Sonication of egg and its effect on foaming behavior
Received
17th April 2023
, Accepted 5th June 2023
First published on 5th June 2023
Abstract
In the food industry, eggs have been used as a multi-purpose ingredient for numerous applications. With their unique protein composition eggs exhibit excellent nutritional as well as functional properties. Among different proteins, egg white protein contributes the most to functional properties of food products, especially the foaming property. The natural foaming ability of egg proteins provides distinguished taste, texture, and mouthfeel making it a versatile raw material for the baking industry. With modified proteins exhibiting different applications as functional ingredients, egg proteins are subject to different treatments to modify their functional properties, especially foaming properties. Among the different treatments, ultrasound is a green technology that leaves no residues and has numerous advantages in terms of operation, sustainability, and energy consumption. The foaming properties of egg proteins are related to different processing parameters of ultrasound including power, amplitude, time, and frequency. Although the foaming ability is easily increased, maintaining or improving the foam stability is a major challenge. In addition, ultrasound assisted with other treatments such as irradiation, hydrolysis, and glycation that are proven to enhance the foaming properties of egg proteins are also discussed.
Sustainability spotlight
The phenomenon of foaming holds immense significance in the food industry. With its emphasis on eggs, this article focuses on the impact of ultrasound treatment on foaming behavior. Sonication is a green technology and offers numerous advantages in terms of operation, sustainability, and energy consumption, in addition to superior product quality. In combination with other approaches, commercially scalable systems can be developed, for egg foaming and other allied applications. As a sustainable food processing approach, the concept aligns with UN SDG 12.
|
1. Introduction
In food technology, foam formation is encouraged in the preparation of airy or low-density food products as it plays a functional role in texture, appearance, and taste of the final product. Foam, in general, represents gas bubbles that are uniformly distributed in a continuous liquid phase. These bubbles contribute greatly to the volume and density of several types of food, especially whipped cream, ice cream, bread, cakes, and mousses.1 They also play a major role in creating a sensory appeal to beverages such as carbonated water, beer, sparkling wine,2,3 and coffee4,5 by influencing their properties like mouthfeel, the release of flavors, and overall taste. However, foaming could also be undesirable depending on the unit operation involved, and product requirements. For manufacturing carbonated beverages or beer, aeration in the form of bubbles or foams is intentionally incorporated to bring about the desirable sensory profile;6–8 whereas, in the case of controlled fermentation systems, foam development is considered undesirable related to undesirable microbial growth.9 Since foams in foods have gotten consumer attention, they play an important role in the quality as well as the lifecycle of the food product.10
For the food foams to be produced at a larger scale in a controlled manner, exploring the behavior of different foaming agents, and understanding the foaming mechanism in the presence of different food ingredients are very important.11 In addition to foam generation, addressing the challenges of foam destabilization, and maintaining foam stability during product development is also equally important, and it has always been a research interest among food technologists. Through several reports over the years, the importance of proteins in food foam is well understood. Proteins by nature have both hydrophilic and hydrophobic groups in their structure; during foam formation, the former groups in protein molecules would orient towards the liquid phase while the latter groups orient towards the air phase. This way, protein molecules are incredibly useful in establishing the foam in liquid. In the food industry, natural proteins such as egg white, soy protein, casein, and whey protein are some of the main foam stabilizers.12
Over the years, egg protein has been commonly used for the preparation of a variety of food products owing to its unique functional and structural properties. Egg white protein (EWP), in its powder form, not only provides better functional benefits, such as better emulsification,13 foaming, and gelling properties,14 but also proves advantageous in shelf-life extension, storage, handling, and transportation compared to fresh eggs.15 In bakery operations, especially during cake batter preparation, EWP plays an important role in stabilizing the foam developed during the beating and mixing of the ingredients.16 EWP is an attractive foaming agent; however, its properties are dependent on various factors including the freshness of the egg, protein concentration, pH values of the egg, and other processing conditions of egg powder manufacturing.17 The functional properties exhibited by the proteins are greatly influenced by their structural properties; hence, modifying the proteins chemically or enzymatically would alter their structure, which in turn impacts their foaming behavior. The foaming characteristics of any protein depend on various factors including particle size, zeta potential, and surface hydrophobicity.18 Over the past decade, numerous studies have investigated modifications of egg protein through different treatments; among them, the effect of ultrasound on egg protein has been exclusively discussed in the current review.
Ultrasound treatment is utilized in food processing for numerous purposes including extraction, purification, and shelf-life extension. In general, the cavitation created during ultrasound treatment is the critical phenomenon that is responsible for effective mass transfer, free radical production, and other intensifying physical or chemical reactions on food materials.19 In the case of protein, this sonication results in the unfolding of protein structures that expose the hydrophobic groups to the surface further impacting the foaming characteristics of the protein.
There are various physical and chemical methods studied to modify the functional properties of egg proteins which are briefly discussed in the following sections. Among various methods, ultrasound technology is a simple yet effective nonthermal technique that can be used to treat egg white proteins to enhance their functional properties, especially foaming properties. Since the ultrasound could be applied to the egg solution without increasing the solution temperature, it has better scope in modifying protein and its functional properties. Also, ultrasound has been reported as a promising method to control foam in various systems including food, chemical, and pharmaceuticals.20 Although there are several studies on ultrasound treatment on egg protein impacting its foaming behavior, a detailed review of this subject will serve as a valuable resource. Keeping this in mind, various ultrasound factors such as treatment time, power, frequency, and amplitude that affect the foaming properties of egg proteins have been discussed in this review. In addition, ultrasound when combined with other treatments also has exhibited encouraging findings which have been discussed in this review. Unless mentioned, an egg in this article refers to a hen's egg.
2. Formation of foam
Foam is a two-phase colloidal system consisting of a large proportion of gas by volume dispersed in a liquid or solid or gel in the form of air bubbles.21,22 When viewed from a structural aspect, they are large numbers of bubbles packed to form an interconnected structure into films, nodes, or plateau borders.23 Foams have characteristic properties like low density, and large surface area as they display both solid and liquid-like properties.24 For foams to be stabilized, agents like surfactants are usually required, for egg foaming, the stability is attained natively through its protein molecules. The stabilizers help in slowing down the foam aging process. First, the liquid foams under the effect of gravity would drain rapidly until the liquid volume fraction reaches a limit. Second, the foams with gas transfer would evolve with coarsening and would coalesce with rupturing the films, separating the bubbles. In the case of foams with a higher liquid fraction, more than 50%, a continuous thin film is encouraged to stabilize the foams.25 The liquid bubbles ideally are dodecahedral shaped and equally sized separated by the thin film. And when three such thin films are intersecting, a plateau border is formed, as in Fig. 1. When the plateau border is smaller, drainage is faster in the film between smaller bubbles. The protein molecules, emulsifiers, and surfactants help in reducing drainage by decreasing the surface tension, thereby stabilizing the foams.26
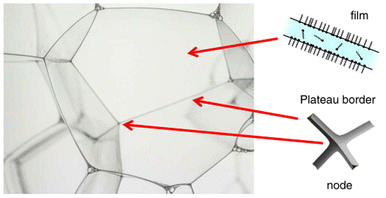 |
| Fig. 1 Liquid foams separated by thin film and formation of plateau border with the films.25 | |
Due to various factors, e.g. gravity-driven aging process, the collapse of bubbles is inevitable;27,28 therefore, the formed foams do not remain stable for a very long time. According to Wilson,29 three mechanisms namely disproportion, drainage, and coalescence are the responsible factors that might occur simultaneously or synergistically controlling foam stabilization. Among them, the disproportion is the major influencing factor that arises with the pressure difference between the bubbles. The pressure is mathematically expressed as Laplace pressure (ΔP) [eqn (1)]:
where,
γ and
R indicate the surface tension and radius of the bubbles, respectively.
The higher pressure inside smaller bubbles tries to diffuse through the liquid phase into the lower pressure inside large bubbles resulting in further expansion of large bubbles. This disproportion of bubble size makes the bubbles coarsen and destabilize. On the other hand, due to the density difference between the gas and water phases, under the influence of gravity, foam also undergoes water leakage. During this drainage process, the liquid film thickness between two bubbles reduces promoting coalescence between them.11
The nature and type of preparation methods, such as whipping apparatus or gas sparging, would also influence the foaming characteristics. Traditionally, foam could be produced through impellers, rotor–stator mixers, turbulence-creating baffles, or steam.33 In methods like whipping and mixing, vigorous agitation of the liquid with gas incorporates air into the liquid bulk. Due to increased turbulence created at higher impeller speeds the air trapped in liquid is often broken into smaller sizes. Similarly, higher whipping speed resulted in not just a higher foam-volume fraction but also a higher liquid fraction in the foam.34 Another common method of foam formation is the bubbling technique in which a nozzle or injector is used to introduce air into liquid bulk in the form of fine bubbles. Bubbling is better than whipping in terms of achieving better control over the size distribution of bubbles.26 The three major methods to assess air/gas that is incorporated in foods and beverages are quality parameters correlated with product appearance, foaming behavior, and gas-phase properties.6
Some of the important indices used to characterize foaming properties are foaming ability (FA), also called foaming capacity (FC), and foaming stability (FS). FA or FC indicates the ability to incorporate air in the protein solution, which is measured by foam volume and expressed as foam expansion at 0 min, mathematically as presented in eqn (2). Meanwhile, FS is the capacity for the foam to be held as such; this is measured by liquid drainage rate from the foam or decreasing foam volume rate, and expressed as a percentage of liquid still present in foam after 30 min eqn (3).35–37 Numerous studies have reported on the improvement of FS without compromising the FA or FC. Generally, FA is dependent on protein solubility, hydrophobicity, and tenderness of solution, while FS is influenced by protein concentration, hydration, and molecular interaction.38
| 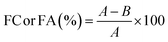 | (2) |
where
A and
B represent volume after whipping and volume after 4 min of whipping (in mL),
35 | 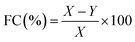 | (3) |
where
X and
Y are foam volume after 30 min standing and volume before 4 min of whipping (in mL).
35
Production of foams, in general, is not favored in any single-component pure liquid because the fluid films tend to rupture as soon as any mechanical or thermal perturbation occurs. Therefore, the foams are commonly stabilized using stabilizing agents like surfactants, proteins, or polymers.24 In surfactant-stabilized foams, surfactants that are amphiphilic in nature help in increasing the film stability at the interface. The surface tension phenomenon develops with an imbalance of intermolecular attractions at the liquid surface; meanwhile for creating a liquid–gas interface additional energy is required which contributes to the surface tension. At this point, surfactants reduce the surface tension without which the bulk liquid would be transformed from low surface area into high surface area foam.25 In protein-stabilized foams, proteins are required to be soluble in the liquid for foam formation. During protein adsorption at the liquid–gas interface, the hydrophobic ends are directed towards the gas phase as the protein molecules lose their tertiary structure to the secondary structure.39 The protein adsorption at the interface influences the foaming behavior of the system.40 In the case of particle-stabilized foams, the surface active colloidal particles are used that could get adsorbed spontaneously at liquid–gas, liquid–liquid interfaces.41 While surfactants reduce the surface tension of the system, particles reduce the total surface energy which stabilizes the system.42
Among the different types of foams, protein-stabilized foam is a common method used in the food industry as protein is a naturally occurring substance across numerous foods that encourages foaming. The role of protein in foaming is attributed to its unique structural properties with the presence of both hydrophilic and hydrophobic ends. Controlling the protein molecules' aggregation has played an important role in controlling the foaming properties of the protein.33 In general, protein foam characteristics depend on various intrinsic parameters – size, structure, protein charge, surface hydrophobicity, and other process parameters including protein concentration, and temperature inclusion of additives.26 In general, during whipping, when air comes in contact with the protein present in the solution, bubbles are formed at the surface of the solution, and denaturation of protein begins with partial unfolding followed by adsorption of air at the interface of hydrophobic groups of protein molecules. This altered structure results in either loss of solubility or protein precipitation at the liquid–air interface, which further decreases the overall surface tension. Partially unfolded proteins associate together to develop a stable film around the bubbles which contributes to the foam stability. On the other hand, excess unfolded protein molecules also produce highly unstable foams because of various factors including the presence of excess protein in solutions, the formation of a small bubble, and the reduction in bubble elasticity.30–32
3. Factors affecting foaming behavior of eggs
Egg white, thanks to its fantastic foaming ability, has numerous applications in food product development. EWPs have been widely used in the preparation of meringues, cakes, chocolate, mousse, whipped cream, and other leavened bakery products.34 Egg white can get adsorbed at the air-liquid interface due to its relatively high surface hydrophobicity. When discussing the components present in the eggs, there are various factors affecting egg production that in turn affects the composition of eggs. Some of the important factors are briefly discussed below:
3.1. Egg production factors
Various source factors control the overall composition and physical properties of eggs like breed of hen, age of hen, type of feed ration, breeding environment, season of egg harvesting, and egg storage period. These factors diversify the number of proteins, fat, and enzymes present in the egg that inturn impacts nutritional and functional properties. Here, some of such important egg production factors discussed that influence the foaming properties of eggs.
3.1.1. Season.
The freshness of eggs is usually measured with egg albumin and yolk index as the values are directly related to the quality of the eggs. Tomar et al. reported that during summer, albumin and yolk index reaches the lowest values while during winter the values are the highest.43 The decrease in freshness found in summer is due to the increase in internal temperatures for chicken that lead to lower feed consumption. In addition, with an increase in internal temperatures the digesting ability for protein, fat, and starch decrease among the chickens.44 Hence, the effect of seasons on egg composition could bring variation in functional properties.
3.1.2. Housing condition.
Tomar et al. also reported that under the same seasonal conditions, free-range grown poultry exhibited higher albumin (11.33%) and yolk index (51.75%) than cage-grown poultry (5.3% and 40.72%, respectively).43 Yenice et al., reported that backyard poultry has shown the highest albumin while free-range poultry has shown the lowest albumin content.45 But Vlčková et al. reported that the type of housing system (enriched/cage/free range) and hen's age (26, 51 week) did not have a significant effect on foaming durability and whipping indices.46 Alamprese et al., also suggested that although the best foaming properties, foam consistency index, and overrun were found for cage than barn eggs, the differences are small and might not have real effects.47 These results indicate that the housing conditions might not produce significantly different foaming properties.
3.1.3. Hen's age.
When eggs collected from hens, aged from 24 to 71 weeks, were studied, the results showed that foam overrun of thin albumin decreased with the increase in hens' age when stored at 4 °C for 14 days. While for foam stability, both thin and thick albumin showed an increase in values with hen age. They suggested that there is an ovomucin increase in both thick and thin albumin which might increase the protein available for adsorption at the surface.48 Silversides and Budgell reported that the whipping volume for whole albumin increased slightly with the hen's age when tested for three different varieties of chicken.49 With changes in albumin content in eggs obtained from different aged hens, the foaming properties are expected to differ with the hen's age.50
3.1.4. Storage time.
During storage of eggs, due to solvent loss in albumin there is a sharp decline in albumin weight irrespective of the storage temperature.51 In addition, the percentages of α-helix and β-sheet structures of ovalbumin decrease while percentages of β-turn and coil increase during storage. This influence the functional properties of the eggs that both emulsifying and foaming properties of OVA dropped in a study during the storge at 22 °C & 65% RH. The FA declined sharply from 35% to 4% while FS decreased from 17 to 13% within 50 days. This was due to the aggregation of protein molecules that led to a lesser explosion of hydrophobic amino acids.52 Alleoni and Antunes reported a positive correlation between s-ovalbumin content and liquid drainage volume. During storage, as the s-ovalbumin increased, the amount of albumin drainage increased, thus decreasing the FS drastically for egg white foam.53 Hence, it could be concluded that storage time has a major influence on the foaming properties of egg proteins. The differences in composition across chicken eggs are predictable due to various reasons like growing conditions, feed material, and temperature of the region.
3.2. Egg proteins
A whole egg is made up of egg white-58%, egg yolk-31%, and eggshell-11% by volume. The major component of egg white contains 88% of water, 10.5% protein, 0.5% carbohydrate, 0.8% ash, and 0.2% lipids while egg yolk contains 50% of water, 31 to 35% of lipids, 15 to 17% of proteins. Egg white, thanks to its fantastic foaming ability, has numerous applications in food product development. EWPs have been widely used in the preparation of meringues, cakes, chocolate, mousse, whipped cream, and other leavened bakery products.34 The proteins present in egg white contains essential amino acids, and possess high bioavailability which makes them a unique food processing ingredient with numerous functional benefits.54 Among different EWPs, ovalbumin, and ovostatin were found responsible for the excellent foaming capacity for egg white while ovomucoid and lysozyme were found closely related to foaming stability.55 The EWPs are briefly discussed below with respect to foam formation. EWP, in most of the literature, is freshly prepared from locally bought fresh eggs, unless mentioned.
3.2.1. Ovalbumin (OVA).
Among 140 proteins that are identified in chicken egg white, ovalbumin (OVA), the major protein with an isoelectric point (pI) of 4.5 contributes 54% of total protein and is the only protein that has free sulfhydryl (SH) groups which are largely responsible for the surface–active properties of EWPs. Egg white can get adsorbed at the air–liquid interface due to its relatively high surface hydrophobicity, hence it is the dominating protein responsible for foaming.56 Food products prepared to utilize the foamability of eggs are mostly attributed to the ability of albumin protein to encapsulate and hold air within food products.27 The susceptible nature of OVA to surface denaturation and interfacial coagulation are factors responsible for the foaming properties of EWP.57 Jin et al., reported that OVA under alkaline conditions showed improved structural stretching and polarity that acts as a driving force to unfold at the interface. The real-time dynamic structural analysis also revealed that it took just 50 ns for OVA to reorient over the air–water interface.58 Overall, it can be concluded that the presence of OVA plays a crucial role in foam formation.
3.2.2. Ovatransferrin (OVT).
The second most available protein in eggs is ovotransferrin (OVT), which is the most heat-sensitive protein and contributes 13% of total proteins. The OVT has very little to no foaming properties by itself although, its interaction with lysozyme encouraged excellent foam.53 Recently, Li et al., showed that OVT has a higher penetration and rearranging rate at the air–water interface at a specific pH such that it produced a highly elastic as well as stable interfacial membrane.59 Similarly, Hu et al., suggested that the molecular flexibility of OVT allowed structural expansion that enhanced natural interactions between hydrophobic groups for improved foam stability.60 Therefore, these results indicate that OVT helps more in foam stabilization than foam formation.
3.2.3. Ovomucoid.
Ovomucoid constitutes 11% of total EWPs. It is a glycoprotein and contributes to a large proportion of the total carbohydrates of eggs. Ovomucoid in itself cannot produce foam but could be combined with other proteins,61 including ovomucoid and lysozyme which resulted in excellent foaming stability.55
3.2.4. Ovomucin.
Ovomucin, contributing around 3% to total EWP, is particularly important in affecting the egg white viscosity, and it exists as a structured biopolymer network cross-linked with disulfide (SS) bridges. Ovomucin solution also cannot produce foam itself and would require other proteins. Instead, Nakamura and Sato suggest that it acts as a foam stabilizer than a foam generator.62 However the interaction between the lysozyme and ovomucin complex is also reported to be responsible for higher foaming stability in egg white.39,40
3.2.5. Lysozyme.
Lysozyme contributes to around 3.5% of total chicken EWP, which has gained significant attention recently regarding foam formation. Lysozyme which was earlier reported to have poor foaming properties than other egg proteins,35–37 has been recently proven useful and essential for better foaming properties of EWP, and the absence of lysozyme would only yield larger and sparser foams due to the absence of electrostatic interaction.38 The positively charged lysozyme plays an important role in foam formation and stabilization. The peptide bonds between lysozyme and ovomucin stretch out to the interface during whipping and produce a protective membrane that decreases drainage as well as coalescence of the foams thereby enhancing the foam stability.57
Understanding that the interaction among proteins plays a significant role, Jin et al. reported that the OVA when mixed with lysozyme in the ratio of 3
:
7 yielded maximum FC, whereas, mixing in the ratio of 5
:
5 yielded maximum FS.125 Similarly, Wang et al. also indicated that presence of ovomucin in EWP could play a potential role in EW-thermal gel (heat-induced gel) formation and that through sonication the ovomucin content can be increased in EW-thermal gel structure.126 Due to the versatile nature of EW-thermal gel, the applications of such gels are being explored in developing functional foods, food packaging, and biopharmaceuticals.43
Meanwhile, from an industrial point of view, the presence of egg yolk (EY) is considered contamination in EWP systems as they interfere with the functional benefits of EWP and can cause rancidity issues during storage. Although major attention is given to separating the EY components in industries, there is always an interest among scientists in understanding the functional properties of mixed systems. EY, which in general does not produce foam, was mixed in different proportions with EW to explore its effect on the foaming characteristics.44 With the addition of whole EY fractions (0–30 wt%) in EW, the FC decreased, while FS increased positively compared with EWP alone. On the other hand, the addition of 0–1 wt% EY in EWP solution was evaluated for foaming properties and its effect on final product (batters and cakes) quality.45 Interestingly, these results indicated that even little addition (0.1%) of EY could impart a negative effect on the foaming profile as well as final product quality. This was because of the increased competition for the air–water interface between EW and EY. After the EWP reached the maximum tolerant EY concentration, both the FA and FS values decreased with the further addition of EY. In general, higher solubility of protein is considered as the basis for improved foaming and emulsifying properties of the same, therefore, increased solubility of protein is encouraged to enhance the FA of any foaming compound. The next section discusses the techniques and additives used to enhance the FA of egg protein.
4. Different techniques and additives influencing egg foaming behavior
Egg white proteins under different structural conformations exhibit different functional properties. The degree of unfolding, and level of aggregation affect the digestibility, emulsifying property, foaming property, or gelling property. The control over the aggregation of EWPs can have a direct influence on its functionality.63 In addition, allergenicity to OVA among consumers is a concern that prevents utilizing the high-bioavailable protein resource. Various physical and chemical treatments modify the protein structures such that they help in the inclusion of the ingredient.64 Different thermal and non-thermal processing technologies have been shown to decrease the allergenicity of egg proteins.65 With the numerous applications of modified EWPs, numerous treatments have been proposed by scientists.
Physical methods involve thermal-heating or non-thermal treatments like high-pressure processing, ultrasound, irradiation, and pulsed electric field which might target one or more than one of the changes including the unfolding of protein structures, partial denaturation, breakage of disulphide bonds.36,66 Chemical methods include Maillard glycosylation, ion modification, enzyme addition, and polysaccharides addition.67 Discussing the effect of different treatments on all the functional properties EWPs is out of the scope of this article, hence discussion is written to emphasize the effects on egg foaming.
The addition of hydrocolloids to EWP alters its foaming properties as they form complexes with the protein molecules.22 Reportedly, the addition of xanthan gum or carrageenan exhibited poor processability or poor stability, while the combination of the two hydrocolloids yielded stable foams with unique functional properties. In addition, different complex/compounds such as caffeic acid,68 basil seed gum,69,70 catechin-chlorogenic acid,71 gum–arabic complex,72,73 xantham gum,74 and pre-heated CaCl2 (ref. 75) have also been reported to increase either the hydrophobic interactions or hydrogen bonding that helped in improving the foaming profile of the complex system. Similarly, EWP modified with soy peptides enhanced the FC while the addition of sucrose to the modified proteins increased the FS.76 The modified EWP when incorporated into the cake yielded the best quality and maximum volume. Moreover, synergetic effects of more than one treatment, like hydrolysis along with glycosylation,77 and hydrolysis combined with phosphorylation,78 have also been reported to influence the foaming properties of OVA. Recently, Jia et al.79 reported a positive influence of Lactobacillus fermentation on the foaming profile of EWP, especially OVA, ovomucin, and ovomucoid. They observed that during fermentation, disulfide bonds of protein molecules were broken along with the expansion of the air–water interface which helped in improving overall surface–active properties. However, the chemical treatments can leave a chemical residue that might lead to adverse effects and reactions in egg white.80
Recently, different types of enzymes such as lipase, phospholipase, and protease were used to treat liquid OVA during the preparation of meringue's batter, and the results indicated that lipase-treated liquid egg exhibited better stability, batter yield, functionality, and overall acceptability for meringue compared to the other enzymes.81 In another study, a mixture containing EWP and EY was enzymatically treated separately with lipase and phospholipase to compare their effect on foaming properties;17 where phospholipase was found to be more effective than lipase in improving the foaming profile of EWP, and it was also indicated that the presence of EY plasma could be the major reason for poor foamability of the EWP-EY mixture. Although the enzymatic treatment has been studied to improve the foaming properties of EWPs, the time taken for the treatment is high and might result in producing bitter peptides.80
During the heating of EW with high temperatures (30 to 60 °C) for 10 min both the FC and FS were enhanced, with maximum enhancement at 50 °C; however, the FA and FS values dropped when the temperature was increased further.82 With a temperature increase from 25° to 50 °C, the surface hydrophobicity increased with a decrease in interfacial tension improving foaming properties. But beyond 50 °C, the protein molecules aggregated and decreased the available hydrophobic groups from interface exposure. Also, higher temperatures may lead to increased protein denaturation, and decreased protein solubility that reduces the foaming activity.83 Hence controlling the temperature-sensitive proteins from denaturation is challenging in conventional heating methods.
On the other hand, the non-thermal technologies had better control over the increasing rate of temperature and helped in avoiding excessive denaturation in EWPs. During supercritical carbon dioxide treatment of 9 MPa & 45 °C on EWPs, Ding et al., found that the FA increased by 107% after 60 min of processing time, while FS was maintained for 75 min of processing time.80 The treatment allowed the protein molecules to rearrange and spread easily at the air-gas interface increasing the foaming capacity but the treatment also destroyed the α-helical structures of protein and particle size became uneven.
Pulsed electric field at 25–30 kV cm−1 when subjected to EWP solution for 600–800 μs produced insoluble aggregates resulting in protein solubility loss.84–86 The loss in protein solubility creates turbidity and is undesirable for foam formation. High-pressure processing of EWPs resulted in protein molecules aggregation for a shorter period of processing time.87 The aggregates led to changes in color and viscosity to the protein solution which are not suitable for foam formation.88,89 Ozonation, a non-thermal technique, is another approach to improving the interfacial properties of protein solutions. Ozone, owing to its high oxidative potential, has been studied for altering the protein structures in enzymes90 and whey protein isolate.91 In the case of ultraviolet radiation, radiation (UV-C) of 24 W m−2 induced lower level protein unfolding in egg white solution than pasteurized solution (56 °C).92 Among all the EWP, lysozyme was found to be the most sensitive to UV radiation while OVA was photostable to the radiation. Kuan et al., reported that UV-treated EWP had better emulsifying and foaming properties as the treatment allowed cross-linking of proteins.93 But the susceptibility of EWP to the UV light caused breakage of SS bonds that could lead to fragmentation or aggregation.
Ding et al.94 compared different physical treatments including supercritical carbon dioxide extraction, ultrasound, heat, and high-pressure homogenization on EWP.94 The authors reported that FC values increased in different treatments-for supercritical carbon dioxide extraction at 9 MPa it increased by 3.6 fold, for ultrasound treatment at 360 W it increased by 3.22 fold, for heating to 45 °C it increased by 3.51, and for homogenization at 600 bar, it increased by 2.9 fold with respect to control-untreated EWP was found respectively. But in case of FS, all the treatments exhibited lower values in a similar range than the control that supercritical carbon dioxide, ultrasound, and homogenization were suggested to be effective methods for improving the foaming properties of EWP. The findings indicated that ultrasound treatment produced more stable and uniform-sized bubbles than other non-thermal treatments. But it is interesting to note that the zeta potential values indicated that for all the treatments, the values decreased whereas for ultrasound alone there was no change in values as the power increased from 120 W to 480 W. It was suggested that the ultrasound treatment has the potential to alter the hydrophobic bond and free sulfhydryl groups without changing the electrostatic repulsion between the protein molecules. With lower operational costs and simpler equipment, ultrasound can be preferred over other treatments for enhancing the foaming profile of EWPs. In addition, it is a green technology that involves minimal to no impact of hazardous chemicals or emissions,95 leaves no residue, and does not require sophisticated equipment for operation. With all these advantages, the application of ultrasound for EWPs and its impact on foaming behavior will be summarized in detail in the following sections. Although there are many ultrasound studies on EWPs being reported at this point, there is no review that is available covering its effect on the foaming behavior of the egg proteins.
5. Effect of ultrasound treatment on egg foaming
The ultrasound technology uses high energy mechanical waves at 20–100 kHz that create generation and collapse of bubbles or cavities. These cavities bring vibrations to the protein structures which inturn affect the foaming properties.96 These vibrations are capable of changing the aggregation of substances by dispersion or emulsification that might modify the diffusion rate, crystallization rate, or solubility.97 Ultrasound has been studied to enhance FC for other proteins such as whey protein,98,99 soybean protein isolate.100,101
Due to the temperature-sensitive nature of the protein, ultrasound has been studied extensively at various power and amplitude levels to understand the effect of the treatment on the foaming behavior of whole eggs and EW. The application of ultrasound impacting protein characteristics has been useful in different applications, such as reducing the turbidity of whey protein suspensions while developing food with specific functional properties.102 Usually after sonication, protein molecules get evenly dispersed increasing the exposure area for foam formation. When high-intensity ultrasound, in the range of 20–100 kHz, was introduced to protein solutions, the protein unfolding enhanced the foaming without changes in peptide profiles.96 In another study, high-intensity (>20 kHz) ultrasound treatment of EWP exhibited disruption in the covalent bonds (peptide and SS bonds) and lysozyme-ovomucin electrostatic complex, resulting in degradation of the fibrillar network of ovomucin, which reduced particle size and increased both the solubility and flexibility for EWPs. These small particles then diffused and got adsorbed at the interface of the EWP solution improving the foamability.36 Thus, depending on the nature of protein and ultrasound parameters, the impact of the treatment on protein structure would vary.103 During a study of the effect of ultrasound on the egg–sugar mixture used for the preparation of sponge cake dough, Myroshnyk97 observed that the treatment had a positive influence on FC, FS, and foam dispersion of the egg–sugar mixture. In addition, it was also found that the ultrasound treatment reduced the whipping duration of the egg–sugar mixture by almost two folds which allowed simultaneous whipping of all components, thereby reducing labor intensity.
The ultrasound treatment of 20 kHz for 2–20 min on EWP created a partial unfolding of protein molecules and also disrupted the ovomucin fibrillar network. This is due to the disturbances of covalent bonds and ovomucin–lysozyme complex upon cavitation. The degraded ovomucin tends to move towards the interface and could get adsorbed increasing the solubility, surface hydrophobicity, and overall foaming capacity. At the same time, since ovomucin which helps in foam stabilization gets degraded, the FS value declines with a reduction in viscosity.35,104 Sometimes, as the diminution force increases the degradation of ovomucin network also increases, which produces smaller fibrils.105,106 There are also studies that reported the aggregation of protein molecules when subjected to ultrasound treatment which resulted in particle size increase and decrease in solubility. These effects are undesirable for foam formation in EWP solution.107,108
Xiong et al.,109 suggested that under sonication, formation of molten globule-like OVA structures are formed which reduce the barrier electrostatic energy required for adsorption thus favoring enhanced solubility and foamability. The shelled eggs when given ultrasound treatment helped in sealing the pores preventing carbon dioxide loss.110 Thus, the treated eggs during storage maintained pH and viscosity than untreated eggs. Overall, it is important to consider the different factors that can affect the foaming properties of EWPs when subjected to ultrasound treatment. In the following section, different factors of EWPs that are responsible for foam formation, as well as different ultrasound treatment parameters affecting foaming properties, are discussed.
6. Impact of ultrasound on different factors associated with protein
Apart from the nature and type of eggs, some of the factors including processing temperature, viscosity, particle size, zeta potential, and surface hydrophobicity of the egg solution can influence the foaming behavior of the solution. The following section discusses the effect of factors on the foaming ability and foaming stability of EWPs solutions.
6.1. Particle size
The particle size of the protein could be directly linked with the foaming properties. During ultrasound treatment, the cavitation force is responsible for breaking the structures of protein molecules into small assemblies of protein particles with OVA and/or lysozyme.78,79 Researchers indicated that ultrasonic-induced cavitation results in high levels of hydrodynamic shear and turbulence within the protein molecules. This disrupts hydrophobic and electrostatic interactions, and even among the hydrogen bonding which is responsible for maintaining the protein aggregates. A 20 kHz frequency and 95% amplitude of sonication were reported to decrease the particle size of EWP from 8.2 nm to 5.8 nm. However, after a week of storage, the size of treated EWP was found to be increased with reorganization and aggregation of protein molecules because of the re-established non-covalent interactions.79,80 Likewise, Yu et al.127 found that the ultrasonic treatment decreased the particle size of liquid EW from 259.43 ± 38.5 nm to 115.83 ± 5.79 nm, and reported the reason as decrease in the particle size of OVA and lysozyme. However, because of the formation of soluble aggregates, the particle size of OVT was found to increase after the ultrasound treatment.82
Treatment of high-intensity ultrasound (20 kHz) on EWP exhibited a two-step linear correlation between FC, FS, and particle size. FC and FS values increased for a decreasing particle size range of ∼370–260 nm, although no significant difference was observed for a particle size range of 260–68 nm suggesting particle size played a critical role in foaming properties (Fig. 2).104 In another study, the ultrasound treatment of 0.6 kW on egg–sugar whipping established identical small-sized bubbles closely located to each other, which protected the bubbles from coalescence thus increasing the FS than the untreated samples.74 Overall, the ultrasound treatment on egg proteins reduces the particle size of the proteins, although the effect is not significant over a smaller range of particles, which has a positive effect on both FC and FS values.
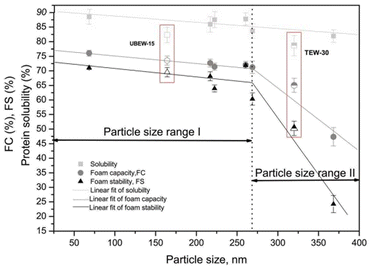 |
| Fig. 2 Change in solubility, foam capacity (FC), and foam stability (FS) during sonication of egg white, as a function of average particle size.104 | |
In general, the particle size of protein molecules is reduced after ultrasound treatment which is easily migrated to the air–water interface such that the hydrophobic groups are allowed to expose for foam formation.
6.2. Zeta potential
Zeta potential refers to the electrokinetic potential of a solution near a solid–liquid interface.84 A direct linear correlation was observed between FC, FS and zeta potential after sonication of EWP for different periods (Fig. 3). With increasing time of treatment, the zeta potential (negatively charged because of amino acids' presence) values increased indicating the retention of electrokinetic potential and stability of EWP throughout the ultrasound treatment. The increasing zeta potential represented the inter-particle electrostatic repulsions that disrupted available protein agglomerates, which prevented further agglomeration. These factors helped maintain the stability of EWP solutions during sonication;104 when ultrasound treatment was applied to shelled whole eggs, it decreased the absolute zeta potential of EWP which was reportedly responsible for lowering the FS after treatment.85 In another study, sonication of EW at 40 kHz, 300 W for 60 min, increased the FC by 25% while FS was decreased, which was attributed to the changes in the zeta potential of EW solution after treatment.86
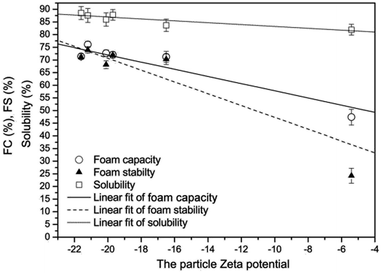 |
| Fig. 3 Linear correlation of foam capacity (FC) and foam stability (FS) and zeta potential of egg white after ultrasound treatment.104 | |
When low-density lipoprotein (LDL) obtained from EW was treated with 200 W of sonication for 10 min, the absolute zeta potential of both E-LDL and U-LDL were increased by 184.57% and 119.56%, respectively, and surface hydrophobicity was increased up to 10.81% owing to the protein unfolding.87 During sonication, physical alterations of the protein structures exposed the hydrophilic groups that increased the FC of the protein system. Overall, the ultrasound treatment, as a result of protein molecules unfolding, increases the zeta potential of the egg protein-based solutions, which has a linear relation with the foaming capacity and foaming ability.
6.3. Surface hydrophobicity and presence of free sulfhydryl groups
Surface hydrophobicity can be estimated from the initial slope value obtained from the plot of fluorescence intensity as the function of protein concentration. The effect of surface hydrophobicity on FA is contradictory. Though reports have proved that increase in surface hydrophobicity results in enhanced FA [84], Townsend and Nakai128 found that surface hydrophobicity has no significant effect on the FA of different untreated proteins. Moreover, the effect of ultrasound on surface hydrophobicity exhibited dissimilar results in different experiments. When EWP was treated with 180 W of ultrasound for 25 min, the free sulfhydryl content increased along with hydrophobicity that resulted in easier adsorption of protein at the air–water interface, and thus 2.3 fold increase in FA was obtained in cold-stored eggs compared to untreated cold stored eggs.85 Similarly, O'Sullivan et al.129 also reported an increase in hydrophobicity in EWP after ultrasound treatment of 20 kHz, 95% amplitude. On the other side, during a high-intensity (0–360 W) ultrasound treatment of EW solution, Sheng et al.130 proved that the free sulfhydryl groups associated with ovomucin decreased, which was attributed to the reduction in the FS value. Meanwhile, there are also reports explaining that the increase in hydrophobicity after high-intensity ultrasound treatment has little effect on the foaming behavior of EW solutions.89 When solution made from frozen EWP was treated with high-intensity ultrasound of 20 kHz and 60% amplitude for 6 min, the FC increased more than fresh eggs. The FC value became twice for the 21 day stored frozen EWP after the ultrasound treatment which was majorly due to the increase in surface hydrophobicity.111 Therefore, the effect of ultrasound treatment on the surface hydrophobicity of proteins, and its effect on foaming behavior depend on the treatment parameters, and more detailed research is warranted.
6.4. Viscosity
The viscosity of the solution is another factor that is expected to significantly influence the FS of egg protein solutions. Delahaije et al.131 reported poor FS, in terms of foam half-life (t1/2), for lower concentration (0.1–0.5 g L−1) of OVA (in phosphate buffer, pH 7). With an increase in OVA concentration from 5 to 10 g L−1, there was an increase in FS until a maximum point. However, higher concentrations (>5–10 g L−1) of OVA decreased the FS value. The study reported the positive effect of lower concentration on FS was due to the increase in the interfacial coverage during foaming, while the effect of higher concentration on FS was not fully understood. For the same concentration of OVA, with the increase in temperature, the t1/2 of OVA foams decreased indicating poor FS (Fig. 4A). Similarly, t4/5, which represents the time taken for 80% volume degradation of foam, showed that irrespective of the temperature, high OVA concentration (>5–10 g L−1) yielded very poor stability (Fig. 4B).
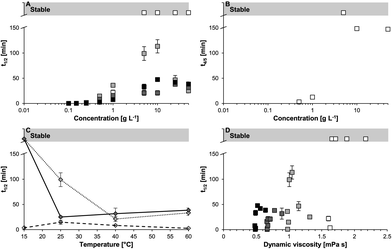 |
| Fig. 4 Relation between foam half-life time (t1/2) and various parameters like concentration (A), temperature (C), viscosity (D) at different temperatures 15 °C ( ), 25 °C ( ), 45 °C ( ), 60 °C ( ). Plot between (t4/5) and concentration (B) of OVA solutions.131 | |
Arzeni et al. reported that the high-intensity ultrasound treatment of 20 kHz, 20% amplitude at 4.27 W on EW solution decreased the apparent viscosity (by 13%), which in turn negatively affected foaming properties.108 In another study, ultrasound treatment during sponge cake preparation increased the viscosity of the egg–sugar mixture influencing the ductility of films that allowed more volume in the air phase in the system. Although the film thickness was observed to decrease, the film strength was found to increase, which was evident through higher FS values after the ultrasound treatment. In addition, in the final product, the porosity was 7.5% higher for the ultrasound-treated mixture compared to the untreated one, which helped in enhancing the overall sensory-to-quality of the final product.74 Therefore, according to the nature of the egg solution, the ultrasound treatment can either increase or decrease the viscosity of the solution. If the treatment induces protein aggregation, then the viscosity increases otherwise the viscosity is expected to decrease for the EWPs solution after sonication.
6.5. Temperature
For the formation of foam, the temperature is considered to be an important parameter as it influences the stabilization and denaturation of the protein molecules. A study reported enhanced FA for OVA when the temperature was increased, but the effect was countered with a reduction in FS.90 For different concentrations (>0.5 g L−1) of OVA, t1/2 of foam was found to decrease rapidly with an increase in temperature (>40 °C), which represents the poor stabilization of foam with higher temperatures (Fig. 4C). Thermal processing, such as pasteurization and spray drying exhibited enhanced (35%) FC of EW owing to high zeta potential and surface hydrophobicity in spray dried EW.91 During the thermal treatment of EW peptides at 90 °C for 30 min followed by ultrasonication of 800 W at 20 kHz and 30% amplitude for 15 min, the peptides exhibited enhanced emulsion stability along with enhanced ionic, thermal and storage stabilities.92 As a result of this combined treatment of heating and sonication, the hydrophobic group distribution over the protein surface was improved and the surface tension was reduced to enhance the surface activity of the peptides present in the EW solution. All these parameters greatly influenced the foaming properties. In another study, when heat aggregation kinetics was plotted for EW solutions treated with high-intensity ultrasound (200 W power), the Arrhenius equation-fitted plot expressed that the treated solutions exhibited lower aggregation rate dependency on temperature than control (untreated sample) over 85 to 70 °C, and the activation energy Ea was found much higher than that of the control (Fig. 5).108
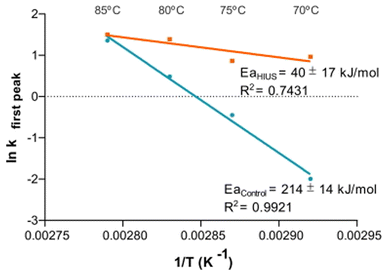 |
| Fig. 5 Aggregation kinetics for EW solutions before (blue line) and after (orange line) high intensity ultrasound treatment heated to 70, 75, 80, and 85 °C for different times.108 | |
During ultrasound treatment of shelled eggs at 180 W for 25 min and subsequent cold storage at 4 °C, the foaming properties of EW were maintained even after 60 days of storage. FA was recorded maximum (99.13%) at 60 days of cold storage, which was around 2.3-fold increased than the untreated EW (29.75%); although FS was decreased after the treatment.85 Overall, the temperature factor is critical for FS, as the FA is generally enhanced with increasing temperatures for egg solutions but the temperature control is essential in maintaining the degree of denaturation of proteins. Because the denatured protein molecules can result in aggregation of molecules which is not suitable for foam formation.
6.6. Oxidation
The degree of oxidation the EWP undergoes also plays an important role in altering protein structure thus foaming behavior. Oxidation induced by 2,2- azobis (2-amidinopropane) dihydrochloride (AAPH) showed improved FA (from 85.6% to 91.4%) and FS (from 80.6% to 85.3%) of EWP, even at a low concentration (0.2 mM) of AAPH. With excessive oxidation, from higher AAPH concentrations of 5 and 25 mmol L−1, the FA values increased but it degraded the FS values of the protein solution. During oxidation, the protein structure was found to be modified with an increase in its surface hydrophobicity, encouraging better FA and FS values although excessive oxidation allowed the proteins to get aggregated, which did not help in improving FS.93 The oxidation treatment (AAPH at 0.2 mM) on EWPS induces interchange between sulfhydryl and disulfide network. This enhances the surface hydrophobicity thereby improving the FC values. Although excessive oxidation (5 and 25 mM) increased FC values, the FS values dropped for the EWP solution.112
7. Effect of ultrasound parameters on egg foaming
During sonication, various process parameters influence the treatment effect on a sample that in turn impacts the functional properties of egg proteins. The summary of the sonication factors influencing foaming properties is described in Table 1.
Table 1 Overview of ultrasound parameters and their effect on foaming propertiesa
S. No. |
Ultrasound factor |
Sample |
Condition |
Effect on foaming properties |
Ref. |
EWP-egg white protein, OVA-ovalbumin, FC-foaming capacity, FS-foaming stability.
|
1. |
Frequency |
EWP |
Single frequency-20 to 40 kHz |
FC did not increase with frequency. FS also did not increase with frequency |
Jun et al., (2020)132 |
Dual frequency- 20/28 and 20/40 kHz |
FC did not change at dual freq. conditions. FS values were significantly higher at 20/48 kHz frequency. |
Jun et al., (2020)132 |
2. |
Power |
Egg yolk |
0 to 300 W |
FC increased with power. FS decreased with power. |
Xie et al., (2020)117 |
270 W |
Increased zeta potential and decreased sulfhydryl groups. |
Geng et al., (2021)118 |
EWP |
120 to 360 W |
FC increased with power |
Ding et al., (2022)94 |
3. |
Amplitude |
OVA |
0, 60, 90% |
FC increased with amplitude. No significant changes in FS |
Xiong et al., (2016)109 |
Egg white |
20% to 40% |
Decrease in foam overrun |
Arzeni et al., (2012)108 |
4. |
Time |
EWP-OVA |
15 min |
FC and FS reached the maximum at 15 min but both values decreased beyond 15 min |
Stefanović et al., (2017)104 |
Egg white |
30, 45, 60 min |
FC did not have significant effect. FS decreased. |
Nagy et al., (2021)116 |
7.1. Frequency
Ultrasound in the range of 20–4520 kHz was experimented with for foam fractionation in bulk aqueous solutions.113 The results indicated that only 20 kHz ultrasound produced finer foams with enhanced separation of dissolved surface-active species from bulk aqueous solutions; it also helped in removing around 125–320% surfactants and bioactive agents from the experimented bulk solution. Thus in most of the sonication studies, 20 kHz was used to modify the EWPs solution. Further, Lei et al.114 found that exposure to high-frequency ultrasound of 60 kHz for more than 4 min altered the structure of OVT by exposing the hydrophobic groups, and generated 50% more reactive sulphydryl groups in 5% of OVT which is not suitable for processing and food applications.
Recently, high-power single and dual frequency type ultrasound was experimented with to study its effect on the functional properties of EWP.115 An increase in both FC and FS of EWP was observed by the sonication treatment at single (40 kHz) frequency, and 20/28 and 20/40 kHz dual frequency (Fig. 6). In addition, in this study, the FC was reported to be dependent on ultrasound frequency, time, and temperature unlike FS, which was not significantly dependent on the mentioned parameters. During treatment of the proteins for 10 to 60 min, initially, an increase in FC was observed owing to the protein unfolding but later it decreased owing to the thermally induced protein aggregation. With a greater decrease in particle size than the control, the 20/40 dual frequency treated EWP could hold more water molecules in their gel structure after treatment. With all these positive results, the authors suggested that this high-power continuous dual frequency ultrasound exhibiting sonochemical effect could be the green technology for improving the various functional and physicochemical properties of EWP.
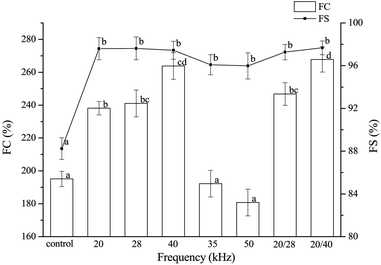 |
| Fig. 6 Effect of ultrasound frequency on foaming properties of egg white proteins; FC and FS represent foam capacity and foam stability, respectively.115 | |
7.2. Power
The power of sonication reportedly has a significant role in the foaming properties of EW.116 In general, the sonication power influences the foaming properties by creating more cavitation that increases in absolute zeta potential value and sulfhydryl values. EY protein treated by ultrasound exhibited an increase of FA with increasing sonication power from 0 to 300 W, while FS values decreased for the same.117 As observed from Fig. 7A, the maximum FA of 125% was observed for 300 W power, which was 2.1 times higher than the control (without sonication) which had an FA of only 60%. At the same time, FS of EY protein was found to be maximum (78.3%) in control and minimum (51%) at 300 W sonication, which contributed almost 34.9% of reduction (Fig. 7B).
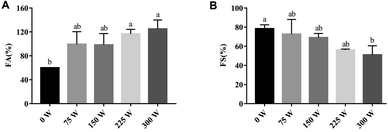 |
| Fig. 7 Effect of increasing power values of high-intensity ultrasound on egg yolk; FC (indicated as foaming ability in (A)) and FS (B) represent foam capacity and foam stability, respectively.117 | |
In another experiment, EY was subjected to a high-intensity ultrasound of 270 W power, and the results demonstrated evidence of depolymerization of EY granules that reduced the average particle size from 289.4 to 181.4 nm. Further, the sonication decreased the free sulfhydryl group and increased the absolute zeta potential influencing FA and FS.118 Similarly, for the EWP solution, the FC values after sonication were found to be increasing with increasing power from 120 to 360 W and reached a maximum from 96.5% to 260%.94 In another study, with an increase in power from 180 to 300 W, the FC of EW increased owing to the increased homogenizing effect and exposure of the hydrophobic groups of the protein molecules.94 Although in this study, frequency, and duration of treatment did not significantly influence the foaming properties of EW solution. However, at the power level beyond 360 W, the FC value was found to decline, which indicated that high power of ultrasound is not recommended for enhancing the FC values of EW.94 Therefore, with increasing power values, FA is found to increase while FS is not.
7.3. Amplitude
Amplitude is an important parameter for ultrasound treatment as it influences the cavitation produced during sonication. Upon subjecting OVA to a 20 kHz ultrasound at varying amplitude levels of 0, 60, and 90% for 20 and 40 min, FA of the protein was found to increase with increasing amplitude, whereas, no significant difference was found in FS among all treatments. With this high-intensity sonication treatment, the surface hydrophobicity increased with the unfolding of tertiary structure and a decrease in net surface charge, which helped in the improvement of overall emulsifying and foaming properties.109 Sonication of EW at 20% of amplitude (maximum net power of 750 W) in a high-intensity probe ultrasound system operated at the frequency of 20 kHz for 20 min resulted in decreased foaming overrun (from 205% to 127%) with an increase in amplitude.108 From the plot of foam drainage kinetics, it was understood that the foam drainage rate constant for treated solutions increased about 10 folds, while drainage t1/2 decreased up to 10 times in comparison with untreated EW solution. The increased foam drainage rate constant indicates the poor foam stabilization of egg solutions with increased amplitude.
7.4. Time
For every process, the treatment duration plays an important role in product quality. Reportedly, there is a positive correlation between the exposure time of ultrasound and FS and FC values of EWP. Even 2 min of sonication treatment of 20 kHz and 40% amplitude increased FC up to 1.5 times of the control; although, beyond that, no rapid increase was observed.104 During the treatment, 15 min of sonication displayed a maximum FC of 60.6%, and FS of 193.3% whereas the control had FC of 47% and FS of 25%. Until 15 min of treatment, the particle size of OVA decreased, but later, with time, the size was found to increase, possibly owing to the aggregation of protein molecules which is responsible for the drop in FC values. From Fig. 8 it can be understood that increasing treatment time plays a more significant role for FS compared to FC of EWP solutions.
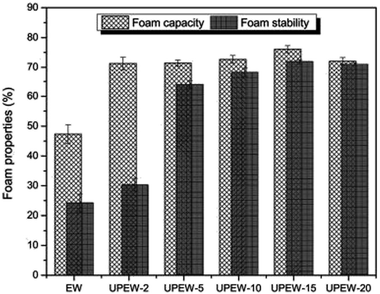 |
| Fig. 8 Effect of ultrasound treatment time on foam capacity and foam stability of egg white, where UPEW-2 to UPEW-20 indicates ultrasound treatment of egg white for 2, 5, 10, 15 and 20 min, respectively.104 | |
In contrast to the above findings, in different research, FS values decreased while no effect of time on FA was observed with increased sonication time from 30, 45, and 60 min for EW at 20/40 kHz, 180/300 W power.116 It was suggested that in the post-ultrasound treatment, the reduction in the potential difference between the dispersed medium and dispersed phase was responsible for foam structure collapse.
8. Ultrasound-assisted treatments influencing foaming properties of egg white proteins
8.1. Ultrasound-assisted irradiation
Ultrasound as a pretreatment method has also been examined to enhance the foaming properties of proteins. When liquid EW was treated with ultrasound (300 W) for 6 min along with 33 kGy of irradiation dose, the FA increased up to 92.6% owing to the decrease in particle size of proteins (OVA and lysozyme) and increase in protein solubility.81 It was reported that post-treatment, the amount of α-helix and β-sheet in proteins such as OVA, OVT and lysozyme were found to be decreased. The ordered polypeptide chain structure was broken into secondary structures which would impact foaming properties.
8.2. Ultrasound-assisted hydrolysis
Hydrolysis of protein in general refers to a chemical reaction involving the splitting of protein molecules into amino acids in the presence of enzymes. When ultrasound was applied as a pretreatment before hydrolysis of EWP, it was found that the hydrolysis rate was increased by 139.8% than the control (hydrolyzed without ultrasound treatment). After optimal ultrasound treatment of 21.3 W and 40 kHz frequency for 15 min at 25 °C, EWP was hydrolyzed with alcalase and neutrase under a two-stage enzymatic process to produce better voluminous foam.96 Treating the liquid whole egg with ultrasound at 605 W cm−2 for 30 min at 35 °C decreased the FC and FS to 19.45% and 3.74%, respectively; whereas, ultrasound along with lysosome hydrolysis increased the values of FC and FS to 21.14% and 9.3%, respectively.119 Despite that, the ultrasound and lysosome treatment inactivated S. typhimurium and the inactivation efficiency increased with ultrasound power and time. Therefore, sonication could be a useful pretreatment for hydrolysis in improving the rate of hydrolysis and microbial inactivation.
8.3. Ultrasound-assisted freezing
In a study, two types of freeze drying, conventional freeze drying (CFD) and pulsed spouted magnetic freeze drying (PSMFD) were compared to produce fresh and desalted duck EW powder with prior ultrasound treatment.95 The method of freeze drying indeed affected the FA or FC and FS of EW. For fresh duck EW, FA was found to be higher in CFD than PSMFD, whereas FS was higher in PSMFD than CFD. Under PSMFD, FS value increased after ultrasound pre-treatment due to the increase in surface charge and surface hydrophobicity of proteins, while FA remained to be unaffected after treatment.
8.4. Ultrasound-assisted glycation
Glycation, a unique process utilizing the Maillard reaction, allows conjugation of the amino group of protein and the carbonyl group of sugar molecules through covalent bonding such that both the exposure of the hydrophobic part of protein molecules and unfolding are increased resulting in faster adsorption at the air/water interface.96 In various studies, the Maillard reaction is proven to enhance some of the functional properties of proteins like emulsifying capacity, foaming ability, gelling ability and solubility.97 In addition, glycation products are found to exhibit good antioxidant properties.98,99 The changes during glycation, apart from imparting distinct flavor to the product, also enhance the foaming profile of the protein system.100,101 The application of ultrasound in decreasing the processing time of Maillard reactions was reported, in which the protein–sugar conjugates displayed better properties like solubility, FC and FS.102–105
In general, saccharides play an important role in the formation and stabilization of egg foams. Recently, Sun et al. established the relationship between saccharides and rheological, interfacial, and foaming characteristics of EW solution. It was observed that higher maltodextrin (3%) increased both the FC and FS values because of increased surface hydrophobicity, and reduced surface tension.133 Recently, ultrasound-pretreated OVT indicated highly flexible and looser tertiary structure that showed improved biological activity against E. coli and S. aureus.107 Ultrasound-assisted glycation of OVA with xylose enhanced the FA and FS with increasing sonication time compared to native OVA molecules (Fig. 8).108 At 50 min of treatment time, sonicated and glycated treatment possessed the highest FA, which was around 2.5 folds higher than that of native OVA. The sonication treatment resulted in increased flexible and loose protein formation while the glycation provided additional hydrophilic groups for protein molecules to interact, which increased the surface hydrophobicity overall, enhancing both the FA and FS%. In comparison with untreated OVA, both sonicated and sonication-assisted glycated OVA expressed superior foaming characteristics.
Researchers have identified the correlation between the degree of glycation with xylose and the functional properties of OVA protein that were influenced during the glycation treatment.109 It was reported that most of the surface properties including emulsifying properties and foaming properties are strongly correlated with the degree of glycation. For OVA, FA was positively correlated with the degree of glycation whereas FS was negatively related. This indicated that xylose-OVA conjugates had undergone unfolding and conformational changes that allowed the conjugates to get adsorbed quickly at the oil–water/air–water interface elevating the FA of OVA. Meanwhile, after sonication was introduced along with glycation, the combined treatment produced higher FA and FS than individual glycated and sonicated OVA molecules. This is owing to the generation of ultrasound cavitation that enhanced the mobility and hydrophobic groups (Fig. 9). Sonication made protein molecules more susceptible to conjugation and increased the probability of collision of interactive groups that improved the degree of glycation simultaneously. Importantly, the sonicated and glycated OVA did not significantly differ in its digestibility value under simulated gastric and intestinal conditions compared to native OVA.110 Likewise, the effect of short-chain xylooligosaccharides (XOS) glycosylation with EWP resulted in enhanced foaming properties for the conjugate than that of EWP alone.111 The increase in sugar content was positively correlated with foaming properties of EWP; for the addition of 5% XOS up to 1 and 1.2-fold, and for 10% addition 1.5 and 1.7-fold increment of FA and FS were observed, respectively , refer to Fig. 10.
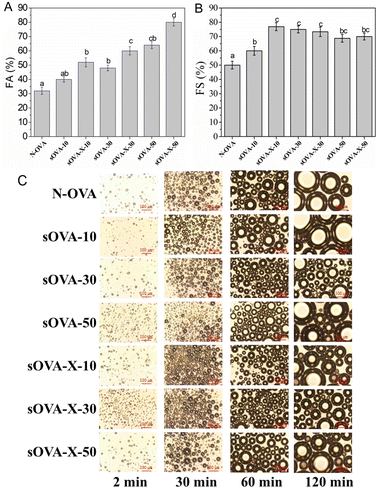 |
| Fig. 9 Effect of ultrasound-assisted glycation treatment on foaming ability (FA) (A), foaming stability (FS) (B), and foam bubbles (C) of ovalbumin (OVA) after different treatments.120 | |
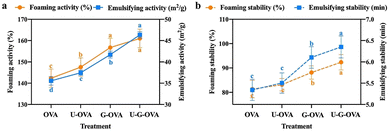 |
| Fig. 10 Effect of different treatments on foaming activity (a) and foaming stability (b) of OVA molecules including ultrasound (U-OVA), glycation with xylose (G-OVA), and combined sonicated and glycated OVA (U-G-OVA).121 | |
8.5. Ultrasound-assisted glycation
It is known that after sonication, the structure of protein molecules gets unfolded and altered. A few studies have reported the aspect of phenolic interaction with these destructed protein molecules, especially the effect of sonication on protein-phenolic binding was investigated for emodin-micellar casein,112 fish myofibrillar protein-gallic acid,113 and β-lactoglobulin, and dietary phenolics.114 After pretreating EW with ultrasound at 28% power for 25 min and incubating with epigallocatechin gallate (EGCG, 240 μmol g−1) at pH 7.0 for 2 h, the foaming properties were found to be altered. The FS was enhanced up to 95.10% than that without pretreatment. This was reasoned with the increased absolute zeta potential of the ultrasound effect that more protein got unfolded to become accessible for phenolic interaction.115 It is interesting to find that the application of ultrasound as pre-treatment has a positive effect not only on foaming ability but also helps in achieving better foam stability.
9. Adverse effects of ultrasound on egg foams
The ultrasound treatment involves the continuous generation and collapse of cavitation inside the egg solution. This cavitation would impart a deteriorating effect on protein structures as there would be an increase in temperature and pressure. With such effects, the protein structures are distorted thereby losing the functional benefits of the protein molecules.38 Even though ultrasound has proven to be effective for increasing foaming capacity, foam stabilization is still a challenge, with increased foaming ability the foams developed tend to collapse early. The ultrasound treatment at higher processing conditions leads to protein aggregation which reduced the protein solubility and prevents the hydrophobic groups from coming in contact with the air–water interface.122 The aggregation of protein increases the viscosity and turbidity of the solution which is not favorable for any of the functional benefits occurring in the case of whole eggs too.123 Meanwhile, ultrasound has also been used as a defoaming and degassing agent in the fermentation and seafood processing industries.124
10. Commercial perspectives of ultrasound application on EWPs
According to the literature, it is clear that the application of ultrasound has a critical effect on foaming properties, especially the ability to produce foam. In food industries, particularly in the baking industry, the foam structure is crucial in determining the texture, taste, flavor, and overall quality of baked goods. In that case, ultrasound treatment which leaves no residues, and green technology with simple equipment can help in achieving desirable foaming for doughs and batters when given as a pretreatment. Also, the sonication for shelled eggs has proven beneficial as they help in the sealing of pores preventing unwanted carbon dioxide loss and thinning of eggs. Similarly, ultrasound treatment for frozen EWP powder yielded better foaming results than fresh EWPs, this was true even for 21 days of stored frozen EWPs. These results bring more scope to egg processing industries as EWP powder is much more convenient for storage and transport than fresh whole eggs. In eggs, storage has always been a critical factor in modifying the functional properties but ultrasound treatment could be provided either before storage or to the stored eggs to enhance the foaming properties. When ultrasound could be used to induce foaming properties in frozen EWP powders, the multi-benefit ingredient could widen its application in the food industry with strong promotion and application value. It is also noteworthy to mention about ultrasound-assisted treatments that it has minimal deteriorating effects with enhanced foaming characteristics.
11. Conclusion
The eggs are largely being explored in the bakery industry for their excellent native foamability. Many factors influence foam formation and foam stability. Due to the poor stabilization of native EWP, there are a lot of studies exploring the recovery of the foam stability by adding either ingredient or introducing some treatments that alter the protein structure which in turn influences the foaming profile. Irrespective of the type of treatment given, the nature of the egg solution, whether it contains only EWPs or only EY or mixed proportions, would play a critical role in controlling the foaming characteristics of the solution. Among various treatments, ultrasound has been an area of interest among researchers as it can introduce changes at the structural level without destroying the peptide bonds. The sonication-induced cavitation results in protein unfolding that allows more contact area for hydrophobic groups at the air–water interface. Overall, the power of ultrasound treatment influences the foaming ability, whereas treatment duration and frequency have significant roles in foam stability. Recently, ultrasound is successfully being applied as a pretreatment for various techniques including glycation, hydrolysis, or irradiation, which has exhibited synergistic effects. From the review, it can be understood that foaming ability is directly influenced by various ultrasound parameters like power, frequency, and amplitude. However, attaining desirable foam stability is also critical; ultrasound-assisted treatments have proven enhanced foam stability along with foaming ability. This introduces new scope in exploring various treatments to achieve a desirable foaming profile for egg-based solutions. Applications of ultrasound at various levels of egg processing, from shelled eggs to stored eggs to frozen egg protein powder, are possible and will have potential benefits at each level. By introducing ultrasound treatment, the overall quality of eggs is improved, especially during storage where most of the quality deterioration occurs for egg handling and processing. This way, the treatment can help increase the market value and functional value of egg white proteins.
Overall, the ultrasound treatment on egg solutions does have significant improvement in foaming properties, which is dependent on the operating conditions. Meanwhile, a greater number of researchers should be encouraged on egg solutions to study how the amount of protein or fat influences the overall foaming behavior during ultrasound treatment. Also, there are very limited studies on modeling related to egg foaming, which would provide more information on bubble characteristics and formation. Understanding the controlling parameters involved in designing the foaming profile for egg proteins would in turn help in designing novel food products with functional benefits.
Author contributions
M. Kavimughil: literature survey, drafting the manuscript; S. Dutta: review and editing of the manuscript; J. A. Moses: review and editing of the manuscript; C. Anandharamakrishnan: concept development, review, and supervision.
Conflicts of interest
There are no conflicts to declare.
Acknowledgements
Sayantani Dutta would like to acknowledge the Department of Science and Technology (DST), Government of India, for providing financial support (INSPIRE Faculty program, IFA-17/ENG-238).
References
-
V. A. Vaclavik and E. W. Christian, in Essentials of Food Science, Springer, 2008, pp. 311–327 Search PubMed.
- C. Gonzalez Viejo, S. Fuentes, D. D. Torrico, K. Howell and F. R. Dunshea, J. Food Sci., 2018, 83, 1381–1388 CrossRef CAS PubMed.
- C. G. Viejo, S. Fuentes, K. Howell, D. D. Torrico and F. R. Dunshea, Physiol. Behav., 2019, 200, 139–147 CrossRef PubMed.
- S. M. Deotale, S. Dutta, J. A. Moses and C. Anandharamakrishnan, Food Bioprocess Technol., 2020, 13, 1866–1877 CrossRef CAS.
- S. M. Deotale, S. Dutta, J. A. Moses and C. Anandharamakrishnan, Appl. Food Res., 2021, 1, 100012 CrossRef CAS.
- C. Gonzalez Viejo, D. D. Torrico, F. R. Dunshea and S. Fuentes, Foods, 2019, 8, 596 CrossRef PubMed.
- C. G. Viejo, S. Fuentes, G. Li, R. Collmann, B. Condé and D. Torrico, Food Res. Int., 2016, 89, 504–513 CrossRef PubMed.
- C. Gonzalez Viejo, S. Fuentes, D. Torrico, K. Howell and F. R. Dunshea, J. Sci. Food Agric., 2018, 98, 618–627 CrossRef CAS PubMed.
-
J. A. Gallego-Juárez, Ultrasound in Food Processing, Wiley Blackwell, Chichester, 2017, pp. 1–26 Search PubMed.
- S. Deotale, S. Dutta, J. A. Moses, V. M. Balasubramaniam and C. Anandharamakrishnan, Food Eng. Rev., 2020, 12, 229–250 CrossRef.
- B. Deng, J. De Ruiter and K. Schroën, Foods, 2019, 8, 476 CrossRef CAS PubMed.
- B. S. Murray, Curr. Opin. Colloid Interface Sci., 2020, 50, 101394 CrossRef CAS.
- Y. Yu, Y. Guan, H. Wen, Y. Zhang, J. Liu and T. Zhang, LWT--Food Sci. Technol., 2021, 151, 112094 CrossRef CAS.
- H. Xue, M. Xu, M. Liao, W. Luo, G. Zhang, Y. Tu and Y. Zhao, Food Hydrocolloids, 2021, 110, 106181 CrossRef CAS.
- H. Jing, J. Sun, Y. Mu, M. Obadi, D. J. McClements and B. Xu, Food Funct., 2020, 11, 7084–7094 RSC.
- M. A. Lambrecht, L. J. Deleu, I. Rombouts and J. A. Delcour, Food Hydrocolloids, 2018, 79, 352–370 CrossRef CAS.
- X. Li, Y.-M. Wang, C.-F. Sun, J.-H. Lv and Y.-J. Yang, Foods, 2021, 10, 2238 CrossRef CAS PubMed.
- F. Zhan, M. Youssef, B. R. Shah, J. Li and B. Li, Food Hydrocolloids, 2022, 125, 107435 CrossRef CAS.
- J. Su and A. Cavaco-Paulo, Ultrason. Sonochem., 2021, 105653 CrossRef CAS PubMed.
- S. M. Deotale, S. Dutta, J. A. Moses and C. Anandharamakrishnan, Discover Chem. Eng., 2023, 3, 9 CrossRef.
- A. G. B. Wouters, I. Rombouts, E. Fierens, K. Brijs, C. Blecker, J. A. Delcour and B. S. Murray, Food Hydrocolloids, 2018, 77, 176–186 CrossRef CAS.
- D. Żmudziński, J. Ptaszek Pawełand Kruk, K. Kaczmarczyk, W. Rożnowski, W. Berski, A. Ptaszek and M. Grzesik, J. Food Eng., 2014, 121, 128–134 CrossRef.
- D. Daugelaite, R.-M. Guillermic, M. G. Scanlon and J. H. Page, Colloids Surf., A, 2016, 489, 241–248 CrossRef CAS.
- C. Hill and J. Eastoe, Adv. Colloid Interface Sci., 2017, 247, 496–513 CrossRef CAS PubMed.
- E. Rio, W. Drenckhan, A. Salonen and D. Langevin, Adv. Colloid Interface Sci., 2014, 205, 74–86 CrossRef CAS PubMed.
- G. Narsimhan and N. Xiang, Annu. Rev. Food Sci. Technol., 2018, 9, 45–63 CrossRef CAS PubMed.
- W. She, Y. Du, C. Miao, J. Liu, G. Zhao, J. Jiang and Y. Zhang, Cem. Concr. Res., 2018, 106, 12–22 CrossRef CAS.
- C. Y. Falco, X. Geng, M. Cárdenas and J. Risbo, Food Hydrocolloids, 2017, 70, 211–218 CrossRef.
-
A. J. Wilson, in Foams: Physics, Chemistry and Structure, Springer, 1989, pp. 69–88 Search PubMed.
- T. M. Johnson and M. E. Zabik, J. Food Sci., 1981, 46, 1237–1240 CrossRef CAS.
- Y. Mine, Trends Food Sci. Technol., 1995, 6, 225–232 CrossRef CAS.
- K. Lomakina and K. Mikova, Czech J. Food Sci., 2006, 24, 110–118 CrossRef CAS.
- N. Taccoen, F. Lequeux, D. Z. Gunes and C. N. Baroud, Phys. Rev. X, 2016, 6, 11010 Search PubMed.
- L. Indrawati and G. Narsimhan, J. Food Eng., 2008, 88, 456–465 CrossRef CAS.
- A. Stefanović, J. Jovanović, M. Dojčinović, S. Lević, M. Žuža, V. Nedović and Z. Knežević-Jugović,
et al.
, J. Hyg. Eng. Des., 2014, 6, 215–224 Search PubMed.
- N. Gharbi and M. Labbafi, Food Hydrocolloids, 2019, 90, 72–81 CrossRef CAS.
- V. Raikos, L. Campbell and S. R. Euston, Food Res. Int., 2007, 40, 347–355 CrossRef CAS.
- L. Sheng, Y. Wang, J. Chen, J. Zou, Q. Wang and M. Ma, Food Res. Int., 2018, 108, 604–610 CrossRef CAS PubMed.
- E. Dickinson, Food Hydrocolloids, 1986, 1, 3–23 CrossRef CAS.
- E. A. Foegeding, P. J. Luck and J. P. Davis, Food Hydrocolloids, 2006, 20, 284–292 CrossRef CAS.
- B. P. Binks, Curr. Opin. Colloid Interface Sci., 2002, 7, 21–41 CrossRef CAS.
- E. Vignati, R. Piazza and T. P. Lockhart, Langmuir, 2003, 19, 6650–6656 CrossRef CAS.
- O. Tomar, G. Akarca, Ö. Istek and M. Ataseven, J. Food Process. Preserv., 2021, 45, e15225 CAS.
- D. F. Kirunda, S. E. Scheideler and S. R. McKee, Poult. Sci., 2001, 80, 1378–1383 CrossRef CAS PubMed.
- G. Yenice, O. Kaynar, M. Ileriturk, F. Hira and A. Hayirli, Czech J. Food Sci., 2016, 34, 370–376 CrossRef CAS.
- J. Vlčková, E. Tůmová, K. Míková, M. Englmaierová, M. Okrouhlá and D. Chodová, Poult. Sci., 2019, 98, 6187–6193 CrossRef PubMed.
- C. Alamprese, E. Casiraghi and M. Rossi, Int. J. Food Sci. Technol., 2012, 47, 1411–1420 CrossRef CAS.
- M. Hammershøj and K. B. Qvist, LWT–Food Sci. Technol., 2001, 34, 118–120 CrossRef.
- F. G. Silversides and K. Budgell, Poult. Sci., 2004, 83, 1619–1623 CrossRef CAS PubMed.
- G. Secci, F. Bovera, G. Parisi and G. Moniello, Animals, 2020, 10 Search PubMed.
- Y. Akter, A. Kasim, H. Omar and A. Q. Sazili, J. Food, Agric. Environ., 2014, 12, 87–92 Search PubMed.
- L. Sheng, M. Huang, J. Wang, Q. Xu, H. H. M. Hammad and M. Ma, J. Food Eng., 2018, 219, 1–7 CrossRef CAS.
- A. C. C. Alleoni and A. J. Antunes, Braz. J. Poultry Sci., 2004, 6, 105–110 CrossRef.
- C. Chang, T. Lahti, T. Tanaka and M. T. Nickerson, J. Sci. Food Agric., 2018, 98, 5547–5558 CrossRef CAS PubMed.
- H. Jin, Y. Jin, J. Pan, S. Mi, Q. Zeng, Z. Li, Q. Wang, Y. Sun and L. Sheng, Food Hydrocolloids, 2023, 134, 108033 CrossRef CAS.
- H. Rostamabadi, V. Chaudhary, N. Chhikara, N. Sharma, M. Nowacka, I. Demirkesen, K. Rathnakumar and S. R. Falsafi, Food Hydrocolloids, 2023, 139, 108514 CrossRef CAS.
- S. M. Razi, H. Fahim, S. Amirabadi and A. Rashidinejad, Food Hydrocolloids, 2023, 135, 108183 CrossRef CAS.
- H. Jin, Y. Sun, J. Pan, Y. Fang, Y. Jin and L. Sheng, Food Hydrocolloids, 2022, 124, 107352 CrossRef CAS.
- S. Li, S. Zhang, Y. Liu, X. Fu, X. Xiang and S. Gao, Ultrason. Sonochem., 2022, 84, 105958 CrossRef CAS PubMed.
- L. Hu, L. Wu, C. Lai, M. Li and W. Yang, J. Dispersion Sci. Technol., 2022, 43, 1755–1765 CrossRef CAS.
- T. M. Johnson and M. E. Zabik, Poult. Sci., 1981, 60, 2071–2083 CrossRef CAS.
- R. Nakamura and Y. Sato, Agric. Biol. Chem., 1964, 28, 524–534 CAS.
- N. Gharbi and M. Labbafi, Food Chem., 2018, 252, 126–133 CrossRef CAS PubMed.
- B. Ma, X. Fu, P. Zhu, Z. Lu, J. Niu and F. Lu, Crit. Rev. Food Sci. Nutr., 2023, 1–17 CrossRef PubMed.
- Y. Zhu, S. K. Vanga, J. Wang and V. Raghavan, Trends Food Sci. Technol., 2018, 78, 188–196 CrossRef CAS.
- Z. F. Bhat, J. D. Morton, A. E.-D. A. Bekhit, S. Kumar and H. F. Bhat, Compr. Rev. Food Sci. Food Saf., 2021, 20, 4703–4738 CrossRef CAS PubMed.
- W. He, N. Xiao, Y. Zhao, Y. Yao, M. Xu, H. Du, N. Wu and Y. Tu, J. Food Sci., 2021, 86, 656–666 CrossRef CAS PubMed.
- K. Chang, J. Liu, W. Jiang, Y. Fan, B. Nan, S. Ma, Y. Zhang, B. Liu and T. Zhang, LWT--Food Sci. Technol., 2021, 146, 111383 CrossRef CAS.
- S. M. Razi, A. Motamedzadegan, S.-A. Shahidi and A. Rashidinejad, Int. J. Chem. Eng., 2019, 1–8 CAS.
- S. M. Razi, A. Motamedzadegan, S. A. Shahidi and A. Rashidinejad, Food Nutr. J., 2018, 3, 192 Search PubMed.
- J. Sun, H. Jing, Y. Mu, D. J. McClements, S. Dong and B. Xu, Food Hydrocolloids, 2020, 108, 106019 CrossRef CAS.
- F. Niu, J. Zhou, D. Niu, C. Wang, Y. Liu, Y. Su and Y. Yang, Food Hydrocolloids, 2015, 47, 14–20 CrossRef CAS.
- F. Niu, D. Niu, H. Zhang, C. Chang, L. Gu, Y. Su and Y. Yang, Food Hydrocolloids, 2016, 52, 607–614 CrossRef CAS.
- C. J. F. Souza and E. E. Garcia-Rojas, Food Hydrocolloids, 2017, 66, 268–275 CrossRef CAS.
- F. Alavi, Z. Tian, L. Chen and Z. Emam-Djomeh, Food Hydrocolloids, 2020, 106, 105887 CrossRef CAS.
- T. Tang, S. Wu, S. Tang, N. Xiao, L. Wu, Y. Tu and M. Xu, J. Food Eng., 2022, 326, 111012 CrossRef CAS.
- L. Liu, Y. Li, S. Prakash, X. Dai and Y. Meng, Int. J. Food Prop., 2018, 21, 395–406 CrossRef CAS.
- L. Liu, Y. Li, X. Dai, Y. Zhu, W. Hao and X. Yang, J. Food Process. Preserv., 2020, 44, e14934 CAS.
- J. Jia, B. Ji, L. Tian, M. Li, M. Lu, L. Ding, X. Liu and X. Duan, Food Hydrocolloids, 2021, 111, 106218 CrossRef CAS.
- L. Ding, L. Lu, L. Sheng, C. Tang, Y. Chen and Z. Cai, Food Chem., 2020, 317, 126349 CrossRef CAS PubMed.
- M. Yüceer and C. Caner, J. Food Sci. Technol., 2021, 1–8 Search PubMed.
- X. Luo, Q. Wang, Y. Wu, W. Duan, Y. Zhang, F. Geng, H. Song, Q. Huang and F. An, LWT--Food Sci. Technol., 2022, 154, 112807 CrossRef CAS.
- H. Wang, Y. Ma and Y. Chi, Foods, 2023, 12(7), 1474 CrossRef CAS PubMed.
- L. Wu, W. Zhao, R. Yang and X. Chen, J. Food Eng., 2014, 139, 13–18 CrossRef CAS.
- L. Wu, W. Zhao, R. Yang and W. Yan, Food Chem., 2015, 175, 115–120 CrossRef CAS PubMed.
- L. Wu, W. Zhao, R. Yang, W. Yan and Q. Sun, J. Sci. Food Agric., 2016, 96, 3334–3341 CrossRef CAS PubMed.
- A. Panozzo, L. Manzocco, S. Calligaris, I. Bartolomeoli, M. Maifreni, G. Lippe and M. C. Nicoli, Food Res. Int., 2014, 62, 718–725 CrossRef CAS.
- A. Singh, M. Sharma and H. S. Ramaswamy, Int. J. Food Prop., 2015, 18, 558–571 CrossRef.
- S. M. Razi, A. Motamedzadegan, L. Matia-Merino, S.-A. Shahidi and A. Rashidinejad, Food Hydrocolloids, 2019, 94, 399–410 CrossRef CAS.
- M. Sachadyn-Król, M. Materska, B. Chilczuk, M. Karaś, A. Jakubczyk, I. Perucka and I. Jackowska, Food Chem., 2016, 211, 59–67 CrossRef PubMed.
- A. Segat, N. N. Misra, A. Fabbro, F. Buchini, G. Lippe, P. J. Cullen and N. Innocente, Food Res. Int., 2014, 66, 365–372 CrossRef CAS.
- P. M. Souza, P. Mendes, A. Müller, A. Beniaich, E. Mayer-Miebach, K. Oehlke, M. Stahl, R. Greiner and A. Fernández, Innovative Food Sci. Emerging Technol., 2015, 32, 156–164 CrossRef.
- Y.-H. Kuan, R. Bhat and A. A. Karim, J. Agric. Food Chem., 2011, 59, 4111–4118 CrossRef CAS PubMed.
- L. Ding, M. Xia, Q. Zeng, Q. Zhao, Z. Cai and Z. Zhu, LWT--Food Sci. Technol., 2022, 153, 112505 CrossRef CAS.
- S. Dutta, S. R. Priyadarshini, J. A. Moses and C. Anandharamakrishnan, ChemBioEng Rev., 2021, 8, 1–12 CrossRef.
- A. B. Stefanović, J. R. Jovanović, S. Ž. Grbavčić, N. Ž. Šekuljica, V. B. Manojlović, B. M. Bugarski and Z. D. Knežević-Jugović, Eur. Food Res. Technol., 2014, 239, 979–993 CrossRef.
- Y. Myroshnyk, V. Dotsenko, L. Sharan and V. Tsyrulnikova, East.-Eur. J. Enterp. Technol., 2020, 1, 103 Search PubMed.
- Y. Meng, Z. Liang, C. Zhang, S. Hao, H. Han, P. Du, A. Li, H. Shao, C. Li and L. Liu, LWT--Food Sci. Technol., 2021, 152, 112272 CrossRef CAS.
- A. R. Jambrak, T. J. Mason, V. Lelas, L. Paniwnyk and Z. Herceg, J. Food Eng., 2014, 121, 15–23 CrossRef CAS.
- R. Morales, K. D. Martínez, V. M. Pizones Ruiz-Henestrosa and A. M. R. Pilosof, Ultrason. Sonochem., 2015, 26, 48–55 CrossRef CAS PubMed.
- S. Yan, J. Xu, S. Zhang and Y. Li, LWT--Food Sci. Technol., 2021, 142, 110881 CrossRef CAS.
- S. Martini, R. Potter and M. K. Walsh, Food Res. Int., 2010, 43, 2444–2451 CrossRef CAS.
- J. A. Téllez-Morales, B. Hernández-Santo and J. Rodr\’\iguez-Miranda, Ultrason. Sonochem., 2020, 61, 104787 CrossRef PubMed.
- A. B. Stefanović, J. R. Jovanović, M. B. Dojčinović, S. M. Lević, V. A. Nedović, B. M. Bugarski and Z. D. Knežević-Jugović, Food Bioprocess Technol., 2017, 10, 1224–1239 CrossRef.
- J. Brand, A. Silberbauer and U. Kulozik, Food Bioprocess Technol., 2016, 9, 501–510 CrossRef CAS.
- J. Brand and U. Kulozik, Food Bioprocess Technol., 2016, 9, 1210–1218 CrossRef CAS.
- C. Arzeni, K. Martinez, P. Zema, A. Arias, O. E. Pérez and A. M. R. Pilosof, J. Food Eng., 2012, 108, 463–472 CrossRef CAS.
- C. Arzeni, O. E. Pérez and A. M. R. Pilosof, Food Hydrocolloids, 2012, 29, 308–316 CrossRef CAS.
- W. Xiong, Y. Wang, C. Zhang, J. Wan, B. R. Shah, Y. Pei, B. Zhou, J. Li and B. Li, Ultrason. Sonochem., 2016, 31, 302–309 CrossRef CAS PubMed.
- C. Caner and M. Yuceer, J. Sci. Food Agric., 2015, 95, 2880–2891 CrossRef CAS PubMed.
- Y. Li, L. Yu, L. Wang and W. Xiong, Food Biophys., 2023, 18, 198–207 CrossRef.
- X. Duan, M. Li, J. Shao, H. Chen, X. Xu, Z. Jin and X. Liu, Food Hydrocolloids, 2018, 75, 223–228 CrossRef CAS.
- H. S. Vo, S. Kentish and M. Ashokkumar, Colloids Surf., A, 2011, 380, 35–40 CrossRef CAS.
- B. Lei, K. Majumder, S. Shen and J. Wu, Food Chem., 2011, 124, 808–815 CrossRef CAS.
- S. Jun, M. Yaoyao, J. Hui, M. Obadi, C. Zhongwei and X. Bin, J. Food Eng., 2020, 277, 109902 CrossRef.
- D. Nagy, V. Zsom-Muha, C. Németh and J. Felföldi, Prog. Agric. Eng. Sci., 2021, 17(S1), 1–8 Search PubMed.
- Y. Xie, J. Wang, Y. Wang, D. Wu, D. Liang, H. Ye, Z. Cai, M. Ma and F. Geng, Ultrason. Sonochem., 2020, 60, 104767 CrossRef CAS PubMed.
- F. Geng, Y. Xie, Y. Wang and J. Wang, Food Chem., 2021, 354, 129580 CrossRef CAS PubMed.
- X. Bi, X. Wang, Y. Chen, L. Chen, Y. Xing and Z. Che, Ultrason. Sonochem., 2020, 60, 104763 CrossRef CAS PubMed.
- X. Fu, Q. Liu, C. Tang, J. Luo, X. Wu, L. Lu and Z. Cai, Ultrason. Sonochem., 2019, 58, 104644 CrossRef CAS PubMed.
- X. Liu, Q. Yang, M. Yang, Z. Du, C. Wei, T. Zhang, B. Liu and J. Liu, Ultrason. Sonochem., 2021, 73, 105477 CrossRef CAS PubMed.
- Y. Xie, J. Wang, Y. Shi, Y. Wang, L. Cheng, L. Liu, N. Wang, H. Li, D. Wu and F. Geng, Ultrason. Sonochem., 2020, 63, 104933 CrossRef CAS PubMed.
- Y. Chen, L. Sheng, M. Gouda and M. Ma, LWT--Food Sci. Technol., 2019, 113, 108303 CrossRef CAS.
-
M. Villamiel, E. Riera and J. V. García-Pérez, Innovative Food Processing Technologies: A comprehensive Review, 2021, vol. 415, p. 438 Search PubMed.
- H. Jin, Y. Sun, J. Pan, Y. Fang, Y. Jin and L. Sheng, Food Hydrocolloids, 2022, 124, 107352 CrossRef CAS.
- J. Wang, X. Liu, S. Li, H. Ye, W. Luo, Q. Huang and F. Geng, Food Chemistry, 2022, 366, 130596 CrossRef CAS PubMed.
- Y. Yu, H. Zhang, J. Zhu, J. Liu and T. Zhang, J. Food Sci., 2020, 85(12), 4312–4318 CrossRef CAS PubMed.
- A. A. Townsend and S. Nakai, J. Food Sci., 1983, 48(2), 588–594 CrossRef CAS.
- J. O’sullivan, B. Murray, C. Flynn and I. Norton, Food hydrocolloids, 2016, 53, 141–154 CrossRef.
- L. Sheng, Y. Wang, J. Chen, J. Zou, Q. Wang and M. Ma, Food Res. Int., 2018, 108, 604–610 CrossRef CAS PubMed.
- R. J. Delahaije, F. J. Lech and P. A. Wierenga, Food Hydrocolloids, 2019, 91, 263–274 CrossRef CAS.
- S. Jun, M. Yaoyao, J. Hui, M. Obadi, C. Zhongwei and X. Bin, J. Food Eng., 2020, 277, 109902 CrossRef.
- J. Sun, C. Chang, Y. Su, L. Gu, Y. Yang and J. Li, Food Hydrocolloids, 2022, 122, 107088 CrossRef CAS.
|
This journal is © The Royal Society of Chemistry 2023 |