DOI:
10.1039/D2FO01637K
(Paper)
Food Funct., 2023,
14, 215-230
Fermented Angelica sinensis activates Nrf2 signaling and modulates the gut microbiota composition and metabolism to attenuate D-gal induced liver aging†
Received
13th June 2022
, Accepted 29th November 2022
First published on 30th November 2022
Abstract
Aging is an inevitable physiological process associated with an imbalance in the oxidative defense system. Angelica sinensis, a kind of traditional Chinese medicine (TCM), has anti-oxidant effects and has been considered as a potential supplement in anti-aging treatment. Nevertheless, it has the disadvantages of slow efficacy and long duration of treatment. Fermentation, as an efficient biotechnological approach, is beneficial for improving the nutritional capacity of the material. Fermented TCMs are considered to be more effective. In this study, fermented Angelica sinensis (FAS) and non-fermented Angelica sinensis (NFAS) were used to investigate changes in the chemical constituents. Furthermore, the improvement effect of FAS on D-galactose-induced aging in mice and the potential mechanisms were explored. The results revealed that FAS and NFAS had different constituents under the influence of fermentation, such as 3-phenyllactic acid, L-5-hydroxytryptophan, taxifolin and methyl gallate. These elevated constituents of FAS might help increase the ability of FAS to improve aging. The aging model was established by intraperitoneal injection of D-galactose (2.5 g kg−1 day−1) for 44 days, and FAS (3 g kg−1 day−1) was administered daily by oral gavage after 2 weeks of induction with D-galactose. FAS was observed to significantly ameliorate changes associated with liver aging, such as reduction of MDA, AGEs and 8-OHdG. The contents of pro-inflammatory cytokines containing TNF-α, IL-1β and IL-6 were significantly suppressed in the FAS group. In addition, FAS activated Nrf2 signaling better than NFAS, improved the expression of Nrf2, HO-1, NQO1, GCLC, GCLM and GSS, and further increased the activities of SOD, CAT and other antioxidant enzymes in the liver. Simultaneously, it had a certain repair effect on the liver tissues of mice. The intestinal microbiota analysis showed that FAS could regulate the microbiota imbalance caused by aging, increase the ratio of Firmicutes/Bacteroidetes by 95% and improve the relative abundance of beneficial bacteria related to Nrf2 signaling, such as Lactobacillus. Besides, fecal metabolite analysis identified uric acid as an evidential metabolite, suggesting that FAS participates in purine metabolism to improve aging. Therefore, the regulation of intestinal microbiota and metabolism may be one of the important mechanisms of FAS in alleviating hepatic oxidative stress via the gut–liver axis. The results of this study could provide information for the future development of postbiotic products that may have beneficial effects on the prevention or treatment of aging.
Introduction
Aging is a natural and inevitable process in the human life course, and can cause the degradation of body tissues and organs.1 In the past decades, it has been recognized that the aging process is associated with the dysfunction of the antioxidant defense system.2 When the antioxidant system function of the body declines, the activities of antioxidant enzymes such as superoxide dismutase (SOD), catalase (CAT) and glutathione peroxidase (GSH-Px) decrease continually. This process produces an excessive accumulation of free radicals that can trigger irreversible oxidative damage and lead to aging ultimately.3 Meanwhile, nuclear factor erythroid 2-related factor 2 (Nrf2) signaling as a cytoprotective mechanism is considered as a regulator of the oxidative system, activating the antioxidant defense system by strengthening oxidative stress resistance and apoptosis.4,5 Under oxidative stress, Nrf2 binds to DNA sequences of the antioxidant response element (ARE) in the nucleus and regulates the expression of a series of downstream antioxidant genes, such as heme oxygenase-1 (HO-1), NADP(H) quinone oxidoreductase 1 (NQO1), glutamate-cysteine ligase catalytic subunit (GCLC), glutamate-cysteine ligase regulatory subunit (GCLM) and glutathione synthetase (GSS).6,7 Thus, the activation of Nrf2 signaling is an underlying mechanism to delay the aging process. On the other hand, accumulating discoveries proved that the gut microbiota and metabolites dominate the pathogenesis of oxidant-related disorders such as Alzheimer's disease, Parkinson's disease and aging.8,9 Aging affects the host immunity–microbiota interaction in the gut, which promotes the release of pro-inflammatory factors such as interleukin-1β (IL-1β), interleukin-6 (IL-6), and tumor necrosis factor-α (TNF-α) and prevents the elimination of senescent cells.10,11 Therefore, the gut microbiota is also a potential target for developing anti-aging functional foods.
The medicinal and edible traditional Chinese medicines (TCMs) used for anti-aging, such as Angelica, Ginseng and Cistanche, have a long history in China. They are suitable for conditioning chronic diseases with few side effects and high safety.12–15 Meanwhile, they can act as prebiotics to ameliorate the intestinal microbiota disorder caused by aging.16,17 Nevertheless, the recalcitrance of plant cell wall and the absorption of macromolecular substances are still obstacles to the development of the clinical potential of these TCMs.18 Therefore, TCMs generally have the disadvantage of slow efficacy and long treatment time. In recent years, the application of TCMs by submerged fermentation of probiotics has become a hot topic that widens the boundaries of TCMs. It uses TCMs as nutrients in the growth process of microorganisms and metabolizes them to generate new active metabolites with toxicity-attenuating and synergistic effects, which greatly improves the active ingredients of TCMs.19–22 Fermented TCMs can also effectively regulate the gut microbiota and restore intestinal homeostasis, thereby improving its dysfunction and related pathological conditions.23–26 Several studies have reported that the polysaccharides and organic acids contained in Angelica sinensis (AS) have good free radical scavenging effects in vitro.27–31 Considering the complexity of AS components and the body's environment, it would be more meaningful to study the antioxidant properties and underlying mechanisms of non-fermented Angelica sinensis (NFAS)/fermented Angelica sinensis (FAS) in vivo. More importantly, the concomitant effects of FAS on the gut microbiota and related metabolites remain to be investigated.
In this study, we used Lactobacillus plantarum LZU-J-TSL6 and LZU-S-ZCJ isolated from the fermented food JiangShui and yak yogurt in northwest China to ferment AS and obtained the FAS successfully. To clarify the difference in composition caused by fermentation, we analyzed the metabolic profiles of NFAS and FAS by ultra-high-performance liquid chromatography-quadrupole time-of-flight mass spectroscopy (UHPLC-QTOF-MS). We further studied the effects and mechanisms of FAS on liver oxidative stress induced by D-galactose (D-gal) in vivo. The purpose of this study was to evaluate whether the FAS can improve aging by regulating the Nrf2-mediated antioxidant defense pathway to improve liver oxidative stress. Meanwhile, to maximize the understanding of the potentially effective interventions of FAS on aging, we evaluated the gut microbiota and fecal metabolites in senescent mice treated with FAS. The results of the gut microbiota and metabolomics analysis may provide a basis to develop postbiotic products as well as a new understanding of the molecular mechanism of FAS-mediated aging therapy.
Materials and methods
Preparation of FAS and NFAS
Lactobacillus plantarum LZU-J-TSL6 and LZU-S-ZCJ were isolated from JiangShui and yogurt, respectively. The strains were identified by 16S rRNA gene sequence analysis (ESI Tables 1 and 2†) and were deposited in the Guangdong Microbial Culture Collection Center (GDMCC), Guangzhou, China, under accession number GDMCC 61242 and GDMCC 61402. AS was purchased by Deshengtang Group Co., Ltd (Lanzhou, China). The following are the preparation details:
NFAS: An appropriate amount of AS powder was extracted two times with 10 volumes of distilled water and the filtrate was obtained. The filtrate was concentrated to a specific concentration (0.6 g mL−1) and the supernatant was collected by centrifugation and sterilized.
FAS: The final concentration of Lactobacillus plantarum LZU-J-TSL6 and LZU-S-ZCJ in the culture was approximately 1012 CFU mL−1. Next, Lactobacillus plantarum LZU-J-TSL6 and LZU-S-ZCJ were mixed in a ratio of 1
:
3 and added into NFAS with 4% initial inoculum. After that, the mixture was fermented at 36 °C for 72 hours. Next, the supernatant was sterilized and centrifuged to obtain FAS. It is worth emphasizing that FAS does not contain living microorganisms.
Component analysis of FAS and NFAS
The samples were analyzed using UHPLC-QTOF-MS (Agilent Technologies, AB Sciex, CA, USA). The samples FAS and NFAS were added to 1000 μL of extracting solution (methanol
:
water = 4
:
1, v/v). All samples were vortexed for 60 s and centrifuged at 12
000 rpm and 4 °C for 10 min. 400 μL of samples were filtered through a 0.22 μm filter membrane for UHPLC-QTOF-MS analysis. The column used was UPLC BEH C18 (1.7 μm × 2.1 × 100 mm) at a flow rate of 400 μL min−1, and the injection volume was 5.0 μL. The samples were eluted using an acetonitrile gradient and a mobile phase consisting of A, water (0.1% formic acid) and B, acetonitrile (0.1% formic acid) with the gradient as follows: 0–3.5 min: 5%–15% B; 3.5–6 min: 15–30% B; 6–6.5 min: 30% B; 6.5–12 min: 30%–70% B; 12–12.5 min: 70% B; 12.5–18 min: 70%–100% B; 18–25 min: 100% B; 25–26 min: 100%–5% B; 26–30 min: 5% B. A Q Exactive Focus mass spectrometer coupled with Xcalibur software (Thermo Scientific, USA) was used to obtain the MS data based on the information dependent acquisition (IDA) mode. During each acquisition cycle, the mass range was from 100 to 1500 Da. The sheath gas flow rate was 45 arb, the aux gas flow rate was 15 arb, and the capillary temperature was 400 °C. The full MS resolution was 70
000. The collision energy was set to 15, 30, and 45 eV in the NCE mode. The spray voltage was set to +4.0 kV or −3.6 kV.
Improvement of FAS and NFAS on D-gal induced aging in vivo
Animals and experimental design.
The animal experimental schematic diagram is shown in Fig. 2A. Fifty male BALB/c mice (7 weeks old) were obtained from Lanzhou veterinary research institute (Lanzhou, China) and housed in a specific pathogen-free facility with ad libitum access to food and water. After a 7-day acclimation, the mice were randomly divided into five experimental groups (10 mice per group). The mice were intraperitoneally injected with D-gal at a dose of 2.5 g kg−1 once a day for 44 days, except for the mice in the control group that received an equal volume of normal saline. Meanwhile, two intervention groups, including the NFAS and FAS groups, were given 3 g kg−1 NFAS or FAS by oral gavage once daily after 2 weeks of D-gal administration. The positive control group (VC) was orally administered with vitamin C (120 mg kg−1) once daily after 2 weeks of D-gal administration. The control and D-gal model groups were treated with 100 μL of normal saline by oral gavage per day at the same feeding frequency.
At the end of the experiment, the blood samples were collected from the eyes and centrifuged at 1200g for 10 minutes to obtain serum. After the mice were sacrificed by cervical dislocation, the liver tissues and feces were obtained from each animal and stored at −80 °C for further analysis. All animal experiments were approved by the Ethics Committee of Lanzhou University (approval number: EAF2022034).
Biochemical assays.
Liver tissues rinsed with pre-cooled PBS (0.01 M, pH = 7.4) were cut into pieces and homogenized in cold normal saline solution with the help of a homogenizer. The supernatant of the homogenate centrifuged at 5000 rpm for 10 min was collected for testing. The BCA protein assay was used to determine the protein content in the supernatant. The levels of advanced glycation end products (AGEs), 8-hydroxy-2 deoxyguanosine (8-OHdG), SOD, CAT and GSH-Px in liver tissues were analyzed using enzyme-linked immunosorbent assay (ELISA, Nanjing Jiancheng Bioengineering Institute, Nanjing, China) kits following the instructions. The malondialdehyde (MDA) level and total antioxidant capacity (T-AOC) were determined according to the kit instructions (Nanjing Jiancheng Bioengineering Institute, Nanjing, China). The levels of pro-inflammatory cytokines IL-1β, IL-6 and TNF-α were determined using ELISA (Nanjing Jiancheng Bioengineering Institute, Nanjing, China) kits according to the instructions. The samples were added into a 96-well plate and run on a microplate reader (Bio-Rad iMARKTM, Shanghai, China). Each experiment was repeated three times, and the average optical density values were taken as statistics.
Hepatic histopathological changes.
Liver tissues were fixed with 10% neutral formalin fixative for 48 h. The fixed tissue was dehydrated, embedded in paraffin, sectioned, and stained with hematoxylin and eosin (H&E). The histological image using a fluorescence microscope with 40× magnification.
Nrf2 signaling gene expression by quantitative real-time PCR (qRT-PCR).
The relative expression levels of Nrf2 signaling genes (Nrf2, HO-1, NQO1, GCLC, GCLM and GSS) were determined using qRT-PCR. Total RNA was extracted from the liver tissues following the protocol by Servicebio, Inc. (Wuhan Servicebio Technology Co., Ltd, Wuhan, China) and reverse transcribed to cDNA using a Servicebio®RT First Strand cDNA Synthesis Kit (Servicebio, China). The relative expression levels of the selected genes were determined using a Bio-Rad PCR machine (Bio-Rad, USA). The standard cycle conditions are as follows: 50 °C for 2 minutes; 95 °C for 2 minutes, then 39 cycles of 95 °C for 15 s, different annealing temperatures for 15 s, and 72 °C for 1 minute. ACTB/GAPDH was used as an internal gene to normalize the selected genes. And the relative expression levels were calculated using the 2−ΔΔCt formula. Each sample in each group had three complex holes, and the average expression values were taken as statistics. The primer sequences of all the genes (ACTB, GAPDH, Nrf2, HO-1, NQO1, GCLC, GCLM and GSS) are provided in ESI Table 3.†
Changes in the gut microbiota of D-gal induced aging mice by FAS and NFAS
The feces of mice in each group after administration were collected for the detection of the gut microbiota. The total genome DNA was extracted from samples using the CTAB method (Qiagen, Hilden, Germany). The DNA concentration and purity were monitored by 1% agarose gel electrophoresis. According to the concentration, DNA was diluted to 1 μg μL−1 using sterile water. PCR primers containing a barcode (V3F: 5′-ACTCCTACGGGAGGCAGCA-3′; V4R: 5′- GGACTACHVGGGTWTCTAAT-3′) were used to amplify the bacterial 16S rRNA variable region V3–V4. All PCR reactions were carried out with Phusion® High-Fidelity PCR Master Mix (New England Biolabs, Ipswich, MA, USA). PCR products were purified with a Qiagen Gel Extraction Kit (Qiagen, Dusseldorf, Germany) and quantified using a Qubit 2.0 fluorometer (Thermo Fisher Scientific, Waltham, USA) and an Agilent bioanalyzer 2100 system. The library was sequenced using an Illumina NovaSeq platform and 250 bp paired-end reads were generated.
Changes in fecal metabolites before and after FAS administration
The feces of the mice in the FAS group before and after the FAS administration were collected (BFAS/AFAS). After processing the samples, ultra-performance liquid chromatography-Q Exactive hybrid quadrupole-Orbitrap mass spectrometry (UPLC-QE-MS) was used to detect and analyze the results. 50 mg of sample was weighed and added to an EP tube, followed by the addition of 1000 μL of extract solution (methanol
:
acetonitrile
:
water = 2
:
2
:
1, with the isotopically-labeled internal standard mixture). The samples were homogenized at 35 Hz for 4 min and sonicated for 5 min in an ice-water bath. The homogenization and sonication cycles were repeated 3 times. Then the samples were incubated for 1 h at −40 °C and centrifuged at 12
000 rpm (RCF = 13
800g, R = 8.6 cm) for 15 min at 4 °C. The resulting supernatant was transferred to a fresh glass vial for analysis. The quality control sample was prepared by mixing an equal aliquot of the supernatants from all samples. LC-MS/MS analyses were performed using a UHPLC system (Vanquish, Thermo Fisher Scientific) with a UPLC BEH Amide column (2.1 mm × 100 mm, 1.7 μm) coupled to a QE HFX mass spectrometer (Orbitrap MS, Thermo). The mobile phase consisted of 25 mM ammonium acetate and 25 mM ammonia hydroxide in water (pH = 9.75) (A) and acetonitrile (B). The auto-sampler temperature was 4 °C and the injection volume was 2 μL. The QE HFX mass spectrometer was used for its ability to acquire MS/MS spectra in the IDA mode with the control of the acquisition software (Xcalibur, Thermo). The acquisition software continuously evaluated the full scan MS spectrum. The electron spray ionization (ESI) source conditions were set as follows: the sheath gas flow rate was 30 arb, the aux gas flow rate was 25 arb, the capillary temperature was 350 °C, the full MS resolution was 60
000, the MS/MS resolution was 7500, and the collision energy was set to 10, 30, and 60 eV. In the NCE mode, the spray voltage is 3.6 kV or −3.2 kV, respectively.
Statistical analysis
Data were expressed as the mean ± standard deviation (SD). All experiments were repeated at least 3 times by independent assays.
The statistical significance of data comparisons was determined using one-way analysis of variance (ANOVA), followed by Duncan's multiple range test or Student's t-test using GraphPad Prism 8.0 (GraphPad Software Inc., USA). Values of p < 0.05 were considered to be statistically significant.
Results
Component analysis of FAS and NFAS
Two ionization modes are included in electrospray ionization: the positive ion (POS) mode and the negative ion (NEG) mode. A combination of the two modes can make the metabolite coverage rate higher and the detection effect better when detecting the metabolome. We illustrated the NEG mode as an example. The detailed compounds with increased or decreased contents of FAS vs. NFAS are shown in ESI Tables 4 and 5.† In the NEG mode, FAS and NFAS were separated in the PCA score plot (Fig. 1A), indicating that the chemical compositions of FAS and NFAS were significantly different. The OPLS-DA score plot revealed an evident separation between the FAS and the NFAS, confirming the PCA simultaneously (Fig. 1B). The R2Y values were 0.98, and Q2 values were 0.25, indicating that the original model has good robustness and there is no overfitting phenomenon (Fig. 1C). The scatter size represented the VIP value of the OPLS-DA model, and the larger the scatter point, the greater the VIP value. Scatter colors represented the final screening results, with significantly upregulated metabolites indicated in red, significantly downregulated metabolites in blue, and no significant differential metabolites in gray (Fig. 1D). A total of 203 compounds were screened by the values of the S-plot and the VIPs, including 66 alkaloids, 48 phenylpropanoids, 11 organic acids, 24 phenolic compounds, 29 carbohydrates, 15 flavonoids, 26 terpenoids and 8 amino acid derivatives. We found that 23 substances were differentially increased and 32 substances were differentially decreased in FAS (Fig. 1E). In addition, the top 15 components with increased/decreased FAS contents were also demonstrated (Fig. 1F). Notably, 3-phenyllactic acid, L-5-hydroxytryptophan and methyl gallate were most significantly up-regulated after fermentation (p < 0.001). These results suggest the existence of biosynthetic and degradation processes in fermentation, which ultimately lead to an obvious difference between FAS and NFAS.
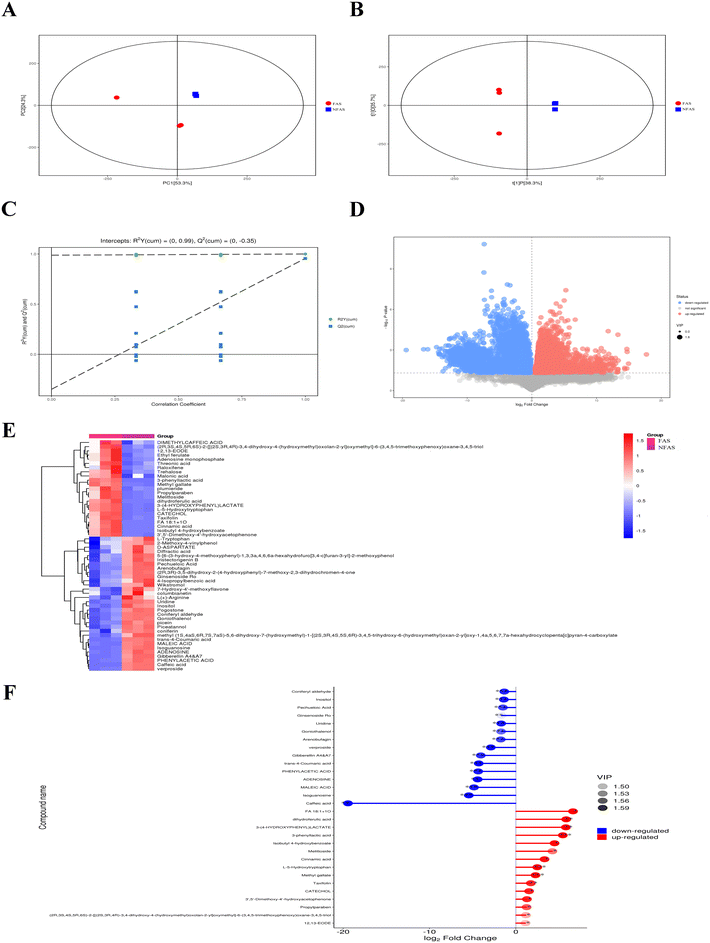 |
| Fig. 1 Compositional distinctions of FAS and NFAS in NEG. (A) The PCA score scatter plot of FAS vs. NFAS, (B) the OPLS-DA score scatter plot of FAS vs. NFAS, (C) the OPLS-DA permutation test of FAS vs. NFAS, (D) the volcano plot of FAS vs. NFAS, (E) the heat map of hierarchical cluster analysis of FAS vs. NFAS, (F) the matchstick plot of the top 15 components with increased/decreased contents of FAS vs. NFAS. *p < 0.05, **p < 0.01 and ***p < 0.001: significantly different compared with NFAS. (NEG: negative ion mode, FAS: fermented Angelica sinensis, NFAS: non-fermented Angelica sinensis). | |
Combined with the results in the NEG and POS modes, the alkaloids represented by haplophyllidine and tubotaiwine disappeared after fermentation, and their contents changed the most (p < 0.001). The contents of most flavonoids, such as rutin and flavanone, decreased slightly after fermentation, while the contents of taxifolin and licoflavone A were higher than NFAS, especially the content of taxifolin increased significantly (p < 0.01). In addition, AS is rich in carbohydrates. Among the carbohydrates detected in this study such as glucose, sucrose, trehalose, ribose and arabinose, trehalose increased the most. Phenolic compounds have a good antioxidant potential due to their high phenolic hydroxyl substitution activity and ability to phagocytose free radicals. In FAS, the contents of acetyl vanillin, vanillin, polydatin, rhaponticin, 2-hydroxyacetophenone, catechol, isobutyl 4-hydroxybenzoate, protosappanin B, phenol, zearalenone and methyl gallate increased. Among them, the contents of protosappanin B and methyl gallate increased most significantly (p < 0.01). The organic acid compounds in NFAS, including malonic acid, 3-(4-hydroxyphenyl) lactic acid and threonic acid, increased in content after fermentation. However, the contents of loganic acid, 12-oxo-phytodienoic acid, γ-linolenic acid and maleic acid decreased, and loganic acid was the most pronounced (p < 0.001). In summary, compared with NFAS, the prominently increased components in FAS, such as 3-phenyllactic acid, L-5-hydroxytryptophan, methyl gallate, taxifolin and trehalose, may contribute to the ability of FAS to improve liver aging. Meanwhile, the antioxidant activities of FAS were examined in vitro. The results indeed showed that the antioxidant capacity of FAS was effectively improved after fermentation (ESI Fig. 1†).
Effect of FAS on D-gal induced aging in mice
Effect of FAS on the body weight.
During the modeling and administration period, the food intake of the mice in each group was stable. The daily food intake of the control group was generally higher than that of the administration group. Meanwhile, the food intake of the mice in each group would also alter with a change in external factors such as cages and water changes. Throughout the experimental period, the body weight of mice in each group had been rising slowly as a whole, and the body weight would also fluctuate according to the food intake. In short, compared with the control group, D-gal intake did not affect the weight gain of mice.
Effect of FAS on the biochemical indexes of the liver.
The level of the antioxidant capacity of the liver is shown in Fig. 2. Compared with the control group, the contents of MDA, AGEs and 8-OHdG in the liver of D-gal treated mice showed a remarkable increase, which proved that the liver was already in an aging state (Fig. 2B–D). The activities of SOD, CAT, GSH-Px and T-AOC were significantly decreased, suggesting the presence of oxidative stress in the liver (Fig. 2E–H). Not surprisingly, the production of pro-inflammatory cytokines TNF-α, IL-1β and IL-6 in the liver was induced by oxidative stress (Fig. 2I–K). In contrast, FAS and NFAS administration reversed these parameters related to aging and oxidative stress. Moreover, FAS exhibited a stronger antioxidant effect comparable to VC.
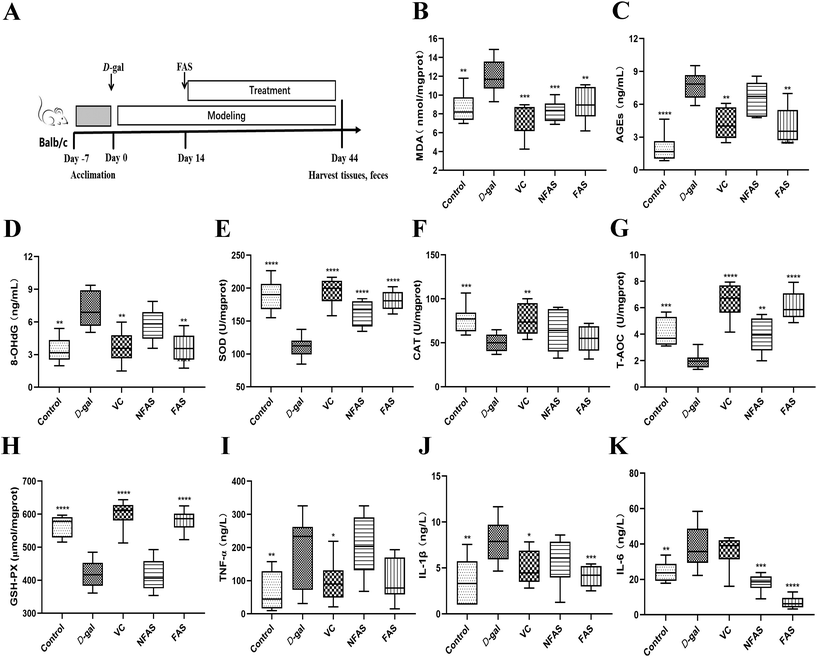 |
| Fig. 2 Effect of FAS on the contents of aging-related indexes, antioxidant indexes and pro-inflammatory indexes in liver tissue of mice. (A) Schematic diagram of the animal experiment, (B) MDA, (C) AGEs, (D) 8-OHdG, (E) SOD, (F) CAT, (G) T-AOC, (H) GSH-Px, (I) TNF-α, (J) IL-1β, and (K) IL-6. All data are expressed as mean ± SD. *p < 0.05, **p < 0.01, ***p < 0.001 and ****p < 0.0001: significantly different compared with the D-gal model group. (FAS: fermented Angelica sinensis, NFAS: non-fermented Angelica sinensis, VC: vitamin C, D-gal: D-galactose). | |
Effect of FAS on hepatic histopathological changes.
A normal hepatic architecture was observed in the control group mice (Fig. 3A), while D-gal exposure caused visible histological damage. The representative photomicrograph of the liver from the model group is presented in Fig. 3B, including swollen hepatocytes, cytoplasmic vacuolization and ballooning degeneration. FAS, NFAS and VC treatments prevented liver injuries, such as the loss of ballooning degeneration and the alleviation of cytoplasmic vacuolization (Fig. 3C, D and E). Nonetheless, they exhibited different protective effects on the hepatic tissues. The improvement was more evident in the FAS group, which was similar to that observed in the control group (Fig. 3A).
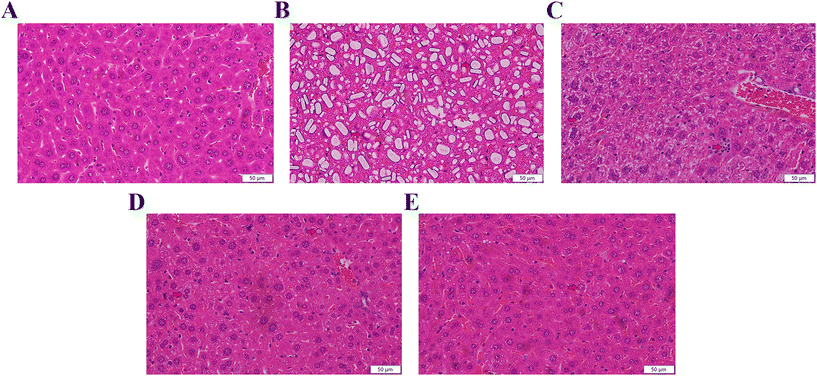 |
| Fig. 3 Effect of FAS on hepatic histopathological analysis (hematoxylin and eosin, magnification ×40). (A) Control group, (B) D-gal group, (C) VC group, (D) NFAS group, (E) FAS group. (FAS: fermented Angelica sinensis, NFAS: non-fermented Angelica sinensis, VC: vitamin C, D-gal: D-galactose). | |
Effect of FAS on Nrf2 signaling gene expression.
The possible mechanism underlying the regulation effect of FAS on oxidative stress in D-gal induced aging mice was further investigated. To identify whether the Nrf2 signaling plays a role in this protective process, the expression level of Nrf2 in the liver was measured. As shown in Fig. 4A, the expression of Nrf2 was significantly inhibited by D-gal (p < 0.05) compared with that of the control group and this downregulation was improved by FAS (p < 0.05). These findings indicated that FAS could activate Nrf2 signaling. To confirm the Nrf2-dependent antioxidant response of FAS, the expressions of Nrf2-downstream target genes were also determined, including HO-1, NQO1, GCLC, GCLM and GSS (Fig. 4B–F). As expected, FAS significantly increased the expressions of HO-1, NQO1, GCLC, GCLM and GSS (p < 0.05, p < 0.001, p < 0.001, p < 0.001 and p < 0.01) in the liver. These results implied that FAS could activate Nrf2 signaling and resulted in the expression of multiple antioxidant genes including HO-1, NQO1, GCLC, GCLM and GSS, further regulating oxidative stress.
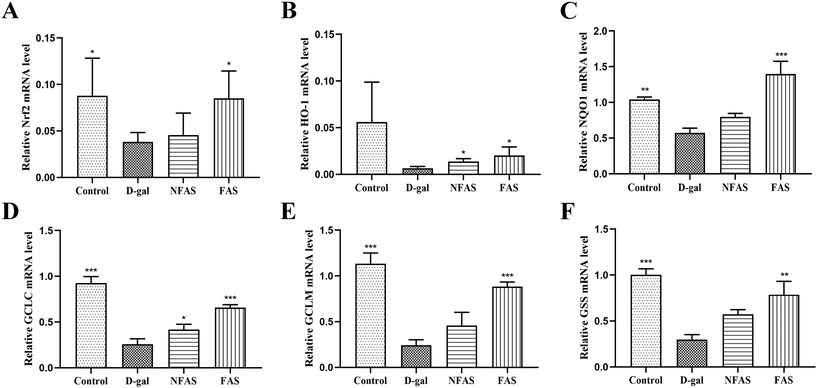 |
| Fig. 4 Effect of FAS on Nrf2 and Nrf2-downstream target genes in the liver tissue of mice. (A) Nrf2, (B) HO-1, (C) NQO1, (D) GCLC, (E) GCLM, and (F) GSS. All data are expressed as mean ± SD. *p < 0.05, **p < 0.01 and ***p < 0.001: significantly different compared with D-gal model mice. (FAS: fermented Angelica sinensis, NFAS: non-fermented Angelica sinensis, D-gal: D-galactose). | |
Effect of FAS on the intestinal microbiota in D-gal induced aging mice
Intestinal microbiota diversity.
The Chao 1 index, observed species and Shannon index are commonly used to evaluate the richness and diversity of bacterial species. As shown in Fig. 5A–C, exposure to D-gal could increase the Chao 1 index, observed species and Shannon index, indicating that D-gal induced oxidative stress in aging mice altered the phylotypic diversity of the gut microbiota. The FAS supplementation reversed this undesirable situation and decreased these indices. It revealed that FAS could reshape the microbial richness and diversity of the gut bacteria, which may be achieved by reducing the intestinal harmful bacterial species. The mutual and unique operational taxonomic units (OTUs) among each group were expressed by the Venn plot in Fig. 5D. 6136 OTUs were mutual in all groups, implying the presence of a strong core microbiota. The species accumulation curve reflected the increase in species upon increasing the sample size, and it is clear that the sample size was sufficient for species richness estimation from Fig. 5E. The greatest species richness was in the model group, indicating that the harmful species associated with senescence gradually increased with the induction of D-gal (Fig. 5F). In contrast, the species richness of the control and FAS groups were similar and significantly less than that of the model group, suggesting that FAS contributes to restoring the changes in the OTU number caused by D-gal. To outline the difference between each group on the gut microbiota composition, principal coordinates analysis (PCoA) was used to calculate the beta diversity (Fig. 5G and H). PCoA with weighted UniFrac distance showed significant variability in beta diversity between the FAS and model groups (p < 0.01). The FAS and control groups clustered together due to high similarity in community structure, while the other groups differed more.
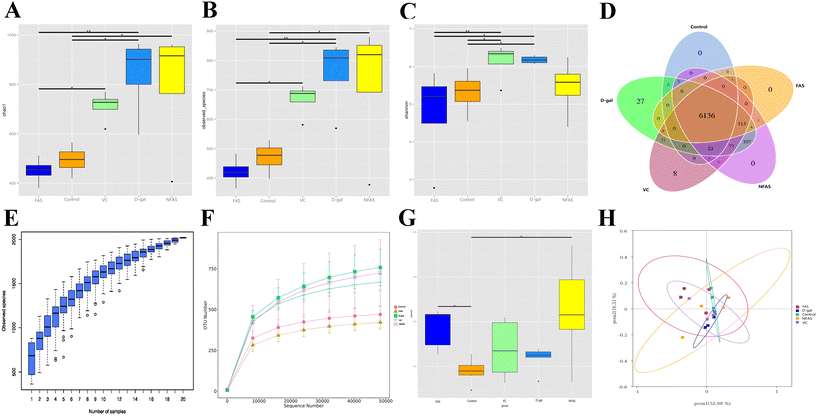 |
| Fig. 5 Alpha diversity and beta diversity analysis. (A) Chao 1 index, (B) observed species, (C) Shannon index, (D) Veen picture that illustrated the observed OTU counts in samples, (E) species accumulation curve, (F) rank abundance curve, (G) box plot based on the diversity of weighted unifrac beta, (H) PCoA based on the weighted UniFrac distance. (FAS: fermented Angelica sinensis, NFAS: non-fermented Angelica sinensis, VC: vitamin C, D-gal: D-galactose). | |
Changes in the intestinal microbiota at phylum and genus levels.
To further explore the difference in the gut microbiota composition, distinct microbial community structures were compared at different taxonomic levels among the groups. At the phylum level, the most abundant phyla in all groups were Bacteroidetes and Firmicutes. After FAS administration, the relative abundance of Bacteroidetes dropped from 57.72% to 41.60% compared with that of the control group. In contrast, the relative abundance of Firmicutes increased from 36.94% to 57.80% (Fig. 6A and C). The ratio of Firmicutes to Bacteroidetes increased by 95%. At the genus level, the growth of Lactobacillus, Alloprevotella and Bacteroides was inhibited and their abundance decreased significantly in the model group. Compared with the model group, the proportion of intestinal Lactobacillus in the FAS group rebounded and even exceeded that in the control group. However, the abundance of Lactobacillus in the NFAS group was lower than that in the control group, while the number of Alloprevotella was higher than that in the control group. On the other hand, the relative abundance of the Lachnospiraceae_NK4A136_group was greatly increased in the model group, which was most effectively reversed by FAS (Fig. 6B and D). The above results imply that FAS has a unique regulatory effect on the intestinal microbiota structure and this effect is completely different from that of NFAS. Furthermore, to demonstrate the correlation between different microbes and Nrf2 signaling, key microbes from Fig. 6B and Nrf2 signaling-related parameters were explored by Spearman correlation analysis. As shown in Fig. 6E. We found that Lactobacillus, Bacteroides and Alistipes were generally positively correlated with Nrf2 signaling-related parameters such as NQO1, HO-1, GCLC and GSS (p < 0.05). In contrast, Alloprevotella and Lachnospiraceae_NK4A136_group were negatively related to the total antioxidant capacity affected by Nrf2 signaling (p < 0.05). Therefore, it may explain the anti-aging effect of FAS through enriching Nrf2 signaling-related bacteria. Furthermore, we predicted the potential metabolic function of microbial communities by constructing a linear relationship between the SILVA classification and the KEGG (Kyoto Encyclopedia of Genes and Genomes) database. The result of metabolic analysis indicated that purine metabolism, pyrimidine metabolism, amino sugar and nucleotide sugar metabolism may be associated with FAS activating Nrf2 signaling and reducing oxidative stress in the liver (Fig. 6F).
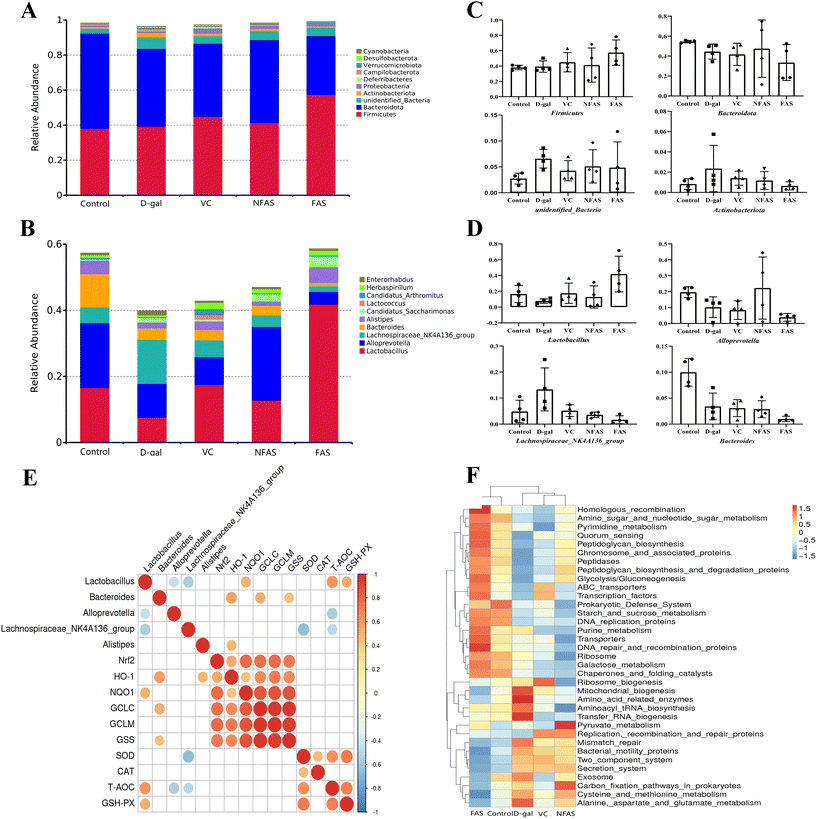 |
| Fig. 6 Analysis of the gut microbiota. (A) Phylum level of the gut microbiota composition, (B) genus level of the gut microbiota composition, (C) relative abundance of the four predominant gut microbes at the phylum level, (D) relative abundance of the four predominant gut microbes at the genus level, (E) correlation between key microbes and Nrf2 signaling-related parameters, (F) clustering heat map of the potential metabolic function. The red color denotes a positive correlation, while blue color denotes a negative correlation. The intensity of the color is proportional to the strength of spearman correlation. (FAS: fermented Angelica sinensis, NFAS: non-fermented Angelica sinensis, VC: vitamin C, D-gal: D-galactose). | |
Effect of FAS on changing the fecal metabolites
The PCA and OPLS-DA score plots in the NEG mode showed clear differences before and after FAS administration (Fig. 7A and B). The prediction results for R2 to describe the goodness of fit and Q2 to estimate the predictive power after cross-validation of the model were shown in Fig. 7C. It indicated that the PLS-DA model was reliable. The volcano map also suggested that the FAS deeply affected fecal metabolites in mice (Fig. 7D). Based on the METLIN database, identified 16 differential metabolites between the BFAS and AFAS, such as uric acid, ethyl tetradecanoate, erucic acid and allose (Fig. 7E, metabolites with VIP > 1 were considered as potential biomarkers). In addition, the AFAS group showed higher levels of pelargonic acid, saccharin, cyclamic acid, 3b-hydroxy-5-cholenoic acid and xanthoxylin than those in the BFAS group (p < 0.01). The metabolic pathway analysis revealed that purine metabolism, biosynthesis of unsaturated fatty acids, ascorbate and aldarate metabolism were regulated by FAS (Fig. 7F). Among them, the biosynthesis of unsaturated fatty acids, ascorbate and aldarate metabolism had a low impact. Notably, purine metabolism was found as the most influential metabolic pathway, which is consistent with the prediction of metabolic function based on gut microbes (Fig. 6F). These results confirmed that fecal metabolites responded to the FAS administration, effectively improved D-gal induced metabolism abnormalities and may contribute to anti-aging.
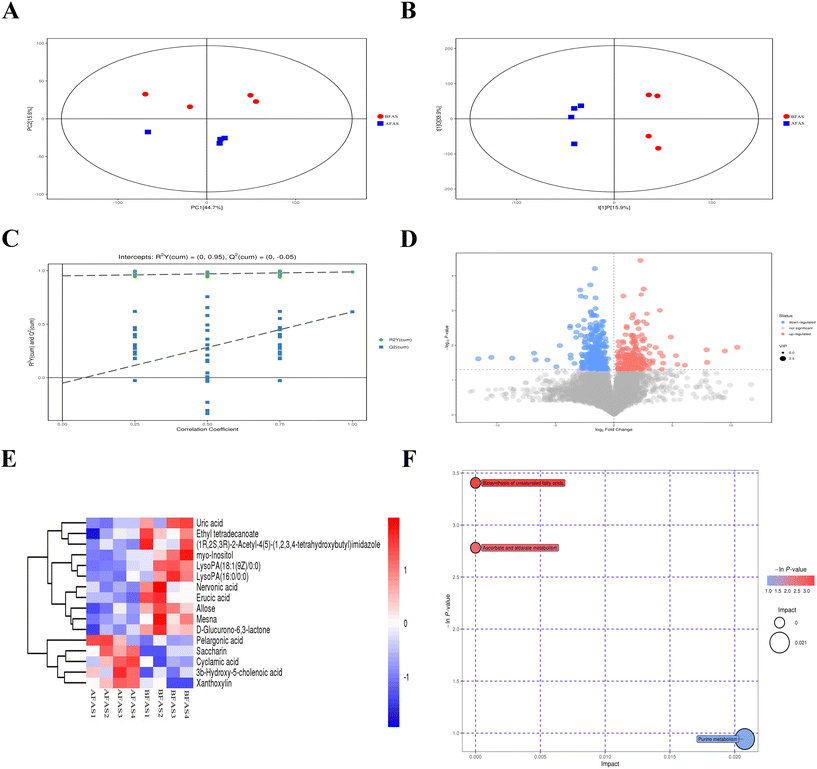 |
| Fig. 7 Effects of FAS on metabolites and metabolic pathways. (A) the PCA score scatter plot of AFAS vs. BFAS, (B) the OPLS-DA score scatter plot of AFAS vs. BFAS, (C) the OPLS-DA permutation test of AFAS vs. BFAS, (D) the volcano plot of screening differential metabolites of AFAS vs. BFAS, (E) the heat map of hierarchical cluster analysis of AFAS vs. BFAS, (F) the metabolic pathways involved in metabolic differentials. (BFAS/AFAS: mice in the FAS group before or after administration). | |
Discussion
Antioxidants can neutralize reactive oxygen and terminate oxidative stress before inducing damage to crucial molecules.32 Due to the conversion of consumer perception to foods as a source of therapeutic value, it is significant to explore effective antioxidants to protect the body from oxidative stress and slow down the aging progress.33 Thus, we used microbial fermentation technology to ferment AS, to further transform the active ingredients in AS, so as to expect the FAS to have a more effective effect on improving aging. Lactobacillus plantarum is capable of using polysaccharides and other substances in TCMs as carbon and energy sources by possessing abundant enzyme systems. These enzyme systems can help to dissolve the effective ingredients in TCMs.34 Meanwhile, the fermentation of TCMs under the action of microbial metabolism can transform the macromolecular substances into small molecules. The body can absorb these small active ingredients more easily, which further improves the efficacy of TCMs.35,36 For this reason, it is necessary to investigate the changed ingredients in FAS. Based on the results of the relative content changes of FAS and NFAS components, the main increased components after fermentation were analyzed. This current study found that multiple differential compounds were up-regulated in FAS. The production of these compounds was closely related to the enzyme systems in the fermentation system, which was involved in the decomposition process of the upstream compounds and the synthesis process of the downstream compounds. Among them, 3-phenyllactic acid, L-5-hydroxytryptophan and methyl gallate were up-regulated most significantly after fermentation (p < 0.001). In addition, the contents of other ingredients in FAS, such as taxifolin, cinnamic acid and trehalose, were also effectively increased (p < 0.05). Despite the availability of these ingredients, some compounds have not been extensively studied. The relationships between oxidative stress and several compounds have been established. For instance, methyl gallate, cinnamic acid and trehalose have been proven to help alleviate oxidative stress-mediated liver injury.37–39 Among them, a study has shown that trehalose is an effective antioxidant against D-gal induced aging via targeting the Nrf2 signaling.40 Another case is the compound 5-hydroxytryptophan, which has been pointed out to have the ability to scavenge free radicals and protect the hepatocyte membrane from lipid peroxidation.41 More importantly, numerous studies have shown that 5-hydroxytryptophan receptor activation is associated with aging and may improve aging-related complications such as cognitive impairment and vascular aging.42–44 As a new natural antibacterial and effective component, 3-phenyllactic acid has a positive effect on intestinal health, although there is no direct evidence for its relationship with oxidative stress in the liver.45,46 Therefore, the potential role of 3-phenyllactic acid in aging can be further explored in the future. In addition, increased taxifolin content is similarly associated with aging. During aging, oxidative stress initiates neuronal apoptosis, leading to neuronal loss. Interestingly, taxifolin has an antioxidant effect and can protect nerve cells.47,48 Therefore, it can effectively prevent chronic neurodegenerative diseases such as Parkinson's disease and Alzheimer's disease. Liu et al.'s study similarly showed that taxifolin alleviated oxidative stress damage and restored spatial learning and memory ability in D-gal induced mice.49 Previous studies have demonstrated the positive correlation between antioxidant capacity and antioxidant component content in different plant extracts, suggesting that these increased antioxidants after fermentation, such as taxifolin, cinnamic acid and trehalose, may improve the antioxidant capacity of FAS.50,51 Similarly, Liu et al. used Lactobacillus rhamnosus L08 to ferment apple pomace, and the antioxidant properties of apple pomace were significantly increased after fermentation.52 The above analysis of the increased ingredients in FAS could also help us partially explain why FAS can effectively improve liver aging.
We verified the antioxidant effects of FAS using a D-gal induced aging model, which is widely used in the study of oxidative stress injury. It has been reported when an excess of D-gal is given to mice, the D-gal is converted to non-degradable galactitol by enzymatic catalysis. Galactitol accumulates in cells and induces the production of reactive oxygen species.53 In general, continuous D-gal injection to mice for 6–8 weeks could induce oxidative stress damage and accelerate the degeneration of several organs such as the brain, liver and kidney. Among them, the liver plays an important role in maintaining homeostasis in the organism.54 In the current study, the increased levels of MDA, AGEs and 8-OHdG in the D-gal model mice indicated that oxidative stress in the liver had been successfully initiated. Not surprisingly, swollen hepatocytes, cytoplasmic vacuolization and ballooning degeneration were observed in the liver histopathological sections of D-gal induced aging mice, indicating severe hepatic oxidative damage. In contrast, FAS administration reduced the pathological changes in the liver and could achieve the same therapeutic effect as VC. The results also revealed that FAS was able to improve the total antioxidant capacity of the liver by increasing the activities of SOD, CAT and GSH-Px. It was similar to the finding of Zhao et al., which showed that nutritional intervention was able to increase liver antioxidant capacity to cope with oxidative stress.55 Inflammation is a crucial factor in the process of organismal aging and oxidative stress.56 Not surprisingly, the levels of pro-inflammatory factors TNF-α, IL-6 and IL-1β were significantly increased in the aging group, while FAS reduced these effects and promoted an overall anti-inflammatory profile. The above results confirm the anti-aging potential of FAS in alleviating oxidative stress in the liver.
Extensive studies have suggested that oxidative stress can activate a series of transcription factors including Nrf2, and lead to the expression of growth factors, inflammatory cytokines, chemokines, and anti-inflammatory molecules.57 The current study showed that FAS administration significantly decreased the pro-inflammatory factors, therefore, how oxidative stress induces inflammatory pathways was further investigated. The Nrf2 signaling is the predominant defense mechanism against oxidative stress damage. In general, Nrf2 is present at a very low cellular level. However, under oxidative stress, Nrf2 is translocated into the nucleus to bind to the antioxidant response element (ARE) and regulate the expression of downstream proteins such as SOD, CAT and GSH-Px.58 In the current study, the decreased SOD, CAT, GSH-Px activity and T-AOC induced by D-gal were reversed effectively by FAS administration, suggesting that the body's ability to scavenge free radicals has been improved. Furthermore, the mRNA expression levels of HO-1, NQO1, GCLC, GCLM and GSS were investigated and results demonstrated that FAS administration significantly increased the expression levels of these target genes. These results indicated that FAS activated the Nrf2 signaling and induced the expression of downstream anti-oxidase and inhibited the production of oxidative stress. Consistent with the observations in this study, Sun et al. found that trehalose was able to ameliorate D-gal induced oxidative stress in mice by activating Nrf2 signaling.40 Liu et al. reported that taxifolin also decreased the apoptosis of the aging brain by up-regulating Nrf2, HO-1 and NQO1 to maximally moderate the oxidative stress injury that occurred after D-gal induction.49 Therefore, the activation of FAS on Nrf2 signaling might be closely related to increased antioxidant components such as trehalose and taxifolin.
Recently, the role of the gut microbiota in aging has been the subject of intense investigation. It is well established that aging can lead to dysbiosis of microbiota and promotes inflammatory responses.14,59,60 To investigate whether gut microbiota alteration was associated with the protective effects of FAS, we determined the gut microbiota composition by 16S rRNA V3-V4 region sequencing technology. Previous studies have found that the diversity of the gut microbes increases during aging.60 Indeed, we found increased intestinal microbial diversity after D-gal induction, which was specifically manifested by the increase of observed species, Chao-1 and Shannon index. It indicated that D-gal could increase the number of harmful bacteria in mice. Conversely, FAS decreased these indices compared to D-gal-challenged mice, whereas FAS seemed to reach the same levels as normal mice. It is worth noting that NFAS can hardly change the increased microbial diversity caused by D-gal. On the other hand, FAS displayed excellent prebiotic properties, including inhibiting the proliferation of conditioned pathogenic bacteria and promoting the growth of beneficial bacteria. Our current work found that FAS reduced the relative abundance of pathogenic bacteria such as Lachnospiraceae_NK4A136, which was negatively correlated with SOD and T-AOC. In contrast, the reduced relative abundance of Lactobacillus was observed in D-gal induced mice, which was consistent with the study of Li et al..59 FAS significantly increased the abundance of Lactobacillus, while the abundance of Lactobacillus was quite low after NFAS administration. The major enriched Lactobacillus improved the ratio of Firmicutes to Bacteroidetes by 95%. The previous study has reported that reduced Firmicutes/Bacteroidetes is associated with the development of Alzheimer's disease and Parkinsonism.61 Meanwhile, we found that Lactobacillus was positively correlated with the NQO1 expression, GSH-PX activity and T-AOC. It suggested a close relationship among Lactobacillus, Nrf2 signaling and oxidative stress. Lactobacillus is the most common probiotic in the body. Previous studies have found that Lactobacillus can promote the expression of antioxidant enzymes by activating Nrf2 signaling in vivo.59,62 In addition, lactic acid, acetic acid and propionic acid produced by Lactobacillus can not only inhibit the growth of pathogenic bacteria to maintain the gut microbiota balance but also promote the production of butyrate.63,64 Butyrate can be transferred from the gut to the liver via the portal vein, where it further activates Nrf2 signaling and induces Nrf2-mediated antioxidant defense systems by modulating histone acetylases.65 Furthermore, unlike FAS, we found that NFAS had an ideal effect on increasing the abundance of Alloprevotella in the gut. Ning et al.'s studies pointed out that Alloprevotella has anti-inflammatory effects and is a potential probiotic.66,67 These results suggest that Alloprevotella may be one of the reasons NFAS plays an anti-aging role. Nevertheless, through fermentation, substances that favor the growth of Alloprevotella may be transformed into substances that favor the growth of Lactobacillus. This specific hypothesis remains to be validated in the future. The functional prediction based on the gut microbiota also showed that the enriched bacterial genera in the FAS group were related to purine metabolism, pyrimidine metabolism, aminoglycan and nucleotide sugar metabolism. The increased proportion of Lactobacillus observed in the FAS group might be related to the intestinal survival of Lactobacillus. It can be speculated that Lactobacillus may interact with the host through various metabolic pathways such as glucose metabolism and lipid metabolism, activate Nrf2 signaling through the liver-gut axis, and reduce oxidative stress injury in the liver.
Based on the above, we elucidated the underlying mechanism of oxidative stress response during aging by analyzing the overall metabolic characteristics of FAS in feces. A previous study proposed that 7 metabolic pathways such as amino acid metabolism, energy metabolism, intestinal microbiota metabolism, and metabolism of short-chain fatty acids were recognized as the influenced pathways associated with gastrointestinal functions of aging rats induced by D-gal.68 Complementarily, we found that purine metabolism, ascorbate and aldarate metabolism, and biosynthesis of unsaturated fatty acids play a role in the present study. Among them, purine metabolism had the most significant effect. Uric acid is the intermediate of purine metabolism in the liver and is excreted by the kidneys. The presence of uric acid as a differential metabolite was detected in the fecal metabolites of mice in the AFAS group versus the BFAS group, inferring that might be a mediator to resist oxidative stress. From the hierarchical clustering plot, the AFAS group had lower expression of uric acid in the fecal metabolites. The functional prediction based on the gut microbiota has shown that FAS increases the abundance of gut bacteria associated with purine metabolism. This result is also consistent with the regulation of uric acid, an important metabolite in purine metabolism. Plenty of studies have shown Lactobacillus can absorb purine and reduce the intestinal transport of purine.69–71 Kuo et al. found that Lactobacillus reuteri TSR332 and Lactobacillus fermentum TSF331 could stabilize serum uric acid levels, regulate purine metabolism and prevent hyperuricemia in rats.71 In the current study, it was also found that the abundance of Lactobacillus increased after FAS administration. Therefore, it was inferred that the high expression of uric acid in mice before FAS administration might be a compensatory mechanism in the body, which sought uric acid in response to oxidative stress induced by D-gal. Conversely, FAS could regulate purine metabolism by increasing the abundance of Lactobacillus and further excreted uric acid oxidative productions through producing allantoic acid.72 It also supports the idea that FAS does achieve anti-aging by regulating the gut microbiota and metabolism.
Conclusions
Collectively, fermentation is an effective way to optimize the therapeutic potential of Angelica sinensis. Compared to NFAS, the increased components in FAS had a positive meaning for FAS to enhance the ability against liver aging. In vivo investigation indicated that FAS effectively attenuated D-gal induced liver aging in mice by activating Nrf2 signaling and modulating the gut microbiota composition and metabolism. This study may provide a scientific basis for the clinical application of FAS in the prevention and therapy of liver aging, and facilitate other oxidative stress-induced disorders.
Conflicts of interest
There is no conflict of interest associated with the authors of this manuscript.
Acknowledgements
This work was supported by the Gansu Province Key R&D Plan (No. 21YF5FA105), the Science and Technology Major Project of Gansu Province (No. 22ZD1FA001), the Guangzhou Science and Technology Planning Project (No. 202206010165), and the “Innovation Star” Project of the Excellent Postgraduate in Gansu Province (No. 2022CXZX-139).
References
- J. R. Beard, A. Officer, I. A. de Carvalho, R. Sadana, A. M. Pot, J. P. Michel, P. Lloyd-Sherlock, J. E. Epping-Jordan, GMEEG. Peeters, W. R. Mahanani, J. A. Thiyagarajan and S. Chatterji, The World report on ageing and health: a policy framework for healthy ageing, Lancet, 2016, 387, 2145–2154 CrossRef PubMed.
- X. Li, Y. Chen, S. Shao, Q. Tang, W. Chen, Y. Chen and X. Xu, Oxidative stress induces the decline of brain EPO expression in aging rats, Exp. Gerontol., 2016, 83, 89–93 CrossRef CAS PubMed.
- J. Park, J. S. Min, B. Kim, U. B. Chae, J. W. Yun, M. S. Choi, I. K. Kong, K. T. Chang and D. S. Lee, Mitochondrial ROS govern the LPS-induced pro-inflammatory response in microglia cells by regulating MAPK and NF-κB pathways, Neurosci. Lett., 2015, 584, 191–196 CrossRef CAS PubMed.
- S. Yuan, Y. Yang, J. Li, X. Tan, Y. Cao, S. Li, H. D. Hong, L. Liu and Q. Zhang, Ganoderma lucidum Rhodiola compound preparation prevent D-galactose-induced immune impairment and oxidative stress in aging rat model, Sci. Rep., 2020, 10, 19244 CrossRef CAS PubMed.
- S. Mirzaei, A. Zarrabi, F. Hashemi, A. Zabolian, H. Saleki, N. Azami, S. Hamzehlou, M. V. Farahani, K. Hushmandi, M. Ashrafizadeh, H. Khan and A. P. Kumar, Nrf2 Signaling Pathway in Chemoprotection and Doxorubicin Resistance: Potential Application in Drug Discovery, Antioxidants, 2021, 10, 349 CrossRef CAS PubMed.
- W. Tu, H. Wang, S. Li, Q. Liu and H. Sha, The Anti-Inflammatory and Anti-Oxidant Mechanisms of the Keap1/Nrf2/ARE Signaling Pathway in Chronic Diseases, Aging Dis., 2019, 10, 637–651 CrossRef PubMed.
- P. Shaw and A. Chattopadhyay, Nrf2-ARE signaling in cellular protection: Mechanism of action and the regulatory mechanisms, J. Cell Physiol., 2020, 235, 3119–3130 CrossRef CAS PubMed.
- P. W. O'Toole and I. B. Jeffery, Gut microbiota and aging, Science, 2015, 350, 1214–1215 CrossRef PubMed.
- M. F. Sun and Y. Q. Shen, Dysbiosis of gut microbiota and microbial metabolites in Parkinson's Disease, Ageing Res. Rev., 2018, 53–61 CrossRef PubMed.
- F. Zhang, F. He, L. Li, L. C. Guo, B. Zhang, S. H. Yu and W. Zhao, Bioavailability Based on the Gut Microbiota: a New Perspective, Microbiol. Mol. Biol. Rev., 2020, 84, e00072–e00019 CrossRef CAS PubMed.
- B. L. Li, P. Du, E. E. Smith, S. Wang, Y. H. Jiao, L. D. Guo, G. C. Huo and F. Liu,
In vitro and in vivo evaluation of an exopolysaccharide produced by Lactobacillus helveticus KLDS1.8701 for the alleviative effect on oxidative stress, Food Funct., 2019, 10, 1707–1717 RSC.
- Y.A. Park, S. R. Lee, J. W. Lee, H. J. Koo, S.A. Jang, S.W. Yun, H. J. Kim, J. S. Woo, M. R. Park, S. C. Kang, Y. K. Kim and E.H. Sohn, Suppressive Effect of Fermented Angelica tenuissima Root Extract against Photoaging: Possible Involvement of Hemeoxygenase-1, J. Microbiol. Biotechnol., 2018, 28, 1391–1400 CrossRef CAS PubMed.
- M. J. Han and D.H. Kim, Effects of Red and Fermented Ginseng and Ginsenosides on Allergic Disorders, Biomolecules, 2020, 10, 634 CrossRef CAS PubMed.
- Y. Gao, B. Li, H. Liu, Y. Tian, C. Gu, X. Du, R. Bu, J. Gao, Y. Liu and G. Li,
Cistanche deserticola polysaccharides alleviate cognitive decline in aging model mice by restoring the gut microbiota-brain axis, Aging, 2021, 13, 15320–15335 CrossRef CAS PubMed.
- C. Y. Shen, J. G. Jiang, Y. Li, D. W. Wang and W. Zhu, Anti-ageing active ingredients from herbs and nutraceuticals used in traditional Chinese medicine: pharmacological mechanisms and implications for drug discovery, Br. J. Pharmacol., 2017, 174, 1395–1425 CrossRef CAS PubMed.
- H. Y. Wang, Y. Y. Dun, D. Yuan, M. Liu, Y. H. Guo and C. C. Zhang, Effects of the Saponins from Panax japonicus on Intestinal Epithelial Tight Junction Proteins in Aging Rats, Zhongyaocai, 2016, 39, 2091–2096 Search PubMed.
- S. Yan, Y. Z. Yue, X. P. Wang, H. L. Dong, S. G. Zhen, B. S. Wu and H. H. Qian, Aqueous Extracts of Herba Cistanche Promoted Intestinal Motility in Loperamide-Induced Constipation Rats by Ameliorating the Interstitial Cells of Cajal, J. Evidence-Based Complementary Altern. Med., 2017, 2017, 6236904 Search PubMed.
- H. Shi, B. Wang, C. Bian, Y. Han and H. Qiao, Fermented Astragalus in diet improved laying performance, egg quality, antioxidant and immunological status and intestinal microbiota in laying hens, AMB Express, 2020, 10, 159 CrossRef CAS PubMed.
- L. Wang, Q. M. Liu, B.H. Sung, D. S. An, H.G. Lee, S.G. Kim, S.C. Kim, S.T. Lee and W.T. Im, Bioconversion of ginsenosides Rb(1), Rb(2), Rc and Rd by novel β-glucosidase hydrolyzing outer 3-O glycoside from Sphingomonas sp. 2F2: cloning, expression, and enzyme characterization, J. Biotechnol., 2011, 156, 125–133 CrossRef CAS PubMed.
- T. B. Dey, S. Chakraborty, K. K. Jain, A. Sharma and R. C. Kuhad, Antioxidant phenolics and their microbial production by ubmerged and solid state fermentation process: A review, Trends Food Sci. Technol., 2016, 53, 60–74 CrossRef.
- L. C. Yang, T. J. Fu and F. C. Yang, Biovalorization of soybean residue (okara) via fermentation with Ganoderma lucidum and Lentinus edodes to attain products with high anti-osteoporotic effects, J. Biosci. Bioeng., 2020, 129, 514–518 CrossRef CAS PubMed.
- S. M. Hong, M. C. Kang, M. Jin, T. H. Lee, B. O. Lim and S. Y. Kim, Fermented blueberry and black rice containing Lactobacillus plantarum MG4221: a novel functional food for particulate matter (PM2.5)/dinitrochlorobenzene (DNCB)-induced atopic dermatitis, Food Funct., 2021, 12, 3611–3623 RSC.
- T. L. Lin, C. C. Lu, W. F. Lai, T. S. Wu, J. J. Lu, Y. M. Chen, C. M. Tzeng, H. T. Liu, H. Wei and H. C. Lai, Role of gut microbiota in identification of novel TCM-derived active metabolites, Protein Cell, 2021, 12, 394–410 CrossRef PubMed.
- Z. R. Huang, M. Chen, W. L. Guo, T. T. Li, B. Liu, W. D. Bai, L. Z. Ai, P. F. Rao, L. Ni and X. C. Lv, Monascus purpureus-fermented common buckwheat protects against dyslipidemia and non-alcoholic fatty liver disease through the regulation of liver metabolome and intestinal microbiome, Food Res. Int., 2020, 136, 109511 CrossRef CAS PubMed.
- J. J. Wen, M. Z. Li, H. Gao, J. L. Hu, Q. X. Nie, H. H. Chen, Y. L. Zhang, M. Y. Xie and S. P. Nie, Polysaccharides from fermented Momordica charantia L. with Lactobacillus plantarum NCU116 ameliorate metabolic disorders and gut microbiota change in obese rats, Food Funct., 2021, 12, 2617–2630 RSC.
- H. T. Shi, B. Y. Wang, C. Z. Bian, Y. Q. Han and H. X. Qiao, Fermented Astragalus in diet improved laying performance, egg quality, antioxidant and immunological status and intestinal microbiota in laying hens, AMB Express, 2020, 10, 159 CrossRef CAS PubMed.
- L. Zhang, Y. Jiang, X. Pang, P. Hua, X. Gao, Q. Li and Z. Li, Simultaneous Optimization of Ultrasound-Assisted Extraction for Flavonoids and Antioxidant Activity of Angelica keiskei Using Response Surface Methodology (RSM), Molecules, 2019, 24, 3461 CrossRef CAS PubMed.
- L. Zhang, J. Yu, Q. Xu, J. Zhu, H. Zhang, G. Xia and H. Zang, Evaluation of total phenolic, flavonoid, carbohydrate contents and antioxidant activities of various solvent extracts from Angelica amurensis root, Nat. Prod. Res., 2020, 4084–4088 Search PubMed.
- Q. Yuan, J. Zhang, C. Xiao, C. Harqin, M. Ma, T. Long, Z. Li, Y. Yang, J. Liu and L. Zhao, Structural characterization of a low-molecular-weight polysaccharide from Angelica pubescens Maxim. f. biserrata Shan et Yuan root and evaluation of its antioxidant activity, Carbohydr. Polym., 2020, 236, 116047 CrossRef CAS.
- C. Zhuang, Y. Wang, Y. Zhang and N. Xu, Oxidative stress in osteoarthritis and antioxidant effect of polysaccharide from angelica sinensis, Int. J. Biol. Macromol., 2018, 115, 281–286 CrossRef CAS PubMed.
- R. Guo, S. Guo, X. Gao, H. Wang, W. Hu, R. Duan, T. T. X. Dong and K. W. K. Tsim, Fermentation of Danggui Buxue Tang, an ancient Chinese herbal mixture, together with Lactobacillus plantarum enhances the anti-diabetic functions of herbal product, Chin. Med., 2020, 15, 98 CrossRef CAS PubMed.
- K. Zhang, J. Tan, C. Xie, R. Zheng, X. Huang, M. Zhang and M. Zhao, Antioxidant Effects and Cytoprotective Potentials of Herbal Tea against H2O2-Induced Oxidative Damage by Activating Heme Oxygenase1 Pathway, Biomed. Res. Int., 2020, 2020, 7187946 Search PubMed.
- C. Zhuang, S. Ni, Z. C. Yang and R. P. Liu, Oxidative Stress Induces Chondrocyte Apoptosis through Caspase-Dependent and Caspase-Independent Mitochondrial Pathways and the Antioxidant Mechanism of Angelica Sinensis Polysaccharide, Oxid. Med. Cell. Longevity, 2020, 2020, 3240820 Search PubMed.
- T. Ahmed, M. R. Rana, W. Zzaman, R. Ara and M. G. Aziz, Optimization of substrate composition for pectinase production from Satkara (Citrus macroptera) peel using Aspergillus niger-ATCC 1640 in solid-state fermentation, Heliyon, 2021, 7, e08133 CrossRef CAS PubMed.
- A. Hussain, S. Bose, J.-H. Wang, M. K. Yadav, G. B. Mahajan and H. Kim, Fermentation, a feasible strategy for enhancing bioactivity of herbal medicines, Food Res. Int., 2016, 81, 1–16 CrossRef CAS.
- C. Stanton, R. P. Ross, G. F. Fitzgerald and D. Van Sinderen, Fermented functional foods based on probiotics and their biogenic metabolites, Curr. Opin. Biotechnol., 2005, 16, 198–203 CrossRef CAS PubMed.
- B. I. Ryu and K. T. Kim, Antioxidant activity and protective effect of methyl gallate against t-BHP induced oxidative stress through inhibiting ROS production, Food Sci. Biotechnol., 2022, 31, 1063–1072 CrossRef CAS.
- E. Babaeenezhad, N. Nouryazdan, M. Nasri, H. Ahmadvand and M. Moradi Sarabi, Cinnamic acid ameliorate gentamicin-induced liver dysfunctions and nephrotoxicity in rats through induction of antioxidant activities, Heliyon, 2021, 7, e07465 CrossRef CAS PubMed.
- Y. Honma, M. Sato-Morita, Y. Katsuki, H. Mihara, R. Baba, K. Hino, A. Kawashima, T. Ariyasu and M. Harada, Trehalose alleviates oxidative stress-mediated liver injury and Mallor-Denk body formation via activating autophagy in mice, Med. Mol. Morphol., 2021, 54, 41–51 CrossRef CAS PubMed.
- L. Sun, Q. Zhao, Y. Xiao, X. Liu, Y. Li, J. Zhang, J. Pan and Z. Zhang, Trehalose targets Nrf2 signal to alleviate d-galactose induced aging and improve behavioral ability, Biochem. Biophys. Res. Commun., 2020, 521, 113–119 CrossRef CAS PubMed.
- M. C. Reyes-Gonzales, L. Fuentes-Broto, E. Martínez-Ballarín, F. J. Miana-Mena, C. Berzosa, F. A. García-Gil, M. Aranda and J. J. García, Effects of tryptophan and 5-hydroxytryptophan on the hepatic cell membrane rigidity due to oxidative stress, J. Membr. Biol., 2009, 231, 93–99 CrossRef CAS PubMed.
- R. Radhakrishnan, N. Nabulsi, E. Gaiser, J. D. Gallezot, S. Henry, B. Planeta, S. F. Lin, J. Ropchan, W. Williams, E. Morris, D. C. D'Souza, Y. Huang, R. E. Carson and D. Matuskey, Age-Related Change in 5-HT(6) Receptor Availability in Healthy Male Volunteers Measured with (11)C-GSK215083 PET, J. Nucl. Med., 2018, 59, 1445–1450 CrossRef CAS PubMed.
- E. S. Mitchell, R. A. McDevitt and J. F. Neumaier, Adaptations in 5-HT receptor expression and function: implications for treatment of cognitive impairment in aging, J. Neurosci. Res., 2009, 87, 2803–2811 CrossRef CAS PubMed.
- G. Froldi, M. Montopoli, M. Zanetti, P. Dorigo and L. Caparrotta, 5-HT1B receptor subtype and aging in rat resistance vessels, Pharmacology, 2008, 81, 70–78 CrossRef CAS PubMed.
- M. Li, X. Meng, Z. Sun, C. Zhu and H. Ji, Effects of NADH Availability on 3-Phenyllactic Acid Production by Lactobacillus plantarum Expressing Formate Dehydrogenase, Curr. Microbiol., 2019, 76, 706–712 CrossRef CAS PubMed.
- Q. Zhou, R. Gu, B. Xue, P. Li and Q. Gu, Phenyl lactic acid alleviates Samonella Typhimurium-induced colitis via regulating microbiota composition, SCFA production and inflammatory responses, Food Funct., 2021, 12, 5591–5606 RSC.
- C. Sunil and B. Xu, An insight into the health-promoting effects of taxifolin (dihydroquercetin), Phytochemistry, 2019, 166, 112066 CrossRef CAS.
- M. Tanaka, S. Saito, T. Inoue, N. Satoh-Asahara and M. Ihara, Potential Therapeutic Approaches for Cerebral Amyloid Angiopathy and Alzheimer's Disease, Int. J. Mol. Sci., 2020, 21, 1992 CrossRef CAS PubMed.
- X. L. Liu, Y. C. Zhao, H. Y. Zhu, M. Wu, Y. N. Zheng, M. Yang, Z. Q. Cheng, C. B. Ding and W. C. Liu, Taxifolin retards the D-galactose-induced aging process through inhibiting Nrf2-mediated oxidative stress and regulating the gut microbiota in mice, Food Funct., 2021, 12, 12142–12158 RSC.
- R. Socha, L. Juszczak, S. Pietrzyk and T. Fortuna, Antioxidant activity and phenolic composition of herbhoneys, Food Chem., 2009, 113, 568–574 CrossRef CAS.
- Y. Xing, L. Cai, T. Yin, Y. Chen, J. Yu, Y. Wang and Z. Ding, Improving the antioxidant activity and enriching salvianolic acids by the fermentation of Salvia miltiorrhizae with Geomyces luteus, J. Zhejiang Univ., Sci., B, 2016, 17, 391–398 CrossRef CAS PubMed.
- L. Liu, C. Zhang, H. Zhang, G. Qu, C. Li and L. Liu, Biotransformation of Polyphenols in Apple Pomace Fermented by β-Glucosidase-Producing Lactobacillus rhamnosus L08, Foods, 2021, 10, 1343 CrossRef CAS PubMed.
- H. Zhang, Z. Wang, Y. Li, J. Han, C. Cui, C. Lu, J. Zhou, L. Cheong, Y. Li, T. Sun, D. Zhang and X. Su, Sex-Based Differences in Gut Microbiota Composition in Response to Tuna Oil and Algae Oil Supplementation in a D-galactose-Induced Aging Mouse Model, Front. Aging Neurosci., 2018, 10, 187 CrossRef PubMed.
- D. J. Park, S. Ha, J. S. Choi, S. H. Lee, J. E. Park and Y. J. Seo, Induced Short-Term Hearing Loss due to Stimulation of Age-Related Factors by Intermittent Hypoxia, High-Fat Diet, and Galactose Injection, Int. J. Mol. Sci., 2020, 21, 7068 CrossRef CAS PubMed.
- J. Zhao, F. Tian, S. Yan, Q. Zhai, H. Zhang and W. Chen, Lactobacillus plantarum CCFM10 alleviating oxidative stress and restoring the gut microbiota in d-galactose-induced aging mice, Food Funct., 2018, 9, 917–924 RSC.
- G. H. Royce, H. M. Brown-Borg and S. S. Deepa, The potential role of necroptosis in inflammaging and aging, Geroscience, 2019, 41, 795–811 CrossRef PubMed.
- T. Wu, X. Wang, H. Xiong, Z. Deng, X. Peng, L. Xiao, L. Jiang and Y. Sun, Bioactives and their metabolites from Tetrastigma hemsleyanum leaves ameliorate DSS-induced colitis via protecting the intestinal barrier, mitigating oxidative stress and regulating the gut microbiota, Food Funct., 2021, 12, 11760–11776 RSC.
- D. D. Zhang and E. Chapman, The role of natural products in revealing NRF2 function, Nat. Prod. Rep., 2020, 37, 797–826 RSC.
- B. Li, S. E. Evivie, J. Lu, Y. Jiao, C. Wang, Z. Li, F. Liu and G. Huo, Lactobacillus helveticus KLDS1.8701 alleviates d-galactose-induced aging by regulating Nrf-2 and gut microbiota in mice, Food Funct., 2018, 9, 6586–6598 RSC.
- J. Zhang, X. Zhao, Y. Jiang, W. Zhao, T. Guo, Y. Cao, J. Teng, X. Hao, J. Zhao and Z. Yang, Antioxidant status and gut microbiota change in an aging mouse model as influenced by exopolysaccharide produced by Lactobacillus plantarum YW11 isolated from Tibetan kefir, J. Dairy Sci., 2017, 100, 6025–6041 CrossRef CAS PubMed.
- M. Sochocka, K. Donskow-Łysoniewska, B. S. Diniz, D. Kurpas, E. Brzozowska and J. Leszek, The Gut Microbiome Alterations and Inflammation-Driven Pathogenesis of Alzheimer's Disease-a Critical Review, Mol. Neurobiol., 2019, 56, 1841–1851 CrossRef CAS PubMed.
- D. Gao, Z. Gao and G. Zhu, Antioxidant effects of Lactobacillus plantarum via activation of transcription factor Nrf2, Food Funct., 2013, 4, 982–989 RSC.
- E. Jacouton, F. Chain, H. Sokol, P. Langella and L. G. Bermúdez-Humarán, Probiotic Strain Lactobacillus casei BL23 Prevents Colitis-Associated Colorectal Cancer, Front. Immunol., 2017, 8, 1553 CrossRef PubMed.
- V. P. Singh, J. Sharma, S. Babu, Rizwanulla and A. Singla, Role of probiotics in health and disease: a review, J. Pak. Med. Assoc., 2013, 63, 253–257 Search PubMed.
- W. Dong, Y. Jia, H. Zhang, T. Li, W. Huang, X. Chen, F. Wang, W. Sun and H. Wu, Sodium butyrate activates NRF2 to ameliorate diabetic nephropathy possibly via inhibition of HDAC, J. Endocrinol., 2016, 232, 71 Search PubMed.
- K. Ning, K. Lu, Q. Chen, Z. Guo, X. Du, F. Riaz, L. Feng, Y. Fu, C. Yin, F. Zhang, L. Wu and D. Li, Epigallocatechin Gallate Protects Mice against Methionine-Choline-Deficient-Diet-Induced Nonalcoholic Steatohepatitis by Improving Gut Microbiota to Attenuate Hepatic Injury and Regulate Metabolism, ACS Omega, 2020, 5, 20800–20809 CrossRef CAS PubMed.
- Q. Fan, M. Wanapat, T. Yan and F. Hou, Altitude influences microbial diversity and herbage fermentation in the rumen of yaks, BMC Microbiol., 2020, 20, 370 CrossRef CAS PubMed.
- D. Zhao, X. Liu, S. Zhao, Z. Li and X. Qin,
1H NMR-Based Fecal Metabolomics Reveals Changes in Gastrointestinal Function of Aging Rats Induced by d-Galactose, Rejuvenation Res., 2021, 24, 86–96 CrossRef CAS PubMed.
- H. Yamanaka, A. Taniguchi, H. Tsuboi, H. Kano and Y. Asami, Hypouricaemic effects of yoghurt containing Lactobacillus gasseri PA-3 in patients with hyperuricaemia and/or gout: A randomised, double-blind, placebo-controlled study, Mod. Rheumatol., 2019, 29, 146–150 CrossRef CAS PubMed.
- Y. Wu, Z. Ye, P. Feng, R. Li, X. Chen, X. Tian, R. Han, A. Kakade, P. Liu and X. Li, Limosilactobacillus fermentum JL-3 isolated from “Jiangshui” ameliorates hyperuricemia by degrading uric acid, Gut Microbes, 2021, 13, 1–18 CAS.
- Y. Kuo, S. Hsieh, J. Chen, C. Liu, C. Chen, Y. Huang and H. Ho,
Lactobacillus reuteri TSR332 and Lactobacillus fermentum TSF331 stabilize serum uric acid levels and prevent hyperuricemia in rats, PeerJ, 2021, 9, e11209 CrossRef PubMed.
- C. D. Todd, P. A. Tipton, D. G. Blevins, P. Piedras, M. Pineda and J. C. Polacco, Update on Ureide Degradation in Legumes, J. Exp. Bot., 2006, 57, 5–12 CrossRef CAS PubMed.
|
This journal is © The Royal Society of Chemistry 2023 |