mean ± SE. Values with different superscript letters are significantly different by one-way ANOVA (P < 0.05). | |
3.3 MyHC mRNA and protein expression levels of the LD muscle
We measured the relative mRNA expression levels of the myosin heavy chain (MyHC) of the LD muscle. As shown in Fig. 4A, A-Leu increased the relative mRNA expression levels of MyHC-I (P < 0.01) and MyHC-IIa (P < 0.01), but decreased the relative mRNA expression levels of MyHC-IIx (P < 0.05). Meanwhile, FO also increased the relative mRNA expression levels of MyHC-I (P < 0.01) and MyHC-IIa (P < 0.05). Interestingly, there was no significant interaction between A-Leu and FO, but the A-Leu/FO group increased the relative mRNA expression levels of MyHC-I and MyHC-IIa and decreased the relative mRNA expression levels of MyHC-IIb compared to the other three groups. Similarly, as shown in Fig. 4B, A-Leu increased the relative protein expression levels of MyHC-I (P < 0.01) and MyHC-IIa (P < 0.01) and decreased the relative protein expression levels of MyHC-IIx (P < 0.01) and MyHC-IIb (P < 0.05). FO also increased the relative mRNA expression levels of MyHC-I (P < 0.05) and MyHC-IIa (P < 0.01) and decreased the relative protein expression levels of MyHC-IIb (P < 0.05). There was also a significant interaction between A-Leu and FO for MyHC-IIx (P < 0.01).
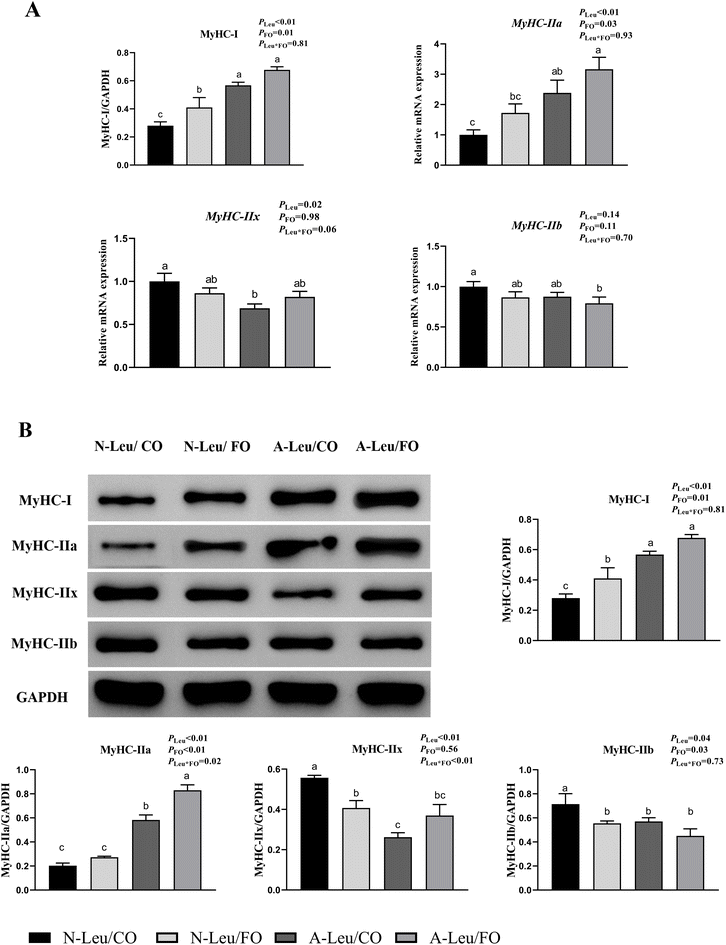 |
| Fig. 4 Effects of A-Leu and FO on the relative mRNA expression levels of myosin heavy chain (MyHC) of LD muscle of pigs. (A) Effects of A-Leu and FO on relative mRNA expression levels of MyHC-I, MyHC-IIa, MyHC-IIx, and MyHC-IIb. (B) Effects of A-Leu and FO on relative protein levels of MyHC-I, MyHC-IIa, MyHC-IIx, and MyHC-IIb, determined by western blot analysis. PLeu, significance of the main effect of A-Leu by two-way ANOVA; PFO, significance of the main effect of FO by two-way ANOVA; PLeu*FO, significance of the interaction effect of A-Leu and FO by two-way ANOVA. Data are expressed as the mean ± SE. Values with different superscript letters are significantly different by one-way ANOVA (P < 0.05). | |
3.4 Muscle chemistry composition of the LD muscle
We determined the content of free amino acids in the LD muscle. As shown in Table S2,† A-Leu increased Leu (P < 0.05), Thr (P < 0.05), Met (P < 0.05), His (P < 0.05), and Tyr (P < 0.05). FO had no effect on amino acids. Neither A-Leu nor FO had a significant effect on total amino acids, total essential amino acids and total non-essential amino acids. There was a significant interaction between A-Leu and FO for Thr (P < 0.01) and His (P < 0.05). Next, we measured the glycolysis-related substrates and enzyme activities in the LD muscle and calculated the glycolytic potential. As shown in Fig. 5, A-Leu decreased glucose (P < 0.01), lactate (P < 0.01), glycogen (P < 0.05), phosphocreatine (P < 0.01), and glycolytic potential (P < 0.05), while FO decreased glucose (P < 0.01). There was an interaction between the supplementation of A-Leu and FO for lactate (P < 0.01) and glycogen (P < 0.05). Taken together, these results indicate that Leu and FO lead to the myofiber type transition from glycolytic to oxidative in the muscles.
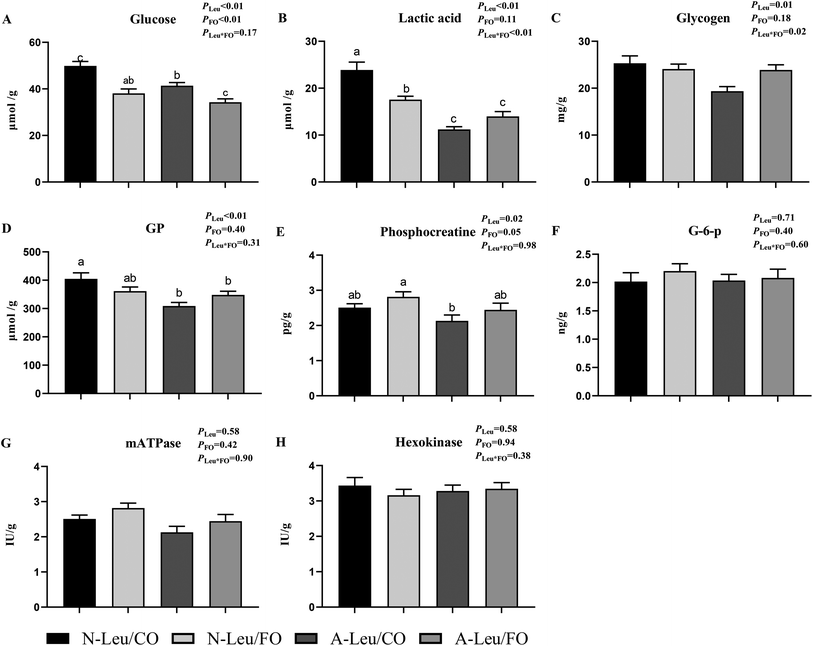 |
| Fig. 5 Effects of A-Leu and FO on glycolysis potential and enzyme activities of LD muscle of the pigs. (A–H) Effects of A-Leu and FO on contents of glucose, lactic acid, glycogen, glycolytic potential (GP) phosphocreatine (G-6-p), glucose 6-phosphate (mATPase) and hexokinase. Glycolytic potential = lactate content + 2 (glycogen content + glucose content + glucose-6-phosphate content). PLeu, significance of the main effect of A-Leu by two-way ANOVA; PFO, significance of the main effect of FO by two-way ANOVA; PLeu*FO, significance of the interaction effect of A-Leu and FO by two-way ANOVA. Data are expressed as the mean ± SE. Values with different superscript letters are significantly different by one-way ANOVA (P < 0.05). | |
3.5 Mitochondrial biogenesis of the LD muscle
We further measured the relative mRNA expression levels of genes related to glucose metabolism. As shown in Fig. 6, A-Leu increased the relative mRNA expression levels of GLUT4 (P < 0.01), Pdk4 (P < 0.01), Cs (P < 0.01), Cox4 (P < 0.01), Cycs (P < 0.01) and ATP5a1 (P < 0.01). FO increased the relative mRNA expression levels of GLUT4 (P < 0.01), Acadm (P < 0.05), Acox1 (P < 0.01), Cycs (P < 0.05) and ATP5a1 (P < 0.01). There was no significant interaction between A-Leu and FO, but the A-Leu/FO group significantly increased the relative mRNA expression levels for GLUT4 (P < 0.05) and ATP5a1 (P < 0.05) compared to the other three groups.
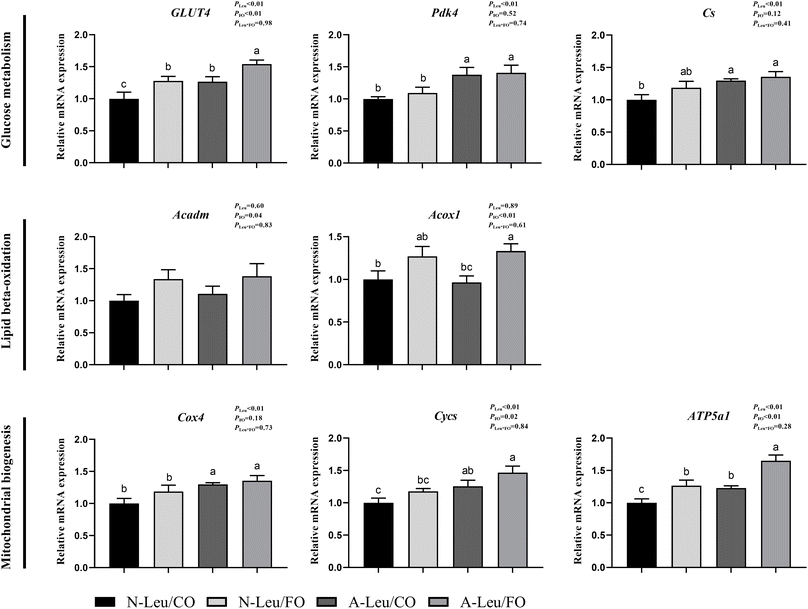 |
| Fig. 6 Effects of A-Leu and FO on the relative mRNA expression levels of genes related to glucose metabolism, lipid metabolism and mitochondrial biogenesis of LD muscle of pigs. (A) Effects of A-Leu and FO on relative mRNA expression levels of glucose transporter type 4 (GLUT4), pyruvate dehydrogenase kinase isoform 4 (PDK4), citrate synthase (Cs), acyl-CoA dehydrogenase medium-chain (Acadm), acyl-CoA oxidase 1 (Acox1), cytochrome-c-oxidase subunit 4 (COX4), cytochrome c (Cycs) and ATP synthase F1 subunit α (ATP5a1). PLeu, significance of the main effect of A-Leu by two-way ANOVA; PFO, significance of the main effect of FO by two-way ANOVA; PLeu*FO, significance of the interaction effect of A-Leu and FO by two-way ANOVA. Data are expressed as mean ± SE. Values with different superscript letters are significantly different by one-way ANOVA (P < 0.05). | |
3.6 Activation of the CaMKII and adiponectin signaling pathway
There were significant alterations in the ultrastructure, MyHC mRNA expression levels and mitochondrial biogenesis in the muscle fiber. To further investigate the possible pathways of myofiber transformation, the relative mRNA and protein expression levels of the key genes were determined. As shown in Fig. 7A, A-Leu significantly increased the relative mRNA expression levels of CaMK2B (P < 0.01), AMPKα (P < 0.01), SIRT1 (P < 0.01), PGC-1α (P < 0.01) and MEF2C (P < 0.01). However, FO increased the relative mRNA expression levels of CaMK2B (P < 0.01), SIRT1 (P < 0.05), AdipoR1 (P < 0.01) and MEF2C (P < 0.01). There was no significant interaction between A-Leu and FO, but the A-Leu/FO group significantly increased the relative mRNA expression levels of CaMK2B (P < 0.05) and MEF2C (P < 0.05) compared to the other three groups. Similarly, as shown in Fig. 7B, A-Leu significantly increased the relative protein expression levels of CaMK2B (P < 0.01), p-AMPKα (P < 0.01), SIRT1 (P < 0.01), PGC-1α (P < 0.01) and MEF2C (P < 0.01). FO increased the relative protein expression levels of CaMK2B (P < 0.01), p-AMPKα (P < 0.01), SIRT1 (P < 0.01), PGC-1α (P < 0.01), AdipoR1 (P < 0.01) and MEF2C (P < 0.01), but there was an interaction between A-Leu and FO for SIRT1 (P < 0.01). These situations were basically consistent with the qPCR results. Taken together, these results indicate that Leu activated the CaMKII signaling pathway to up-regulate MEF2, and FO further activated the adiponectin signaling pathway and enhanced the CaMKII pathway.
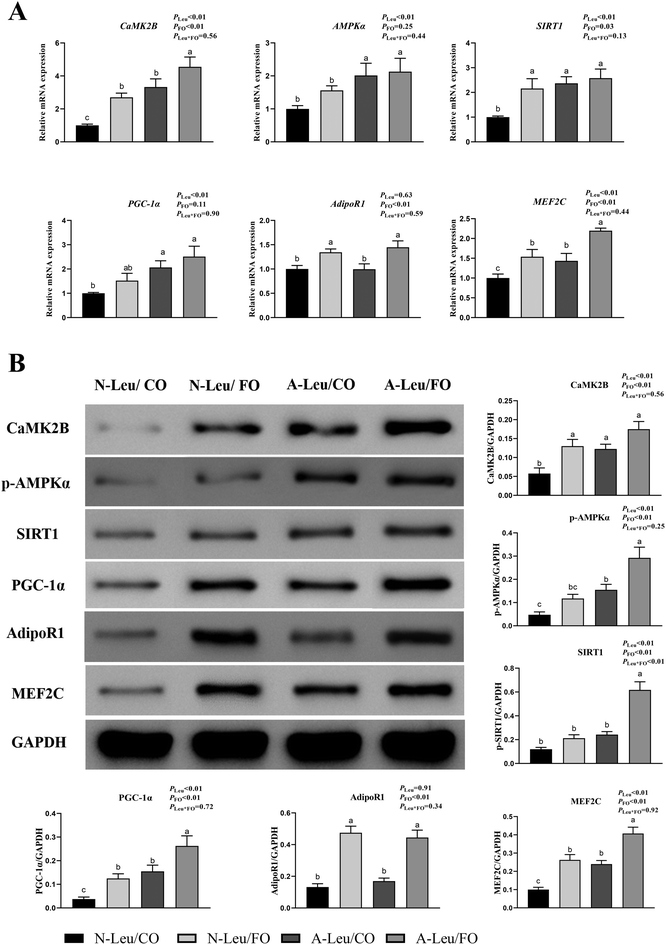 |
| Fig. 7 Effects of A-Leu and FO on the activation of the CaMKII pathway and adiponectin pathway of the LD muscle of pigs. (A) Effects of A-Leu and FO on relative mRNA expression levels of calcium/calmodulin-dependent protein kinase II B (CaMK2B), phospho-AMP-activated protein kinase (p-AMPK), sirtuin 1 (SIRT1), peroxisome proliferator-activated receptor γ coactivator 1 α (PGC-1α), adiponectin receptor 1 (AdipoR1), and myocyte enhancer factor 2 C (MEF2C). (B) Effects of A-Leu and FO on relative protein levels of CaMK2B, p-AMPK, SIRT1, PGC-1α, AdipoR1 and MEF2C were determined by western blot analysis. PLeu, significance of the main effect of A-Leu by two-way ANOVA; PFO, significance of the main effect of FO by two-way ANOVA; PLeu*FO, significance of the interaction effect of A-Leu and FO by two-way ANOVA. Data are expressed as the mean ± SE. Values with different superscript letters are significantly different by one-way ANOVA (P < 0.05). | |
3.7 Muscle metabolomics
Whether A-Leu and FO are metabolites associated with skeletal myofiber type conversion was further determined. The UHPLC-MS/MS method detected a total of 870 metabolites in the longest back muscle of pigs by comparison with reliable databases. PCA analysis showed that muscle metabolite scatter plots for corn oil and FO were crossed between the two groups; however, muscle metabolite scatter plots for N-Leu and A-Leu were significantly different (Fig. S1A†). OPLS-DA analysis showed that different Leu levels and muscle metabolite patterns of different oils were significantly different (Fig. S1B†). By screening with VIP > 1 and t-test P-value < 0.05, a total of 117 differential metabolites of N-Leu and A-Leu were identified, and the heat map of metabolites was drawn (Fig. S1C†), among which the levels of 52 differential metabolites were diminished by high Leu and those of 65 differential metabolites were elevated. By screening with VIP > 1 and t-test P-value < 0.05, a total of 104 differential metabolites of the addition of 3.5% CO and FO were identified, and the heat map of metabolites was drawn (Fig. S1D†), among which 56 differential metabolites were diminished and 48 differential metabolites were elevated in FO. Metabolic pathway analysis showed that different levels of Leu affected amino sugar and nucleotide sugar metabolism, the pentose phosphate pathway and ubiquinone and biosynthesis of other terpenoid–quinones (Fig. 8A). As shown in Fig. 8B, different kinds of oils affected D-glutamine and D-glutamate metabolism and linoleic acid metabolism. We then related the pathways involved in these metabolic differentials to the mRNA expression profile and found that A-Leu significantly increased cyclic AMP (P < 0.01) and caffeine (P < 0.05). FO, on the other hand, significantly increased linoleic acid (P < 0.01), linoleylcarnitine (P < 0.01) and L-glutamine (P < 0.01).
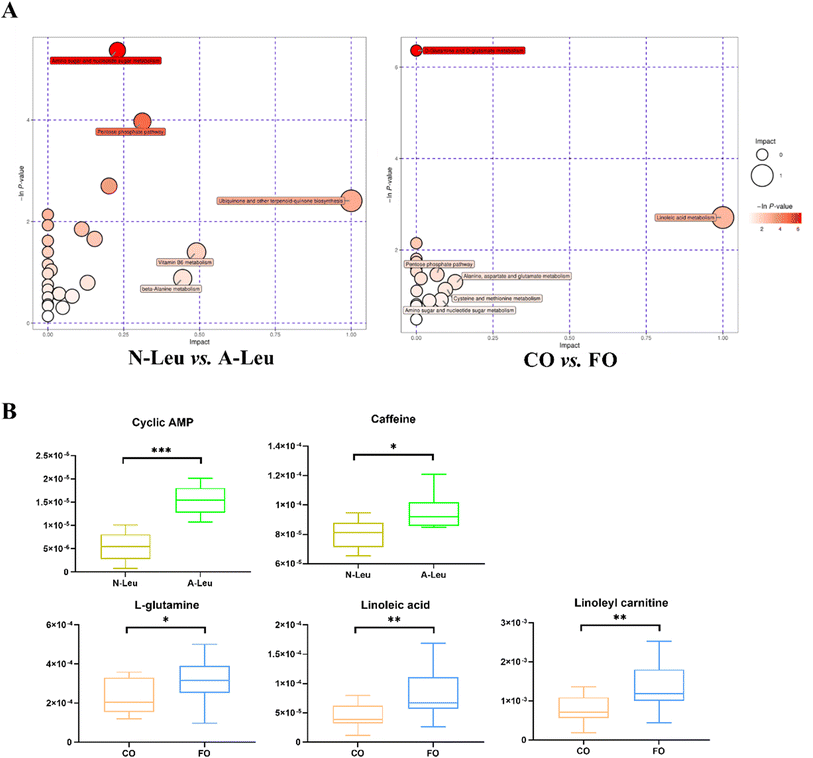 |
| Fig. 8 Effects of A-Leu and FO on metabolites of LD muscle of pigs. (A) Bubble plot showing pathway enrichment of various metabolites in N-Leu vs. A-Leu and CO vs. FO. (B) Differences of cyclic AMP, caffeine, L-glutamine, linoleic acid and linoleyl carnitine. Data are expressed as the mean ± SE. Differences between the two groups after the t-test are marked by * (P < 0.05), **(P < 0.01) and *** (P < 0.001). | |
4. Discussion
In this study, we conducted a comprehensive evaluation of the efficient effects of Leu and FO on myofiber type and muscle metabolism in piglets to determine if Leu and FO have favorable effects and interactions during the early development of pigs. Our study found that A-Leu and FO increase the proportion of slow myofibers and upregulate the expression of MyHC-I and MyHC-IIa, and A-Leu activates the CaMKII-AMPK/SIRT1-PGC-1α pathway and upregulates MEF2-triggered myofiber type transformation from glycolytic to oxidative. FO activates the adiponectin signaling pathway to further enhance the effects of Leu. We found that neither leucine nor fish oil altered the performance of piglets. However, Dang et al.19 found that fish oil from a 0.5% coated refined fish oil diet increased BW and ADG in finishing pigs.
Our previous studies reported that the supplementation of low protein diets with BACC, especially Leu, induced a glycolytic to oxidative shift of the myofiber type in the meat of growing pigs.10,20 A previous study from our team also found that 8% levels of fresh and oxidized fish oil had a negative effect on carcass traits and muscle fiber characteristics in finishing pigs,21 so we chose a lower level of fish oil (3.5%) for this trial. The results of immunofluorescence staining of the LD muscle directly indicate that A-Leu and FO substantially increased the proportion of slow muscle fibers and there was a mutually reinforcing interaction between Leu and FO. Furthermore, skeletal myofiber size was adversely correlated with the oxidative capacity in pork.22 A-Leu and FO reduced the muscle fiber diameter but increased the fiber number and sarcomere length, indirectly indicating their effect on muscle fiber function and types. At the molecular level, the present-day characterization of myofiber types is primarily based on the immunoreactivity to different MyHC subtype-specific antibodies, and MyHC subtypes can be detailed into four isoforms, I, IIa, IIb and IIx.23 MyHC type I muscle fibers are highly oxidative and have the highest mitochondrial content. The complete opposite is true for type IIb fibers, which lack mitochondria and rely mainly on glycolysis as an energy source. The transformation of the four MyHC isoforms does not immediately leap from one extreme to the other, which basically follows an ordered sequence: IIb → IIx → IIa → I.24 In this study, A-Leu and FO increased MyHC-I, MyHC-IIa and MyHC-IIx and there was a decreasing trend in MyHC-IIb, suggesting that Leu and FO, especially Leu, could promote the transformation of myofiber type from glycolytic to oxidative and basically follow the foregoing sequence.
The difference in the metabolic patterns of oxidative and glycolytic muscle fibers would cause the muscle rich in oxidative fiber to require less glucose for the same amount of energy. In our results, A-Leu decreased glucose, lactate, glycogen, creatine phosphate, and GP in the skeletal muscle, while FO also decreased glucose, where both were present interactions in lactate and glycogen. Furthermore, both A-Leu and FO have been shown to improve mitochondrial biogenesis, characterized by increasing glucose metabolism in A-Leu and fatty acid beta-oxidation in FO. In the C2C12 cell experiment, Leu increased the oxidative capacity, carbohydrate oxidation efficiency, and mitochondrial biogenesis.25 Additionally, recent research has demonstrated that treatment with PUFAs elevates Sirt1 and PGC-1, two essential mitochondrial energy metabolism genes, in C2C12 cells.26 Thus, Leu may trigger alterations in metabolic patterns by altering the myofiber type, increasing the efficiency of glucose utilization and thus decreasing the levels of energy-supplying substances in muscle. FO, on the other hand, reduces TC, LDL and abdominal fat by improving the utilization of fatty acids.
Later, we focused on the expression of related genes and proteins based on the influence of A-Leu and FO on energy metabolism and adiponectin. Calmodulin (CaM) is a Ca2+ receptor protein that undergoes conformational changes when Ca2+ is bound, modifying Ca2+-CaM-dependent phosphatases and kinases, and Ca2+ signaling by kinases and phosphatases may be an essential mechanism for the regulation of skeletal muscle cell response.27,28 The downstream signals of CaMKII, AMPK and SIRT1 are cellular energy-sensing molecules with functional resemblance regarding actions in such diverse processes as cellular metabolism, inflammation and mitochondrial function.29 MEF2 is a conserved and critical transcription factor that activates gene expression and cellular transformation in muscle cells.30 PGC-1α cooperates with the MEF2 protein to activate transcription and can drive the development of slow myofibers.31 Our study found that Leu elevated the mRNA expression of CaMKII and its downstream genes AMPK/Sirt1/PGC-1α in muscle, elevated the mRNA expression of MEF2, and similarly enhanced the expression of GLUT4 and mitochondrial biogenesis-associated genes. MEF2-dependent GLUT4 gene expression is regulated by CaMKII activation as it triggers hyperacetylation of histones close to the MEF2 structural domain and promotes binding to cis-elements of MEF2.32 Previous studies have reported that Leu boosts the amount of slow muscle fibers and enhances mitochondrial activity via the SIRT1/AMPK signaling pathway in porcine primary skeletal muscle cells.33 It has also been proposed that different BACC ratios, with Leu being the most prominent, under low-protein diets may induce more oxidative myofiber type in growing pigs through the activation of the AMPK-SIRT1-PGC-1α axis.10 In addition, we also found that FO activated AdipoR1 of the adiponectin signaling pathway elevated the mRNA expression of MEF2 and similarly improved the expression of genes involved in fatty acid oxidation and mitochondrial respiration. The mechanism of action of adiponectin is through the stimulation of Ca2+ influx by AdipoR1, which causes activation of CaMKII signaling, which also activates the AMPK-SIRT1-PGC-1α pathway, thereby enhancing fatty acid oxidation and mitochondrial biogenesis of skeletal muscle.34 Huang et al. showed that the activation of the AdipoR1-AMPK-PGC-1α pathway increased the percentage of oxidized muscle fibers in pigs and porcine primary muscle cells.35 The supplementation of feed with grape seed proanthocyanidin extract (GSPE) increased the percentage of slow fibers in finishing pigs and was accompanied by an increase in n-3 PUFA and the ratio of total PUFA in the muscle.36 In contrast, FO is rich in omega-3 PUFA, which suggests that it may be PUFA that causes the activation of AdipoR1. However, this does not suggest that it is PUFA that acts directly in the muscle, but most likely one of the metabolites of PUFA.
Thus, we further explored the differences in metabolites within the muscle. Our study found that A-Leu increased cyclic AMP and caffeine in the skeletal muscle. In skeletal muscle cells, caffeine activates the release of Ca2+ from the sarcoplasmic reticulum via ryanodine receptor type 1 (RyR1).37 Also, caffeine stimulates glucose uptake and mitochondrial biogenesis by activating the cyclic AMP/protein kinase A (PKA) pathway, and is responsible for increased expression of myoglobin in skeletal muscle cells.38 This may be one of the reasons why CaMKII signaling is activated. Studies have shown that glutamine promotes satellite cell and muscle regeneration,39 FO increased glutamine but the relationship with myofiber conversion remains to be investigated. In other studies, increasing linoleic acid enhanced mitochondrial biosynthesis40 and skeletal muscle proliferation and differentiation.41 And we also found that FO increased linoleic acid and linoleoyl carnitine, so this could be the effective factor through which FO works within the muscle, but further studies are needed.
The foregoing results demonstrate that Leu increases MEF2 and mitochondrial biogenesis through the activation of the CaMKII-AMPK/Sirt1-PGC-1α pathway, inducing a shift in the skeletal myofiber type from glycolytic to oxidative in the order IIb → IIx → IIa → I in pigs. FO further enhances CaMKII and fatty acid oxidation via AdipoR1, enhances mitochondrial biogenesis, and synergizes with Leu to promote myofiber transformation.
5. Conclusion
In conclusion, the current study demonstrated that the addition of 0.5% Leu and 3.5% FO to the feed of piglets reduced the glycolytic potential in the LD muscle and cooperatively regulated the transformation of myofiber type from glycolytic to oxidative. It is hypothesized that there is an interaction between amino acids and polyunsaturated fatty acids, which may be achieved by enhancing mitochondrial biogenesis through the CaMKII signaling pathway. Subsequent validation will be performed using the in vitro cell culture model.
Abbreviations
AMPKα | AMP-activated protein kinase α |
ADG | Average daily gain |
ADFI | Average daily feed intake |
Acadm | Acyl-CoA dehydrogenase medium-chain |
Acox1 | Acyl-CoA oxidase 1 |
AdipoR1 | Adiponectin receptor 1 |
ATP5a1 | ATP synthase F1 subunit α |
BW | Body weight |
BACC | Branched-chain amino acids |
CO | Corn oil |
CaM | Calmodulin |
CaMKII | Calcium/calmodulin-dependent protein kinase II |
COX4 | Cytochrome-c-oxidase subunit 4 |
Cs | Citrate synthase |
Cycs | Cytochrome c |
FO | Fish oil |
FGF 21 | Fibroblast growth factors 21 |
GAPDH | Reduced glyceraldehyde-phosphate dehydrogenase |
GLU | Glucose |
GLUT4 | Glucose transporter type 4 |
GP | Glycolytic potential |
HDL | High-density lipoprotein-cholesterol |
IGF1 | Insulin-like growth factor 1 |
Leu | Leucine |
LD |
Longissimus dorsi
|
LDL | Low-density lipoprotein-cholesterol |
MEF2C | Myocyte enhancer factor-2C |
MSTN | Myostatin |
MyHC | Myosin heavy chain |
OPLS-DA | Orthogonal partial least-squares discrimination |
PCA | Principal component analysis |
PDK4 | Pyruvate dehydrogenase kinase isoform 4 |
PGC-1α | Peroxisome proliferator-activated receptor γ coactivator 1 α |
PUFA | Polyunsaturated fatty acids |
SIRT1 | Sirtuin 1 |
TC | Total cholesterol |
TG | Total triglycerides |
VIP | Variable important in projection |
Conflicts of interest
All authors declared that they have no known competing financial interests or personal relationships that could have appeared to influence the work reported in this paper.
Acknowledgements
This work was supported by the National Natural Science Foundation of China (31972582), the Distinguished Young Youths of Hunan Province (2020JJ2030), the Science and Technology Innovation Program of Hunan Province (2021RC4039), the Key R & D Program of Hunan Province (2022NK2026), the Youth Innovation Promotion Association CAS (Y202079), the China Agriculture Research System of MOF and MARA (CARS-35).
References
- A. H. Karlsson, R. E. Klont and X. Fernandez, Skeletal muscle fibres as factors for pork quality, Livest. Prod. Sci., 1999, 60(2), 255–269 CrossRef
.
- S. Schiaffino and C. Reggiani, Fiber types in mammalian skeletal muscles, Physiol. Rev., 2011, 91(4), 1447–1531 CrossRef CAS PubMed
.
- K.-C. Chang and K. Fernandes, Developmental expression and 5′ cloning of the porcine 2x and 2b myosin heavy chain genes, DNA Cell Biol., 1997, 16(12), 1429–1437 CrossRef CAS PubMed
.
- Y. M. Choi, K. W. Nam, J. H. Choe, Y. C. Ryu, M. P. Wick, K. Lee and B. C. Kim, Growth, carcass, fiber type, and meat quality characteristics in Large White pigs with different live weights, Livest. Sci., 2013, 155(1), 123–129 CrossRef
.
- D. A. Columbus, M. L. Fiorotto and T. A. Davis, Leucine is a major regulator of muscle protein synthesis in neonates, Amino Acids, 2015, 47(2), 259–270 CrossRef CAS PubMed
.
- M. J. Drummond, P. T. Reidy, L. M. Baird, B. K. Dalley and M. T. Howard, Leucine differentially regulates gene-specific translation in mouse skeletal muscle, J. Nutr., 2017, 147(9), 1616–1623 CAS
.
- R. Manjarín, C. Boutry-Regard, A. Suryawan, A. Canovas, B. D. Piccolo, M. Maj, M. Abo-Ismail, H. V. Nguyen, M. L. Fiorotto and T. A. Davis, Intermittent leucine pulses during continuous feeding alters novel components involved in skeletal muscle growth of neonatal pigs, Amino Acids, 2020, 52(9), 1319–1335 CrossRef PubMed
.
- C.-X. Wang, F. Chen, W.-F. Zhang, S.-H. Zhang, K. Shi, H.-Q. Song, Y.-J. Wang, S. W. Kim and W.-T. Guan, Leucine promotes the growth of fetal pigs by increasing protein synthesis through the mTOR signaling pathway in longissimus dorsi muscle at late gestation, J. Agric. Food Chem., 2018, 66(15), 3840–3849 CrossRef CAS PubMed
.
- L. Zhang, F. Li, Q. Guo, Y. Duan, W. Wang, Y. Yang, Y. Yin, S. Gong, M. Han and Y. Yin, Different proportions of branched-chain amino acids modulate lipid metabolism in a finishing pig model, J. Agric. Food Chem., 2021, 69(25), 7037–7048 CrossRef CAS PubMed
.
- Y. Duan, F. Li, W. Wang, Q. Guo, C. Wen and Y. Yin, Alteration of muscle fiber characteristics and the AMPK-SIRT1-PGC-1α axis in skeletal muscle of growing pigs fed low-protein diets with varying branched-chain amino acid ratios, Oncotarget, 2017, 8(63), 107011 CrossRef PubMed
.
- Y. Yin, Y. Liu, G. Duan, M. Han, S. Gong, Z. Yang, Y. Duan, Q. Guo, Q. Chen and F. Li, The effect of dietary leucine supplementation on antioxidant capacity and meat quality of finishing pigs under heat stress, Antioxidants, 2022, 11(7), 1373 CrossRef CAS PubMed
.
- A. V. Lee, L. You, S.-Y. Oh, Z. Li, A. Code, C. Zhu, R. E. Fisher-Heffernan, T. R. Regnault, C. F. De Lange and L.-A. Huber, Health benefits of supplementing nursery pig diets with microalgae or fish oil, Animals, 2019, 9(3), 80 CrossRef PubMed
.
- Q. Nong, L. Wang, Y. Zhou, Y. Sun, W. Chen, J. Xie, X. Zhu and T. Shan, Low Dietary n-6/n-3 PUFA ratio regulates meat quality, reduces triglyceride content, and improves fatty acid composition of meat in heigai pigs, Animals, 2020, 10(9), 1543 CrossRef PubMed
.
- G. I. Smith, S. Julliand, D. N. Reeds, D. R. Sinacore, S. Klein and B. Mittendorfer, Fish oil-derived n-3 PUFA therapy increases muscle mass and function in healthy older adults, Am. J. Clin. Nutr., 2015, 102(1), 115–122 CrossRef CAS PubMed
.
- R. A. Murphy, M. Mourtzakis, Q. S. Chu, V. E. Baracos, T. Reiman and V. C. Mazurak, Nutritional intervention with fish oil provides a benefit over standard of care for weight and skeletal muscle mass in patients with nonsmall cell lung cancer receiving chemotherapy, Cancer, 2011, 117(8), 1775–1782 CrossRef CAS PubMed
.
- B. Picard, C. Berri, L. Lefaucheur, C. Molette, T. Sayd and C. Terlouw, Skeletal muscle proteomics in livestock production, Briefings Funct. Genomics, 2010, 9(3), 259–278 CrossRef CAS PubMed
.
- B. Picard, L. Lefaucheur, C. Berri and M. J. Duclos, Muscle fibre ontogenesis in farm animal species, Reprod., Nutr., Dev., 2002, 42(5), 415–431 CrossRef PubMed
.
-
N. R. Council, Nutrient requirements of swine, 2012 Search PubMed
.
- D. X. Dang and I. H. Kim, Coated refined fish oil supplementation improves growth performance and meat quality in finishing pigs, Livest. Sci., 2022, 265, 105099 CrossRef
.
- L. Zhang, F. Li, Q. Guo, Y. Duan, W. Wang, Y. Yang, Y. Yin, S. Gong, M. Han and Y. Yin, Balanced branched-chain amino acids modulate meat quality by adjusting muscle fiber type conversion and intramuscular fat deposition in finishing pigs, J. Sci. Food Agric., 2022, 102(9), 3796–3807 CrossRef CAS PubMed
.
- Q. Guo, L. Zhang, Y. Duan, W. Wang, R. Huang and F. Li, Changes in carcass traits, meat quality, muscle fiber characteristics, and liver function of finishing pigs fed high level of fish oil, Can. J. Anim. Sci., 2021, 101(2), 342–352 CrossRef CAS
.
- T. Van Wessel, A. De Haan, W. Van Der Laarse and R. Jaspers, The muscle fiber type–fiber size paradox: Hypertrophy or oxidative metabolism?, Eur. J. Appl. Physiol., 2010, 110(4), 665–694 CrossRef CAS PubMed
.
- G.-D. Kim, Y.-C. Ryu, C. Jo, J.-G. Lee, H.-S. Yang, J.-Y. Jeong and S.-T. Joo, The characteristics of myosin heavy chain-based fiber types in porcine longissimus dorsi muscle, Meat Sci., 2014, 96(2), 712–718 CrossRef CAS PubMed
.
- D. Pette and R. S. Staron, Mammalian skeletal muscle fiber type transitions, Int. Rev. Cytol., 1997, 170, 143–223 CAS
.
- R. A. Vaughan, R. Garcia-Smith, N. P. Gannon, M. Bisoffi, K. A. Trujillo and C. A. Conn, Leucine treatment enhances oxidative capacity through complete carbohydrate oxidation and increased mitochondrial density in skeletal muscle cells, Amino Acids, 2013, 45(4), 901–911 CrossRef CAS PubMed
.
- M. A. Risha, P. Siengdee, D. Dannenberger, K. Wimmers and S. Ponsuksili, PUFA treatment affects C2C12 myocyte differentiation, myogenesis related genes and energy metabolism, Genes, 2021, 12(2), 192 CrossRef PubMed
.
- A. J. Rose, T. J. Alsted, J. B. Kobberø and E. A. Richter, Regulation and function of Ca2+-calmodulin-dependent protein kinase II of fast–twitch rat skeletal muscle, J. Physiol., 2007, 580(3), 993–1005 CrossRef CAS PubMed
.
- X. Mu, L. D. Brown, Y. Liu and M. F. Schneider, Roles of the calcineurin and CaMK signaling pathways in fast-to-slow fiber type transformation of cultured adult mouse skeletal muscle fibers, Physiol. Genomics, 2007, 30(3), 300–312 CrossRef CAS PubMed
.
- N. B. Ruderman, X. J. Xu, L. Nelson, J. M. Cacicedo, A. K. Saha, F. Lan and Y. Ido, AMPK and SIRT1: A long-standing partnership?, Am. J. Physiol.: Endocrinol. Metab., 2010, 4, E751–E760 CrossRef PubMed
.
- M. V. Taylor and S. M. Hughes, Mef2 and the skeletal muscle differentiation program, Semin. Cell Dev. Biol., 2017, 72, 33–44 CrossRef CAS PubMed
.
- J. Lin, H. Wu, P. T. Tarr, C.-Y. Zhang, Z. Wu, O. Boss, L. F. Michael, P. Puigserver, E. Isotani and E. N. Olson, Transcriptional co-activator PGC-1α drives the formation of slow-twitch muscle fibres, Nature, 2002, 418(6899), 797–801 CrossRef CAS PubMed
.
- E. O. Ojuka, V. Goyaram and J. A. Smith, The role of CaMKII in regulating GLUT4 expression in skeletal muscle, Am. J. Physiol.: Endocrinol. Metab., 2012, 303(3), E322–E331 CrossRef CAS PubMed
.
- X. Chen, L. Xiang, G. Jia, G. Liu, H. Zhao and Z. Huang, Leucine regulates slow-twitch muscle fibers expression and mitochondrial function by Sirt1/AMPK signaling in porcine skeletal muscle satellite cells, Anim. Sci. J., 2019, 90(2), 255–263 CrossRef CAS PubMed
.
- M. Iwabu, T. Yamauchi, M. Okada-Iwabu, K. Sato, T. Nakagawa, M. Funata, M. Yamaguchi, S. Namiki, R. Nakayama and M. Tabata, Adiponectin and AdipoR1 regulate PGC-1α and mitochondria by Ca2+ and AMPK/SIRT1, Nature, 2010, 464(7293), 1313–1319 CrossRef CAS PubMed
.
- Y. Huang, Q. Xia, Y. Cui, Q. Qu, Y. Wei and Q. Jiang, Resveratrol increase the proportion of oxidative muscle fiber through the AdipoR1-AMPK-PGC-1α pathway in pigs, J. Funct. Foods, 2020, 73, 104090 CrossRef CAS
.
- M. Xu, X. Chen, Z. Huang, D. Chen, M. Li, J. He, H. Chen, P. Zheng, J. Yu and Y. Luo, Effects of dietary grape seed proanthocyanidin extract supplementation on meat quality, muscle fiber characteristics and antioxidant capacity of finishing pigs, Food Chem., 2022, 367, 130781 CrossRef CAS PubMed
.
- V. R. Chirasani, D. A. Pasek and G. Meissner, Structural and functional interactions between the Ca2+-, ATP-, and caffeine-binding sites of skeletal muscle ryanodine receptor (RyR1), J. Biol. Chem., 2021, 297(3), 712–718 CrossRef PubMed
.
- T. Yokokawa, T. Hashimoto and N. Iwanaka, Caffeine increases myoglobin expression via the cyclic AMP pathway in L6 myotubes, Physiol. Rep., 2021, 9(9), e14869 CAS
.
- M. Shang, F. Cappellesso, R. Amorim, J. Serneels, F. Virga, G. Eelen, S. Carobbio, M. Y. Rincon, P. Maechler and K. De Bock, Macrophage-derived glutamine boosts satellite cells and muscle regeneration, Nature, 2020, 587(7835), 626–631 CrossRef CAS PubMed
.
- Y. Kim and Y. Park, Conjugated linoleic acid (CLA) stimulates mitochondrial biogenesis signaling by the upregulation of PPARγ coactivator 1α (PGC-1α) in C2C12 cells, Lipids, 2015, 50(4), 329–338 CrossRef CAS PubMed
.
- J.-H. Lee, H. Tachibana, Y. Morinaga, Y. Fujimura and K. Yamada, Modulation of proliferation and differentiation of C2C12 skeletal muscle cells by fatty acids, Life Sci., 2009, 84(13–14), 415–420 CrossRef CAS PubMed
.
|
This journal is © The Royal Society of Chemistry 2023 |