Food-related engineered nanoparticles and food-grade TiO2 impact the metabolism of a human commensal bacterial strain in physiologically relevant conditions†
Received
18th July 2022
, Accepted 17th November 2022
First published on 22nd November 2022
Abstract
Considering the widespread application of nanotechnology in the food industry, humans are increasingly exposed to food-related engineered nanoparticles (NPs). Upon ingestion, NPs come into contact with human commensal bacteria that play an important role in human health and disease. Therefore, the effects and biotransformation of food-related NPs in the digestive tract, including microbiota, need to be assessed. Herein, artificial saliva was used as a physiologically relevant test medium to investigate the effects of Ag, SiO2 and TiO2 NPs and food-grade TiO2 on the growth and antagonistic activity of Streptococcus salivarius K12, a human beneficial bacterium inhabiting the oral cavity, and bacterially induced biotransformation of NPs. SiO2 NPs, TiO2 NPs and food-grade TiO2 at concentrations up to 100 mg L−1 did not affect the growth of S. salivarius K12, while Ag NPs were expectedly growth-inhibitory already at 10 mg L−1, both in aerobic and anaerobic conditions. At sub-growth-inhibitory concentrations, NPs and food-grade TiO2 significantly modified the transcription of structural genes (salA and sboA) of two bacteriocins (salivaricins) in S. salivarius K12 genome, signal transduction gene (sboK) and bacteriocin immunity conferring gene (sboG, an ABC transporter). Changes in gene expression were accompanied by the increased antagonistic activity of the bacterial strain. Spent artificial saliva, where bacteria had been cultured, markedly affected the transformation of NPs compared to fresh artificial saliva. The transformations included significant agglomeration, increased dissolution of Ag NPs and enhanced bioavailability of SiO2 NPs, TiO2 NPs and food-grade TiO2, while Ag NPs became less toxic. The results showed that food-related NPs can affect beneficial bacterial traits needed for competitiveness in microbiota and bacterial activity influences the transformation of NPs, potentially affecting the fate and toxicity of NPs in the digestive tract.
Environmental significance
Humans are chronically exposed to inorganic nanomaterials (NM) that are added to food for enhanced quality and shelf-life. While food-grade NM have been considered generally safe to humans, the indirect effects manifested through microbiota are unknown. Here we found that engineered NM, representative of typical food-added NM, and food-grade TiO2, at relevant concentrations for human daily oral exposure, affected bacteriocin transcription by oral commensal bacteria, potentially impacting their competitiveness in oral microbiota. Importantly, food-grade TiO2 and engineered TiO2 nanoparticles had opposite effects on the bacteriocin gene expression as well as different physico-chemical properties in artificial saliva, highlighting the importance of including food-grade materials as well as representative human commensal bacteria in the risk assessment of NM.
|
1. Introduction
Owing to the unique physicochemical properties of engineered nanoparticles (NPs), they are widely used in the food industry to improve the taste or sensory, nutritional and visual properties of products, enhance the bioavailability of nutrients, or control flavor release.1 For example, nanosized Ag can be added as a coloring agent in cakes and candies, used as a food supplement or added to packaging materials in the form of nano-coatings to prevent microbial contamination, thereby extending the shelf life of foods.2 The European Food Safety Authority (EFSA) estimates that daily exposure levels of Ag NPs range from 0.03 to 2.6 μg kg−1 of body weight (bw) for adults and children, respectively.3 SiO2 NPs are added as anticaking agents to powdered foods, such as salt, spices, and milk powder,4 with daily exposure levels ranging between 2.7 and 31.2 mg kg−1 bw in children and 0.9 and 13.2 mg kg−1 bw in adults.5 Food-grade TiO2 is used as a flocculant and whitening agent in candy, sauces and chewing gum6,7 and its daily exposure levels range from 0.9 to 10.4 mg kg−1 bw in children, and 0.3 to 6.8 mg kg−1 bw in adults.8 With the increasing use of NPs in food packaging, there is a potential for NP migration to food that is unaccounted for in the current human NP exposure levels.9
Recently, the health effects of food-grade NPs have received considerable attention in risk assessment and regulation. France issued a national ban on the usage of TiO2 (E171) in food starting from 2020 and in 2021, EFSA followed by issuing a warning regarding food-grade TiO2 (E171), disputing the safety of its use as a food additive due to potential genotoxicity risks.10 Although inorganic NPs may not induce acute toxicity to humans at the concentrations added in food,11,12 the effects may manifest at the molecular level, e.g., inducing oxidative stress which may lead to inflammation and chronic diseases.13 In addition to the risks associated with NPs directly interacting with human cells, there is evidence that the chronic effects of NPs on human health may be mediated via microbiota which is known to have important physiological roles in the host health, while dysbiosis has been associated with inflammation, obesity, cancer and neurological diseases.14,15In vivo studies have demonstrated that ingested NPs modulate the composition and metabolism of gut microbial communities which are associated with physiological changes in the host.16,17 Chronic exposure to TiO2 may even lead to permanent genetic changes that increase the pathogenicity of normally commensal bacteria.18 The potential hazards that may result from NPs have been demonstrated, but health risk assessment of NPs is still in its infancy and in vitro and in vivo studies have typically focused on gut microbiota, with relatively few studies on the effects of NPs on host oral microbiota.
The oral cavity is the entryway of ingested NPs to the organism where NPs instantly interact with the components of the saliva and mucus layer on the epithelial cells.19 Oral mucosal surfaces are also inhabited by diverse microbial populations, including probiotic bacteria, that become into contact with ingested NPs. One of the earlier colonizers of the upper digestive tract after birth is Streptococcus salivarius K12, a Gram-positive human commensal bacterium belonging to the order Lactobacillales.20S. salivarius K12 is a facultative anaerobe, known to secrete bacteriocin-like inhibitory substances (BLIS), called salivaricins, that inhibit the growth of closely related, often pathogenic, bacterial species.21 In addition, salivaricins have immunomodulatory properties (via affecting nuclear factor-κB or NF-κB pathways and proinflammatory cytokines) to promote host defense processes against inflammation.22 Due to its well-characterized beneficial properties, S. salivarius K12 serves as a highly suitable model for NP effect assessment on human commensal bacteria. Specifically, as NP interactions with bacteria are strongly influenced by their cell wall structure, S. salivarius K12 as a Gram-positive bacterium represents the majority of the commensal microbiota.17 Also, S. salivarius K12 as a facultative anaerobe allows assessing NP effects in aerobic and anaerobic conditions, representative of oxygen level gradients along the digestive tract. Its ability to acidify the surrounding medium during growth provides a time-evolving pH gradient for NP effect studies to simulate the intraluminal pH range of the gastrointestinal tract.23 The bacterially secreted compounds in physiologically relevant conditions may transform food-related NPs, thus changing their physico-chemical properties24,25 and possibly bioactivity.26
As the potential consequences of interactions between oral symbiotic bacteria and NPs remain largely unexplored, we assessed the effects of food-related NPs (Ag, SiO2 and TiO2) and food-grade TiO2 (not fully nanosized) on the model human beneficial bacterium (S. salivarius K12) and bacterially induced biotransformation of NPs under relevant physiological conditions. Since Ag that is authorized as a food additive in the EU (E174) contains 97% of nanosized particles27 and the primary size of food-grade SiO2 particles is in the range of 9–26 nm,28 NPs assessed here were representative of food-grade materials. However, food-grade TiO2 has been shown to contain ∼20% of NPs with diameter <100 nm.29 Nanosized TiO2 was included in this study to simulate a worst-case scenario (i.e., if fully nanosized TiO2 was consumed) and compared to the effects of food-grade TiO2. S. salivarius K12 was exposed to sub-growth-inhibitory levels of Ag, SiO2 and TiO2 NPs and food-grade TiO2 to assess the effects of potential oral exposure to these NPs on the competitiveness of an oral probiotic bacterium in physiologically relevant conditions (artificial saliva, aerobic versus anaerobic environments). The bacterial ability to transcribe and secrete antibacterial compounds that are crucial for controlling the abundance of closely related pathogenic bacterial strains were quantified under NP and food-grade TiO2 stress. The biological effects of NPs and food-grade TiO2 were correlated with their physico-chemical transformations in artificial saliva which either contained secreted bacterial compounds or not. The study employed a human beneficial bacterial strain in artificial saliva, to simulate its natural ecological niche, which allowed the assessment of food-relevant NP effects in physiologically relevant conditions to explore the potential impacts on human health via NP interactions with the commensal bacteria.
2. Materials and methods
2.1 Materials
Ag (60–80 nm, 99.9%, XFJ14-1), SiO2 (20 nm, 99%, XFI03) and TiO2 NPs (20–40 nm, 99%, XFI02) were purchased as powders from XFNano (Nanjing, China). Food-grade TiO2 (200–500 nm, 98.5%) was purchased from Jiangsu Hushen Titanium White Technology Co. Ltd. (Shanghai, China). Potassium chloride, potassium dihydrogen phosphate, magnesium chloride hexahydrate, potassium bicarbonate, sodium nitrate, calcium carbonate, ammonium chloride, urea, and D-glucose (all 99% pure) were purchased from J&K Scientific (Beijing, China). All the reagents were of analytical grade and used as obtained without further purification. Ultrapure water from a Direct-Q system (Millipore, Billerica, MA, USA) with a resistivity of 18.2 MΩ cm−1 was used for the preparation of all solutions.
2.2 Preparation of stock dispersions and physico-chemical characterization of NPs and food-grade TiO2
The stock dispersions of NPs and food-grade TiO2 were prepared by weighing 100 mg of Ag, SiO2, TiO2, or food-grade TiO2 into a sterile, acid-cleaned bottle and adding 50 mL sterile ultrapure water. To disperse the NPs, the bottles with stock dispersions were placed in an ultrasonication bath for 15 min and then stored at 4 °C. The morphology and pristine size of NPs were characterized by using scanning electron microscopy (SEM, HITACHI SU8010, Tokyo, Japan) at 5–10 kV, and transmission electron microscopy (TEM, HITACHI HT7700, Tokyo, Japan) at 100 kV. SEM samples were prepared by coating the conductive gel with the NP stock dispersion immediately after bath sonication for 15 min, after which the excess solution was blotted off with blotting paper and the sample was allowed to air-dry. TEM samples were prepared by depositing ∼3 μL of NP dispersion (0.02 g L−1) on a carbon-coated copper grid and allowed to air-dry. The particle size distribution was obtained by measuring approximately 100 NPs in the SEM or TEM images of each NP using ImageJ.30 The hydrodynamic size and ζ potential of NPs were determined by using Zetasizer Nano S90 (Malvern, UK) and nanoPartica SZ-100V2 (Horiba, Kyoto, Japan), respectively. For this, NP dispersions were prepared in ultrapure water or culture media at 100 mg L−1 and measured immediately after dilution. Three independent measurements were taken.
2.3 Bacterial culturing
S. salivarius K12 was isolated from a commercial dietary supplement BLIS ThroatGuard Pro (Blis Technologies Limited, Dunedin, New Zealand) as reported previously.22 One lozenge was aseptically ground, dissolved in 1 mL of Todd-Hewitt broth (THB, Oxoid, Basingstoke, UK) and serially diluted. From each dilution, 30 μL was pipetted onto an individual Petri plate containing Columbia blood agar (CBA or Columbia agar base, Oxoid, Basingstoke, UK, supplemented with 5% sheep blood, Nanjing Lezhen Biotechnology Co., Ltd, China, and 0.1% CaCO3). The plates were incubated aerobically at 37 °C for 48 h, then the CBA plate which had been inoculated with the 1 × 105-fold diluted lozenge suspension was used to collect a single bacterial colony and streaked onto a fresh CBA plate. After 48 h incubation at 37 °C the colonies were used to prepare long-term glycerol (15% v/v in THB) stocks for storage at −80 °C. For experiments, S. salivarius K12 was streaked from a frozen stock onto a CBA plate and cultivated at 37 °C for 48 h aerobically. For growth in liquid media, several colonies from the agar plate were dispersed in the culture medium and optical density at 600 nm (OD600) was adjusted to 0.01 in a 96-well plate (100 μL per well). The liquid culture medium used for S. salivarius K12 was either THB or artificial saliva. Artificial saliva with a ‘representative meal’ was prepared as reported previously:31 0.90 g L−1 KCl, 0.66 g L−1 KH2PO4, 0.08 g L−1 MgCl2·6H2O, 0.49 g L−1 KHCO3, 0.37 g L−1 NaNO3, 0.12 g L−1 CaCO3, 0.6 g L−1 NH4Cl, 0.2 g L−1 urea and 2 g L−1 porcine stomach type II mucin (Sigma-Aldrich, St. Louis, MO, USA), with 5 g L−1 glucose and 1.4 g L−1 meat extract (Solarbio, Beijing, China) added as a representative meal. pH was adjusted to 6.7 with HCl and the medium was sterilized by autoclaving. Micrococcus luteus (CGMCC1.3749, Beijing, China) was used as an indicator strain in a deferred antagonism test. M. luteus was maintained as a frozen stock at −80 °C in 15% (v/v) glycerol in nutrient broth (Oxoid, Basingstoke, UK) and was streaked onto CBA plate for culturing at 37 °C for 48 h, aerobically.
2.4 Bacterial growth assays in aerobic and anaerobic conditions
The effects of NPs and food-grade TiO2 on the growth of S. salivarius K12 were determined by quantifying the ATP concentrations of the cultures over time as described previously.32,33 Briefly, the dilutions of NPs or food-grade TiO2 were prepared in THB or artificial saliva at twice the final exposure concentrations and 50 μL of each dilution or the culture medium (for controls) were pipetted in the wells of a clear 96-well plate (NEST, Wuxi, China). S. salivarius K12 inoculum, prepared by suspending a few colonies from the CBA plate in THB or artificial saliva to achieve OD600 of 0.02, was added in 50 μL portions to the wells of the microplate containing 50 μL of medium or NP or food-grade TiO2 dilutions, yielding the bacterial OD600 of 0.01. The NP or food-grade TiO2 concentrations in the wells were 1, 10 and 100 mg L−1, AgNO3 was used as a positive control at the concentration which inhibited the specific growth rate at a pre-determined level compared to untreated bacterial culture. Culture medium (100 μL) with or without NPs or food-grade TiO2 at each tested concentration or AgNO3 were included in the microplates as abiotic controls. To achieve anaerobic conditions, 100 μL of bacterial suspensions with or without NPs and controls in the wells were overlayed with 100 μL of sterile mineral oil (Sigma-Aldrich, St. Louis, MO, USA) as reported previously.34 The microplates were incubated at 37 °C statically in the incubator and the OD600 of the contents of the wells was recoded every hour using a Spark microplate reader (TECAN, Männedorf, Switzerland). For ATP extraction, at 0, 3, 5, 7, 9, 11 and 24 h, two wells per treatment or control were sacrificed by pipetting 100 μL of ice-cold ATP extraction solution (4% trichloroacetic acid, TCA and 4 × 10−3 M ethylenediaminetetraacetic acid, EDTA) into the microplate wells and mixed by pipetting. In the case of the wells overlayed with mineral oil for anaerobic culturing, oil was removed from the wells by pipetting before the addition of ATP extraction solution. The contents of the wells were then transferred to 1.5 mL centrifuge tubes, vortexed, placed on ice for 10 min and were then frozen at −20 °C until analysis. ATP was quantified using ATP Bioluminescent Assay Kit (Sigma-Aldrich, St. Louis, MO, USA)35 and a Spark microplate reader. For pH measurements of the bacterial growth medium during culturing, the contents of the microplate wells were transferred at regular time intervals to 1.5 mL centrifuge tubes, centrifuged at 10
000g for 10 min and the pH of the supernatant was measured with indicator paper.
2.5 Deferred antagonism assay
To assess the antagonistic activity of S. salivarius K12, a soft-agar overlay technique was used.36S. salivarius K12 was grown in artificial saliva with or without NPs (10 mg L−1) or food-grade TiO2 (10 mg L−1), aerobically at 37 °C and 2 μL portions of the cultures were spotted on CBA plates at different times over 7–22 h of cultivation. CBA plates were cultivated at 37 °C to allow growth of S. salivarius K12. After 12 h cultivation, S. salivarius K12 was killed by exposure to chloroform vapors for 30 min (ref. 37) and the remaining CHCl3 vapors were evaporated by leaving the agar plates open in the biohood for an additional 30 min. Then, 5 μL of nisin (100 IU mL−1, Sigma Aldrich, St. Louis, MO, USA) was spotted on agar as a positive control for antibacterial action. A few colonies of the indicator strain M. luteus were transferred to nutrient broth and the OD600 was adjusted to 0.6. Soft agar (0.5% agar in deionized water) was brought to 60 °C, 3 mL portion was pipetted into a sterile borosilicate test tube, let cool slightly before adding 100 μL of M. luteus suspension, mixed by vortexing and poured over chloroform-killed S. salivarius K12 spots on the CBA plate. The procedure was repeated for each CBA plate. After solidification the plates were cultivated aerobically at 37 °C overnight. The diameters of the inhibitory zones were measured to quantify the antibacterial efficiency of S. salivarius K12.
2.6 Bacterial exposures to NPs or food-grade TiO2, RNA extraction and quantitative PCR analysis
The expression of salivaricin genes of S. salivarius K12 (with and without NP or food-grade TiO2 exposures) in artificial saliva was quantified by using real-time quantitative polymerase chain reaction (RT-qPCR) analysis. S. salivarius K12 was cultivated in artificial saliva with or without SiO2, TiO2 or Ag NPs or food-grade TiO2 at 1 and 10 mg L−1 either in aerobic or anaerobic conditions at 37 °C, 200 rpm, for 6 h. Anaerobic conditions were achieved by placing the test tubes in an airtight box which was depleted of oxygen and enriched in CO2 by using AnaeroPack-Anaero sachets (MGC, Niigata, Japan). To measure salivaricin gene expression after different exposure times to NPs or food-grade TiO2, several colonies of S. salivarius K12 were transferred from CBA plates to artificial saliva and first incubated without NPs for 6 h at 37 °C, 200 rpm in aerobic conditions. After that, 1.5 mL of bacterial culture was added to borosilicate test tubes, followed by an equal volume of NP dispersions in artificial saliva to yield exposure concentrations of 1 and 10 mg L−1 SiO2, TiO2, or Ag NPs or food-grade TiO2 and cultured at 37 °C, 200 rpm, aerobically. At 1, 4 and 18 h 3 mL of cultures were harvested by centrifugation at 4 °C, 7000g for 8 min and the supernatant was discarded. 1 mL of Trizol reagent (Takara Bio, Kusatsu, Japan) was added to the pellet and mixed by pipetting until complete lysis. Then, 100 μL of chloroform was added, mixed and let stand for 5 min. After centrifugation at 4 °C, 12
000g for 15 min 500 μL of supernatant was mixed with an equal volume of isopropanol, shaken and let stand for 20 min. A portion of 700 μL of 80% anhydrous ethanol was added and the tube was inverted repeatedly to mix, then centrifuged at 4 °C, 12
000g for 5 min. The step was repeated twice. Finally, the supernatant was discarded and RNA was air-dried. Target genes salA, sboA, sboG and sboK were selected based on previous studies.38,39gyrA was used as a reference gene.40 Primers for RT-qPCR were designed using an NCBI tool (Table S1†). All samples were analyzed on a qTower3 G touch RT-qPCR machine (Analytik Jena, Germany) using the SYBR Green Master Mix (Yeasen, Shanghai, China). The reaction volume was 20 μL, consisting of 1 μL cDNA template, 10 μL SYBR Green Master Mix, 0.5 μL each of forward and reverse primers (10 μM), and 8 μL of ultrapure water. PCR amplification conditions were 95 °C for 5 min, followed by 40 cycles of 95 °C for 10 seconds, 60 °C for 30 seconds, and 72 °C for 20 seconds. The RT-qPCR analysis was conducted in three technical replicates. The results were analyzed using the qPCRsoft 4.0 software and calculation was performed using the comparative Ct method (2(−ΔΔCt)).41
2.7 Preparation of spent artificial saliva
NP and food-grade TiO2 transformation was investigated in fresh and spent artificial saliva. To prepare spent artificial saliva, S. salivarius K12 was cultivated aerobically in artificial saliva at 37 °C, 200 rpm for 18 h. Artificial saliva without S. salivarius K12 was incubated in identical conditions as a fresh medium control. Both media, i.e., “spent” and “fresh” medium, were then centrifuged at 4 °C, 12
000g for 10 min, supernatants were collected and filtered through a 0.45 μm microporous membrane (Jet Biofil, Guangzhou, China). The media were used in NP transformation assays as described below.
2.8 Fourier transform infrared (FTIR) spectroscopy
In sterile glass test tubes, 1.5 mL of spent or fresh media (prepared as described in section 2.7) were mixed with 1.5 mL of NP or food-grade TiO2 stock dispersions (2 g L−1), to achieve concentrations of 1 g L−1, incubated aerobically at 37 °C, 200 rpm, for 24 h. Then, supernatants were removed after centrifugation at 4 °C, 15
000g for 10 min and the NP precipitate at the bottom was collected for freeze-drying. The freeze-dried and pristine NPs or food-grade TiO2 were prepared for FTIR spectroscopy by mixing the samples with 0.07 g of KBr powder in an agate mortar, ground and pressed with a tablet press. The spectra in the mid-infrared region of 400–4000 cm−1 were obtained for the pristine and transformed NPs and food-grade TiO2 using an FTIR spectrometer (Thermo Nicolet iS50, Japan). The spectra were analyzed using Origin software (OriginLab, Northampton, MA, USA).
2.9 Sodium dodecyl-sulfate polyacrylamide gel electrophoresis (SDS-PAGE)
The spent and fresh media were prepared as described in section 2.7. A portion of fresh or spent medium (9.5 mL) was pipetted into sterile glass beakers, 0.5 mL of NP and food-grade TiO2 stock dispersion (2 g L−1) was added to achieve concentrations of 100 mg L−1 and incubated aerobically in a shaker (200 rpm) at 37 °C for 24 h. Then, the supernatant was removed after centrifugation at 4 °C, 15
000g for 20 min. NP precipitates were then washed with 100 μL of 1× phosphate buffered saline (PBS, pH 7.4, Gibco, Grand Island, NY, USA) and centrifuged at 12
000g for 5 min at 4 °C. The supernatant was removed and NPs were suspended in 60 μL RIPA lysis buffer and 15 μL 5× SDS-PAGE sample loading buffer (Biosharp, Hefei, China), then 0.6 μL protease inhibitor (MCE, Monmouth Junction, NJ, USA) was added, vortexed and boiled for 20 min, followed by centrifugation at 4 °C, 12
000g for 15 min, and the supernatant was collected. Then, 10 μL of protein samples were added to a 7.5% polyacrylamide separation gel and 5% stacking gel and run in running buffers (cathodic electrophoresis solution: 6.05 g Tris-base, 8.95 g tricine, 5 mL 10% SDS added into 495 mL H2O; anodic electrophoresis solution: 12.12 g Tris-base, 1.66 mL HCl added into 500 mL H2O) at 60 V for about 1 h, followed by a run at 140 V for approximately 40 min. The PageRuler Prestained Protein Ladder (Thermo Scientific, Waltham, MA, USA) was run as a molecular weight standard (10–180 kDa). Gel protein bands were visualized by Coomassie Brilliant Blue R-250 (BioFroxx, Einhausen, Germany).
2.10 Ultraviolet-visible (UV-vis) spectroscopy
For UV-vis, separation of NPs or food-grade TiO2 from the spent medium was not done by centrifugation, differently from sample preparation for other characterization methods, to avoid particle agglomeration. Thus, particle biocorona formation experiments in the bacterial spent medium were done using dialysis tubing. First, artificial saliva was filtered through a 0.45 μm microporous membrane (Jet Biofil, Guangzhou, China) to remove larger particulate matter from the medium. The filtered artificial saliva was inoculated with S. salivarius K12 at the OD600 of 0.01. A portion of NPs or food-grade TiO2 dispersion (1.5 mL, 2 g L−1) were loaded into the dialysis tubings (10 kDa MWCO, Sangon Biotech, Shanghai, China) which were placed into the beakers containing 1.5 mL inoculated artificial saliva. The beakers were incubated at 37 °C, 200 rpm for 6 h (until the bacteria reached the stationary phase). Then, the contents of the dialysis tubing were collected, 100 μL of each suspension (50 μL for Ag NPs) was transferred into 2 mL of ultrapure water, mixed well and measured by UV-vis spectroscopy (Shimadzu, UV-3600 Plus, Tokyo, Japan).
2.11 Inductively coupled plasma-mass spectrometry (ICP-MS)
Ag NP stock in ultrapure water (2 g L−1) was diluted in spent or fresh artificial saliva or THB by first mixing equal volumes of the stock and 2-fold concentrated artificial saliva or THB, then diluting to 100 mg L−1 in the respective media. Further dilutions were made to prepare 1 and 10 mg L−1 Ag NP dispersions in the media. For dissolution experiments, the Ag NP dispersions in the media were incubated for 0, 6 and 24 h at 37 °C and 200 rpm in aerobic/anaerobic conditions to simulate the bacterial exposure conditions to Ag NPs. Anaerobic conditions were achieved by placing the tubes in a closed box containing AnaeroPack-Anaero sachets. After incubation, the samples were centrifuged at 4 °C, 15
000g for 30 min and 200 μL of the supernatant was mixed with 1800 μL of 5% HNO3. The samples were filtered through 0.22 μm microporous membrane and the concentration of Ag+ was measured by inductively coupled plasma mass spectrometry (ICP-MS, Thermo iCAP RQ, Waltham, MA, USA).
2.12 Growth assays of S. salivarius K12 exposed to transformed NPs and food-grade TiO2
To investigate the bioactivity of transformed NPs or food-grade TiO2, growth assays with S. salivarius K12 were conducted. A portion of spent or fresh artificial saliva (4.5 mL), prepared as described in section 2.7, was pipetted into a sterile beaker and mixed with 0.5 mL of NP or food-grade TiO2 stock dispersions (2 mg L−1) to achieve the concentration of 200 mg L−1, and incubated at 37 °C, 200 rpm for 24 h in aerobic conditions. Then, NPs or food-grade TiO2 were used in growth assays as described in section 2.4. Briefly, the transformed NPs or food-grade TiO2 (either in spent or fresh artificial saliva) were diluted to 1, 10, 100 mg L−1 with fresh artificial saliva and inoculated with S. salivarius K12 in a 96-well plate, incubated at 37 °C and sampled at 0, 3, 5, 7, 9 and 11 h to quantify ATP content.
2.13 Statistical analysis
All experiments were performed at least in three replicates and the results were presented as mean ± standard deviation (SD). Statistical analysis was performed using GraphPad Prism. The differences between the groups were assessed by ANOVA, Student's t-test or Tukey's multiple comparison test. The results were considered statistically significant when p ≤ 0.05.
3. Results and discussion
3.1 Characteristics of NPs and food-grade TiO2
Based on electron microscopy, pristine Ag and SiO2 NPs, dispersed in ultrapure water, appeared spherical, despite SiO2 NPs not being clearly visualizable due to lower conductivity and small size (Fig. 1a–d). The average measured size of Ag NPs was 47 ± 24 nm which was similar to the manufacturer-reported size range of 60–80 nm. SiO2 NPs were 20 nm in diameter according to the manufacturer but were confirmed to be 13 ± 4 nm based on TEM (Fig. 1d), appearing mostly unagglomerated in the SEM image (Fig. 1c). The two types of TiO2, i.e., engineered NPs and food-grade TiO2, appeared as very different materials based on SEM and TEM images (Fig. 1e–h). TiO2 NPs were smaller in size (30 ± 8 nm, consistent with the manufacturer-reported 20–40 nm), but mainly agglomerated into larger aggregates (Fig. 1e and f), while food-grade TiO2 was not agglomerated and consisted of polyhedral primary particles with an average size of 115 ± 41 nm (Fig. 1g and h). Based on the manufacturer, the size range of food-grade TiO2 was 200–500 nm, thus, the material had a relatively wide particle size distribution which is in accordance with the previously reported characteristics of food-grade TiO2.29 There was no manufacturer-provided information available, but generally, food-grade TiO2 is composed of ∼98% anatase and ∼2% rutile.42 Also, it is possible that TiO2 particles were coated to improve particle stability as the application of silica, alumina and phosphate coatings has been reported.29,43,44 Here, TEM images indicated possible coatings on the surface of food-grade TiO2 (Fig. 1h).
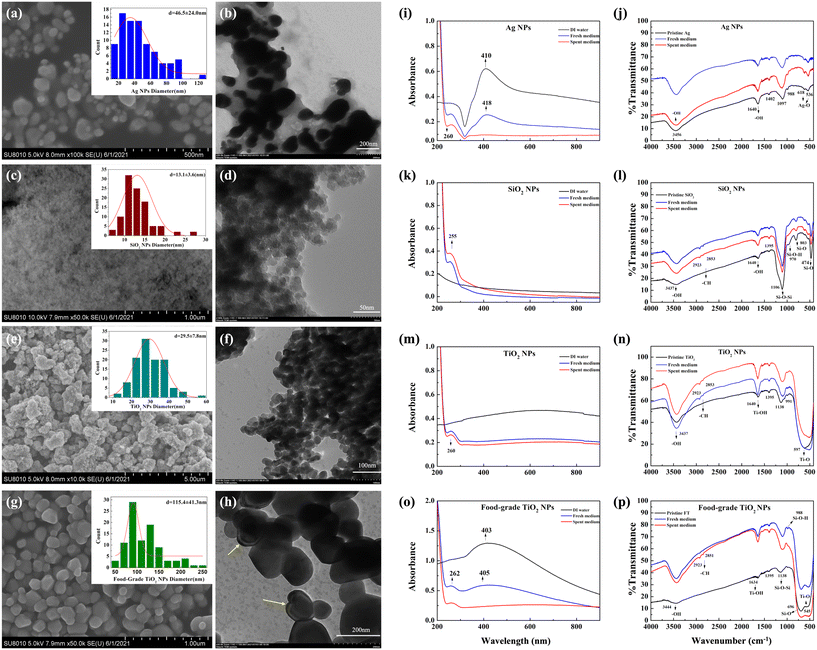 |
| Fig. 1 Characterization of pristine and transformed NPs and food-grade TiO2. Electron microscopy images of (a) Ag NPs (SEM), scale bar = 500 nm; (b) Ag NPs (TEM), scale bar = 200 nm; (c) SiO2 NPs (SEM), scale bar = 1 μm; (d) SiO2 NPs (TEM), scale bar = 50 nm; (e) TiO2 NPs (SEM), scale bar = 500 nm; (f) TiO2 NPs (TEM), scale bar = 100 nm; (g) food-grade TiO2 (SEM), scale bar = 1 μm; and (h) food-grade TiO2 (TEM), scale bar = 200 nm. Size distribution is shown in the insets, where red lines are standard normal distributions. The yellow arrows show the potential coating29 on the food-grade TiO2. Spectra of (i, k, m and o) UV-vis absorption and (j, l, n and p) FTIR obtained from Ag, SiO2 and TiO2 NPs and food-grade TiO2 as pristine particles and after incubating in fresh or spent artificial saliva. | |
When NPs and food-grade TiO2 were dispersed in ultrapure water, the measured hydrodynamic diameters (HDD) indicated that the size of Ag NPs was slightly larger than the pristine size, while SiO2 and TiO2 NPs significantly agglomerated, with Z-average diameters ∼20 and 50–100 times larger than the pristine sizes, respectively (Table 1). The great differences in the size of TiO2 NPs obtained from SEM and DLS may be attributed to the easier aggregation of TiO2 NPs compared to other NPs, which could also be observed from the SEM and TEM images (Fig. 1e and f). However, such agglomeration in ultrapure water was not observed for the food-grade TiO2 which had an HDD similar to its pristine size, in agreement with what has been reported elsewhere.29 The higher dispersion stability of food-grade TiO2 compared to other NPs was also indicated by its lower polydispersity indices (PDI) (Table S2†). The measured ζ-potentials indicated that all materials were negatively charged in ultrapure water (Table 1).
Table 1 Sizes, specific surface areas, hydrodynamic diameters (HDD) and ζ-potentials of nanoparticles and food-grade TiO2 in different media
Nanoparticle |
Size, nm (by manufacturer) |
SSA,a m2 g−1 (by manufacturer) |
HDD, nm (ζ-potential, mV) in ultrapure water |
HDD, nm (ζ-potential, mV) in rich growth medium (THB) |
HDD, nm (ζ-potential, mV) in artificial saliva |
HDD,b nm (ζ-potential, mV) in spent artificial saliva |
Specific surface area measured by Brunauer–Emmett–Teller analysis, reported by the manufacturer.
Spent artificial saliva refers to the medium that was inoculated with S. salivarius K12, incubated at 37 °C, 200 rpm for 18 h, then centrifuged at 12 000g for 10 min to remove bacteria. NA – not available.
|
Ag |
60–80 |
≥3 |
98 ± 2 |
505 ± 6 |
417 ± 30 |
3471 ± 746 |
(−53 ± 2) |
(−4 ± 1) |
(−12 ± 0.3) |
(−5 ± 0.5) |
SiO2 |
20 |
145–160 |
367 ± 39 |
747 ± 48 |
154 ± 3 |
1065 ± 26 |
(−16 ± 2) |
(−5 ± 0.8) |
(−8 ± 2.5) |
(−4 ± 0.3) |
TiO2 |
20–40 |
77 |
1950 ± 23 |
5028 ± 503 |
1129 ± 75 |
2528 ± 11 |
(−39 ± 2.7) |
(−3 ± 0.5) |
(−11 ± 0.4) |
(−4 ± 0.7) |
Food-grade TiO2 |
200–500 |
NA |
205 ± 9 |
568 ± 10 |
197 ± 2 |
2098 ± 181 |
(−5 ± 3) |
(−6 ± 1) |
(−8 ± 0.5) |
(−6 ± 1) |
When NPs and food-grade TiO2 were dispersed in the rich growth medium (THB), the HDD indicated significant agglomeration for all materials and the ζ-potentials of NPs were close to zero, suggesting instability of the dispersions. This is expected due to the high ionic strength and protein content of the medium which facilitates interactions between NPs.26 In artificial saliva, the HDDs of SiO2 and TiO2 NPs and food-grade TiO2 were smaller than their HDDs in THB and ultrapure water, however, HDD of Ag NPs was similar in artificial saliva and THB. Although THB contained higher levels of nutrients (derived from beef heart infusion, yeast extract and pancreatic digest of casein) than artificial saliva, the latter included, in addition to meat extract, also mucin, high-molecular-weight heavily glycosylated proteins, which are the major macromolecular components of mucus. TiO2 NPs have been shown to strongly interact with mucin, which adsorbs to NP surface and reduces the aggregation of NPs.25 Interactions with mucin may have also influenced the aggregation behavior of other NPs in our study as salivary conditions and saliva components have been shown to affect also Ag and SiO2 NPs.24,45 In addition, more negative ζ-potential values in artificial saliva than in THB (Table 1) confirmed higher stability of NPs in artificial saliva.
3.2 Transformation of NPs and food-grade TiO2 in spent artificial saliva
The major factors that affect the bioeffects of food-related NPs are the interactions with biomolecules in the physiological solutions and food matrix or bacterial secreted compounds. The transformations of food-relevant NPs in the simulated gastrointestinal fluids have been investigated in several studies.24,25,46,47 It has been shown that in simulated oral digestion step NP properties are relatively less modified than in the gastric or intestinal phases,46,47 while protein corona formation on NPs in saliva has been shown to be NP type-dependent and potentially affect the digestive and antimicrobial properties of saliva.48 However, previous studies have not considered the combined effects of the salivary environment and bacterial secreted compounds on NP transformation.
Here we assessed the physico-chemical changes of food-related NPs and food-grade TiO2 upon incubation in spent artificial saliva that contained bacterial secreted compounds. Significant agglomeration of NPs and food-grade TiO2 was evident in the spent artificial saliva compared to fresh artificial saliva where the HDD of the tested materials were similar to those in ultrapure water (Table 1). In addition, lower stability of NP dispersions in spent artificial saliva was corroborated by close-to-zero ζ-potential values, which may be due to the change in pH during bacterial growth, where pH decrease causes increased ζ-potential.49 Thus, bacterial secreted compounds considerably increased particle agglomeration which highlights the importance of taking into account the effects of microbial compounds when simulating digestive tract conditions. The agglomeration of NPs to micrometer-sized particles was also reflected by the UV-vis spectra where the NP-characteristic absorbance peaks (410 nm for Ag NPs and 403 nm for food-grade TiO2) did not appear after incubation of NPs in spent artificial saliva (Fig. 1i and o). The NP-characteristic peaks were red-shifted in fresh artificial saliva compared to the peaks in ultrapure water, indicating slight agglomeration or particle protein-coating in the artificial saliva. The UV-vis absorbance spectra of SiO2 and TiO2 NP dispersions in ultrapure water did not display NP-specific peaks, however, all spectra indicated typical protein absorbance peaks at ∼260 nm (corresponding to aromatic amino acids),50 suggesting that NPs were associated with proteins after incubation in fresh and spent artificial saliva (Fig. 1i, k, m and o).
NP association with biomolecules was also reflected in the FTIR spectra. Compared to the spectra of pristine NPs and food-grade TiO2 the spectra of artificial saliva-incubated materials showed peaks characteristic of biological compounds: methyl (C–CH3) and methylene (CH2) at 2923 and 2853 cm−1, respectively, and –C
O and N–H stretching vibrations at 1634 and 1395 cm−1, respectively (Fig. 1j, l, n and p).51 The peak at 1640 cm−1 in the spectra of pristine materials was assigned to OH vibrations52 and this could have overlapped with the –C
O peaks in the spectra of the artificial saliva-incubated materials. The FTIR spectra of food-grade TiO2 showed absorption peaks at 1634 and 545 cm−1 for Ti–OH and Ti–O bonds, in addition to absorption peaks at 1138, 988 and 696 cm−1, indicating the presence of Si–O–Si bond stretching vibrations, Si–OH vibrations and Si–O bonds, respectively.52 It implies that the coating observed in TEM images of the food-grade TiO2 (Fig. 1h) may consist of silicon, which is consistent with literature that has reported P, Al and Si in food-grade TiO2.29 SDS-PAGE also indicated that SiO2 and TiO2 NPs and food-grade TiO2 were coated with proteins, appearing mainly as bands at 10–20 kDa (Fig. S3†). Differently from other NPs, Ag appeared to be associated with no or fewer proteins based on the less intensive bands in the gel, which was consistent with the FTIR spectra that did not indicate biomolecule-characteristic bonds associated with Ag NPs after incubation in artificial saliva (Fig. 1j). The SDS-PAGE analysis of supernatants after NP and food-grade TiO2 removal indicated that fresh artificial saliva contained considerably higher amounts of unbound proteins than spent artificial saliva, suggesting that bacteria had consumed or degraded the proteins in the medium (e.g., by secreting proteases and acidifying the pH of the medium) during the growth process. The major source of proteins in artificial saliva was meat extract added as a representative meal. Also mucin that was added to artificial saliva could have been used as a nutrient by bacteria. These are heavily glycosylated proteins and thus would be difficult to visualize in the SDS-PAGE gel. In spent artificial saliva, there would also be bacterially secreted compounds, including salivaricins, that would need to be present at micromolar levels to be effective against other bacteria. Thus, the concentration of salivaricins in spent media would be below the detection limit of SDS-PAGE gel visualization and would also be outside the size range of the molecular weight standard (10–180 kDa) used here with the molecular weights of SalA and SalB being 2.4 and 2.7 kDa, respectively.53
In summary, the HDD measurements and UV-vis spectra indicated differences in the physico-chemical properties of NPs and food-grade TiO2 in fresh and spent artificial saliva, illustrating the effects of bacterial compounds. However, FTIR and SDS-PAGE were not sensitive enough to distinguish the differences in protein adsorption patters of NPs and food-grade TiO2 in fresh and spent artificial saliva and additional analysis is needed to assess the interactions between bacterial metabolites and NPs.
3.3 Growth of S. salivarius K12 in rich growth medium and artificial saliva, aerobic and anaerobic conditions
S. salivarius K12 is a facultative anaerobe, which means that it can grow in both aerobic and anaerobic environments. S. salivarius K12 has mainly been found to colonize the human oral environment, where aerobic conditions prevail, but the strain has also been detected in the small intestine where conditions are microaerophilic.20 To be able to assess the effects of NPs and food-grade TiO2 on S. salivarius K12 in both aerobic and anaerobic conditions, bacterial growth was evaluated at both oxygen levels and growth parameters in artificial saliva were compared to these in the conventional rich growth medium (THB).
In THB, no significant difference in the specific growth rates of S. salivarius K12 was detected in aerobic and anaerobic conditions (1.21 ± 0.03 h−1 and 1.26 ± 0.03 h−1, respectively) (Fig. 2a). Due to the high turbidity of artificial saliva, assessment of bacterial growth based on OD600 was not feasible and ATP concentrations were measured instead. ATP-based growth assessment of bacterial cultures has been successfully applied previously32,33 and the suitability of the approach was also validated for S. salivarius K12, demonstrating that ATP concentrations and OD600 values, measured during the early exponential growth phase (Fig. S1a†), correlated positively (Fig. S1b†). Also, the specific growth rates of S. salivarius K12, calculated based on the OD600 values and ATP concentrations were not significantly different (Table S3†). At late-exponential and stationary growth phases, a decrease in ATP concentrations occurred (Fig. S1a†), which could be explained by the reduced requirement for protein synthesis once the cells enter the stationary phase.54
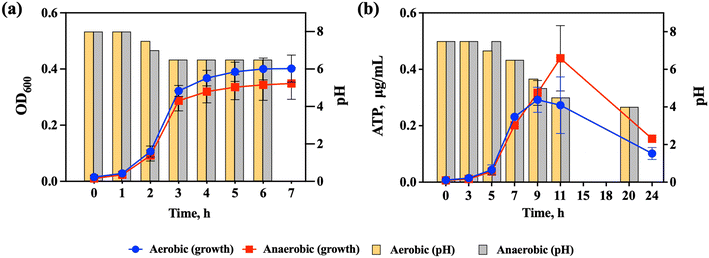 |
| Fig. 2 Growth of S. salivarius K12 and pH changes in the medium during growth (a) in the rich growth medium (THB) and (b) in artificial saliva in aerobic and anaerobic conditions. The data points are the average of 3 replicate experiments each conducted in triplicate and the error bars indicate standard deviations. When error bars are not visible, the standard deviations were small and the error bars are hidden by the data points. | |
Similarly to the growth in THB, there was no significant difference in the specific growth rates of S. salivarius K12 in aerobic and anaerobic conditions in artificial saliva (0.55 ± 0.04 h−1 and 0.47 ± 0.01 h−1, respectively) (Fig. 2b). Compared to growth in THB, the specific growth rates in artificial saliva were significantly lower than in THB and the late exponential phase was reached in ∼9–10 h compared to 3 h in THB which was expected due to a lower level of nutrients in artificial saliva. Still, artificial saliva supported the growth of S. salivarius K12 allowing simulation of its natural ecological niche. After 9–10 h of growth in artificial saliva, the ATP concentrations decreased (Fig. 2b), which signified the start of the late exponential or stationary phase, based on the analogy with the cultures grown in THB (Fig. S1a†). Another indication of the culture reaching the late exponential or stationary phase was the significant downward shift in the pH of the culture medium (from the initial pH 7.5 to pH 4 at 20 h; Fig. 2b), which was a considerably larger pH change than in THB (from the initial pH 8 to pH 6.5 after 3 h; Fig. 2a). Consistent with similar growth, there were no significant differences in pH changes in aerobic and anaerobic conditions in either THB or artificial saliva. It has been determined that the growth of S. salivarius K12 is uncoupled from lactic acid synthesis when the culture reaches the late exponential phase in artificial saliva, meaning that even when the growth stops the cells continue to consume sugar to produce lactic acid.31 This was different from the cultures in the rich growth medium where no uncoupling was detected, which suggested that growth arrest in artificial saliva was necessary for the cells to tolerate high concentrations of lactic acid.31 Thus, the growth of S. salivarius K12 in artificial saliva provides a test system for NP bioeffect assessment that is considerably different from the bacteria cultivated in the rich growth medium, allowing simulation of bacterial physiological conditions prevailing in their natural habitat, the human oral cavity.
3.4 Effects of NPs and food-grade TiO2 on the growth of S. salivarius K12 in artificial saliva and rich growth medium at aerobic and anaerobic conditions
In the rich growth medium (THB), both in aerobic and anaerobic conditions, the specific growth rates of S. salivarius K12 exposed to NPs or food-grade TiO2 at concentrations 1, 10 and 100 mg L−1 were similar, without significant difference from the specific growth rate of the untreated control (Fig. 3a and b). However, Ag NPs tended to slightly inhibit the specific growth rate, while SiO2 NPs tended to stimulate the specific growth rate, although not significantly. Differently, in artificial saliva, which mimicked physiological conditions, Ag NPs significantly inhibited the specific growth rate of the bacteria both in aerobic and anaerobic conditions dose-dependently (Fig. 3c and d). While there was a slight trend for TiO2 NPs to promote bacterial growth in aerobic conditions, it was not statistically significant, and there was no effect on growth in anaerobic conditions. SiO2 NPs and food-grade TiO2 NPs did not impact bacterial growth in artificial saliva regardless of the oxygen levels. Consistent with previous studies,7,55 SiO2 and TiO2 NPs are not expected to inhibit bacterial viability or growth in physiologically relevant conditions, i.e., in the dark where photoactivation does not occur. However, it has been shown that Gram-positive bacteria may be more susceptible to SiO2 (ref. 55) than Gram-negative bacteria which is relevant when considering NP effects on human microbiota where the majority of beneficial bacteria are Gram-positive.
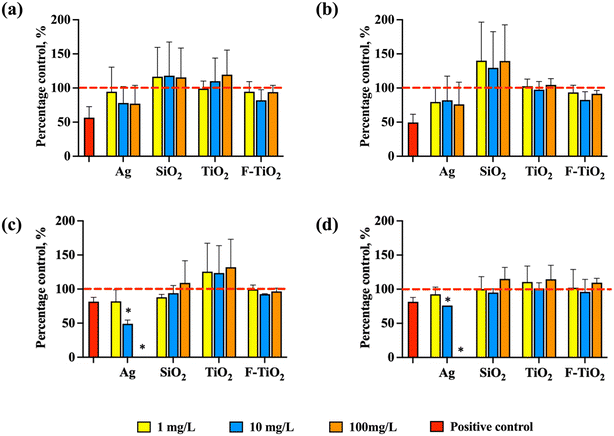 |
| Fig. 3 Normalized specific growth rates of S. salivarius K12 in aerobic and anaerobic conditions when exposed to Ag, SiO2 or TiO2 NPs or food-grade TiO2 (F-TiO2) at 1, 10, 100 mg L−1. The specific growth rates of the untreated control bacteria were designated as 100% (marked with red dashed lines). Growth in (a) rich growth medium (THB) in aerobic conditions, (b) rich growth medium (THB) in anaerobic conditions, (c) artificial saliva in aerobic conditions, and (d) artificial saliva in anaerobic conditions. AgNO3 at 1.25 mg L−1 (in THB) and 0.0125 mg L−1 (in artificial saliva), inhibiting bacterial growth by ∼50% or ∼20%, respectively, was used as a positive control. The concentrations of AgNO3 were selected so that the growth inhibition would be in the range of 20–80% in each medium (Fig. S2†). The data bars are the average values of four replicates and error bars indicate standard deviations. * – significantly different from the untreated control. | |
Interestingly, in the rich growth medium, even the highest tested concentration of Ag NPs (100 mg L−1) did not inhibit significantly the growth of S. salivarius K12 in either aerobic or anaerobic conditions but was 100% growth-inhibitory in artificial saliva (Fig. 3c and d). Ag NPs are known to exert their antimicrobial effects mainly through two mechanisms, including contact- and ion-mediated killing.56,57 Ion-mediated killing exerts its antimicrobial effect mainly through Ag+,58 thus the quantities of released Ag+ from Ag NPs dispersed in THB and artificial saliva were determined after incubation in identical conditions as bacterial growth assays (but excluding bacteria), to reveal if the growth-inhibitory effects of Ag NPs could be correlated with the shed Ag+ in different media. Indeed, the measured concentrations of dissolved silver in 100 mg L−1 Ag NP dispersions at 0 h were 100-fold higher in artificial saliva than in THB (0.06 mg L−1versus 0.0006 mg L−1, Fig. 4a and d) which could have explained the growth inhibition in artificial saliva (Fig. 3c and d). Indeed, in THB, AgNO3 was not growth-inhibitory to S. salivarius K12 at up to 0.625 mg L−1 (corresponding to 0.4 mg L−1 Ag+, above the level of dissolved Ag+ in 100 mg L−1 Ag NP dispersion in THB) (Fig. S2a and b†), while ten-fold lower levels of AgNO3 (starting from 0.0625 mg L−1) which corresponded to 0.04 mg L−1 Ag+, similar to the level of dissolved Ag+ in 100 mg L−1 Ag NP dispersion in artificial saliva, were 100% growth-inhibitory in artificial saliva (Fig. S2c and d†). Overall, the concentration of Ag+ in Ag NP dispersions in THB remained below 0.001 mg L−1 at all time points and NP concentrations (Fig. 4a–c), explaining the lack of antibacterial action in THB (Fig. 3a and b). The increased dissolution of Ag NPs in artificial saliva compared to THB was significant at 10 and 100 mg L−1 of Ag NPs (Fig. 4). Higher levels of Ag+ in artificial saliva than THB were likely caused by the differences in the compositions of the two media; THB contained high levels of nutrients and salts that could have either stabilized Ag NPs or facilitated transformations of Ag+ to insoluble Ag species (e.g., metallic Ag or AgCl), resulting in lower Ag+ concentrations. In anaerobic conditions, lower Ag NP dissolution in THB was evident only in the case of 100 mg L−1 Ag NPs (Fig. 4b and c), while in artificial saliva the concentration of dissolved silver was lower in anaerobic compared to aerobic conditions at all tested NP concentrations (Fig. 4e and f). This was expected due to reduced oxidative dissolution in the lack of oxygen and was consistent with the lower toxicity of Ag NPs in the artificial saliva in anaerobic, compared to the aerobic environment (Fig. 3c and d). In agreement with our study, the toxicity of Ag NPs to E. coli was higher in aerobic conditions than in anaerobic conditions,59 likely due to lower dissolution of Ag NPs in the oxygen-depleted environment. It is plausible that Ag NPs also induce particle-specific effects in bacteria as reported by others,60 but clearly, dissolved Ag+ correlated with the observed toxicity to S. salivarius K12 in artificial saliva.
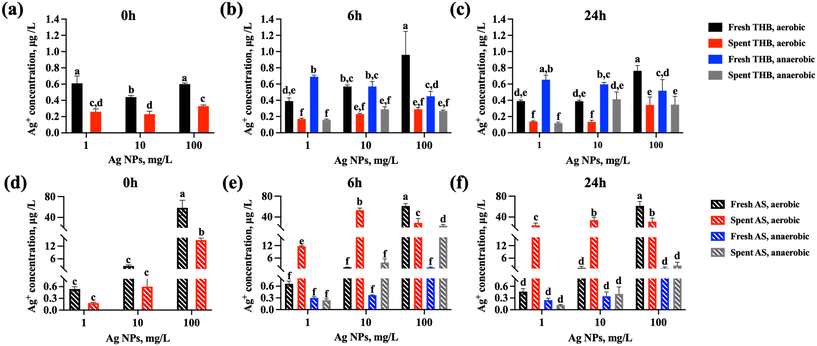 |
| Fig. 4 Concentrations of Ag+ after incubating Ag NPs at different concentrations in fresh and spent rich growth medium (THB) and in artificial saliva (AS) at 37 °C, 240 rpm for 0, 6 or 24 h. Concentrations of Ag+ in (a) THB in aerobic conditions at 0 h, (b) THB in aerobic and anaerobic conditions at 6 h, (c) THB in aerobic and anaerobic conditions at 24 h, (d) artificial saliva in aerobic conditions at 0 h, (e) artificial saliva in aerobic and anaerobic conditions at 6 h, (f) artificial saliva in aerobic and anaerobic conditions at 24 h. The concentrations at 0 h reflect the Ag concentrations in the media immediately after diluting Ag NP stock in either THB or artificial saliva, in aerobic conditions. The data bars are the average values of 3 replicate samples and error bars indicate standard deviations. Different letters indicate significantly different (p < 0.05) data, the same letters indicate no significant difference. | |
Other factors that influence the dissolution, and thus, the toxicity of Ag NPs include the medium pH and NP interactions with the bacterial cells. It has been reported that cell-NP contact increased the bacterial uptake of NP-associated Ag ions that caused antibacterial action.61 This effect could not be accounted for in the Ag NP dissolution measurements conducted in the culture medium (without bacteria present), but bacterial effects on the Ag NP dissolution could be simulated by determining Ag NP dissolution in the spent media, i.e., media where bacteria had been cultured. In spent media, the dissolution trends were similar as in fresh media: artificial saliva contained significantly higher concentrations of Ag+ than THB (Fig. 4). However, spent THB contained less Ag+ than fresh THB at all NP concentrations and time points (Fig. 4a–c), which could be attributed to the presence of bacterial metabolites that could act as reducing agents of Ag+, forming secondary zerovalent Ag NPs or biomolecule bound silver compounds, thus reducing the “free” Ag+ levels in the media. The dissolved Ag ions from Ag NPs have been shown to be subject to transformations in biological media and physiologically relevant conditions.62,63 However, in artificial saliva, Ag NP dissolution at 1 and 10 mg L−1 was increased in spent compared to fresh medium (Fig. 4e and f). This could be explained by the acidification of the medium by bacteria (pH 4 at the end of culturing, Fig. 2). It has been demonstrated that bacterial acidification of the medium promotes the solubilization of Ag NPs, which leads to the accumulation of Ag ions.64 Higher Ag+ levels in spent artificial saliva than in spent THB could have been also due to lower levels of bacterial metabolites in artificial saliva because the bacterial growth was slower in the latter. Thus, there may have been less bacterial metabolites in spent artificial saliva to transform the released Ag+ into insoluble or particulate Ag compounds as likely happened in spent THB. Still, as expected, the dissolution of Ag NPs in spent artificial saliva was reduced in anaerobic conditions compared to aerobic conditions. Overall, Ag NP dissolution in spent media confirmed that the reasons that led to no growth inhibition at high Ag NP concentrations (100 mg L−1) in rich growth medium (THB) and 100% growth inhibition in artificial saliva could be due to higher Ag+ dissolution in artificial saliva and possibly also the higher protein content of THB that may bind Ag+ and make them less bioavailable. This makes Ag NPs more likely to agglomerate or form a protein coating on their surface and have higher chemical stability compared to exposure to artificial saliva with slightly less protein content. It has been demonstrated previously that NPs exhibit higher chemical stability in simulated tear fluid containing proteins compared to the fluid without proteins and this further affected the antimicrobial effect of NPs.26 In the oral cavity, saliva is a major component in maintaining oral health, and direct exposure of NPs to the oral salivary environment is unavoidable. The higher antimicrobial activity of Ag NPs in artificial saliva may inhibit or kill beneficial bacteria such as S. salivarius K12, disrupting the balance of the oral microbiota and thus leading to the development of some oral diseases.
To test if the transformation of NPs and food-grade TiO2 after incubation in artificial saliva also affected their bioactivity, their growth-inhibitory effects on S. salivarius K12 were assessed. Interestingly, unlike the pristine Ag NPs, the transformed Ag NPs at 10 mg L−1 did not inhibit the growth of bacteria in aerobic conditions (Fig. S4a†). The growth-inhibitory effect had reduced from ∼50% to no inhibition. Since it was established that the toxicity of Ag NPs correlated with its solubility, the reduced toxicity could be linked to lower release of Ag+ due to Ag NP agglomeration in spent artificial saliva (Table 1) and thus, reduction of the specific surface area. Conversely, increased antibacterial activity was demonstrated for Ag NPs transformed in simulated human tear fluid, due to increased dissolution of transformed Ag NPs.26 In the same study, however, the antibacterial efficiency of ZnO NPs was reduced in the simulated tear fluid, suggesting NP-specific transformations, in addition to physiological fluid properties, playing a role in the modification of NP bioeffects. The increased toxicity of transformed NPs was partially attributed to acquiring protein coating in the simulated tear fluid. Here, physico-chemical characterization indicated that SiO2 and TiO2 NPs and food-grade TiO2 were associated with proteins from artificial saliva. This could explain the increased antibacterial activity of these food-related NPs after transformation when added to S. salivarius K12 cultures at 100 mg L−1 (Fig. S4b–d†). It has been shown that NP interactions with natural organic matter (NOM) and macromolecular compounds such as proteins affect their bioavailability;65,66 also, increased toxicity has been observed due to the formation of protein coronae on the surface of NPs in physiological fluids.67 However, it was confirmed that the spent medium itself did not exert any growth-inhibitory effect to S. salivarius K12 (Fig. S5†).
3.5 Effects of NPs and food-grade TiO2 on the salivaricin gene expression of S. salivarius K12
Despite the lack of acute toxicity (no growth inhibition) of SiO2 and TiO2 NPs and food-grade TiO2, the impacts of food-grade NPs on beneficial bacteria can be more subtle. These subtle changes can be detected at the gene expression level as demonstrated before.32,33 Since salivaricins secreted by S. salivarius K12 are important metabolites that ensure the competitiveness of these bacteria in the microbial communities such as human microbiota and may also play a role in the immunoprotective properties of the bacteria in humans,22,68 the expression of salivaricin-encoding genes by S. salivarius K12 upon exposure to NPs and food-grade TiO2 was quantified. As 10 mg L−1 is relevant for human daily oral exposure to food-grade NPs,18,69 and both 1 and 10 mg L−1 of SiO2 and TiO2 NPs and food-grade TiO2 were sub-growth-inhibitory (Fig. 3), these two exposure concentrations of NPs were used in gene expression experiments. Ag NPs were included at 1 and 10 mg L−1 which were higher than relevant human exposure concentrations, however bacterial growth was not affected at these concentrations in THB, thus, similarly to other tested NPs, sub-growth-inhibitory conditions were employed.
S. salivarius K12 can produce at least two different bacteriocins, salivaricin A (SalA) and salivaricin B (SalB). Both salivaricins are encoded by megaplasmid-located genes which are organized in loci containing genes for the production, modification, transport and regulation of each salivaricin.70 In their respective multi-gene loci, salA and sboA are the structural genes of SalA (2368 Da peptide) and SalB (2733 Da peptide), respectively. In SalB-encoding gene locus, sboK encodes a histidine kinase that has a role in recognizing the induction signals that activate the transcription of SalB structural gene sboA. sboG belongs to the cluster of genes in SalB locus that encode immunity proteins, necessary for the self-protection of S. salivarius K12 against its own synthesized salivaricin.53 The effects of NPs and food-grade TiO2 were assessed on the transcription of the two salivaricin structural genes (salA and sboA) as well as the genes associated with the signal transduction and immunity of SalB (sboK and sboG). The gene expression was first quantified comparatively in aerobic and anaerobic conditions after 6 h cultivation. In aerobic conditions, most of the NPs at the tested concentrations upregulated the structural genes of SalA and SalB (Fig. 5a and b). Interestingly, Ag NPs significantly upregulated SalB, but not SalA expression while food-grade TiO2 induced SalA and, depending on concentration, either downregulated or did not affect the expression of SalB. The expression patterns of the signal transduction- and immunity-related genes of SalB (sboK and sboG) followed the same pattern as the expression of the structural gene sboA, suggesting that the transcription and expression of the full SalB locus were stimulated by the food-related NPs. Since food-grade TiO2 did not induce the structural gene sboA, consistently, it did not induce the signal-transduction gene (sboK) or immunity gene (sboG) either (Fig. 5b).
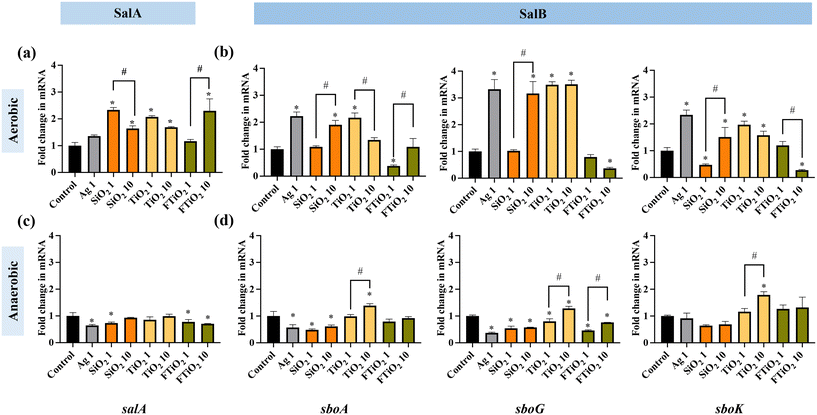 |
| Fig. 5 Bacteriocin gene expression in S. salivarius K12 upon exposure to NPs (1 mg L−1 Ag NPs, 1 or 10 mg L−1 of SiO2 and TiO2 NPs and food-grade TiO2) for 6 h in (a and b) aerobic and (c and d) anaerobic conditions, compared to the respective gene expression in unamended control bacteria. FTiO2 – food-grade TiO2, the numbers in the x-axis labels (1 or 10) indicate the concentrations (mg L−1) of different NPs. The data bars are the average values of three biological replicates, error bars indicate standard deviations. * – significantly different from the control; # – within-group differences. | |
In contrast to the aerobic environment, in anaerobic conditions, NPs and food-grade TiO2 either downregulated or did not affect the transcription of the bacteriocin structural genes (Fig. 5c and d). Differently from other NPs, TiO2 NPs at 10 mg L−1 stimulated SalB expression, reflected by upregulation of sboA, sboG as well as sboK. Based on the gene expression results it is plausible to suggest that, in anaerobic conditions, food-related NPs, except for TiO2, may potentially inhibit the secretion of bacteriocins and that TiO2 NPs may have different effects on S. salivarius K12 than other NPs.
Considering that the main habitat of S. salivarius K12 is the oral environment and upper digestive tract where aerobic conditions prevail, the assessment of time-evolution of salivaricin gene regulation upon exposure to NPs and food-grade TiO2 was conducted in aerobic conditions. Different exposure times of bacteria to NPs (1, 4 or 18 h) also simulated short-term and chronic human exposure to food-grade NPs. The results indicated that short-term exposure (1 h) to NPs and food-grade TiO2 suppressed bacteriocin structural gene expression both for SalA and SalB (Fig. 6a and b). Also the transcription of the immunity-related gene (sboG) did not change or was downregulated while there was downregulation of the transcription regulatory gene (sboK), consistent with no induction of the SalB structural gene. Interestingly, food-grade TiO2, especially at 10 mg L−1, exerted a more pronounced suppressing effect on salivaricin gene expression than TiO2 NPs, suggesting different bioeffects of the two TiO2 materials. After 4 h of growth in the presence of NPs, there were mostly no significant differences in the expression of SalA compared to the expression in the unamended control culture (Fig. 6c). However, at 10 mg L−1, TiO2 NPs significantly increased SalB structural gene expression while food-grade TiO2 had an opposite effect at the same concentration (Fig. 6d). While Ag NPs did not affect the structural gene transcription of SalB (sboA), the signal-transduction and immunity-related genes were upregulated, possibly suggesting different time-evolution in the transcription of these genes due to regulatory feedback loops as salivaricin synthesis is autoregulated by sensing the active salivaricin molecules in the extracellular environment.53 After long-term exposure (18 h), NPs and food-grade TiO2 significantly upregulated the gene expression of SalA, but there was almost no significant difference in the gene expression of SalB, with the exception of TiO2 NPs at 10 mg L−1 which also induced SalB at 18 h, similar to its action after 4 h exposure (Fig. 6e and f). On the other hand, food-grade TiO2 did not affect SalB structural gene transcription, however, its induction-related gene was significantly downregulated at 10 mg L−1 exposure concentration, contrarily to the significant stimulation of the same gene by TiO2 NPs (Fig. 6f). Although TiO2 NPs and food-grade TiO2 seemed to exert opposing effects on the transcription of SalB, both upregulated SalA gene significantly at 1 mg L−1, which was different from the effects of Ag and SiO2 NPs that impacted SalA expression only at 10 mg L−1. Time evolution of gene expression is little studied so far but would provide important insight into the effects of short- and long-term exposures to NPs via ingestion.
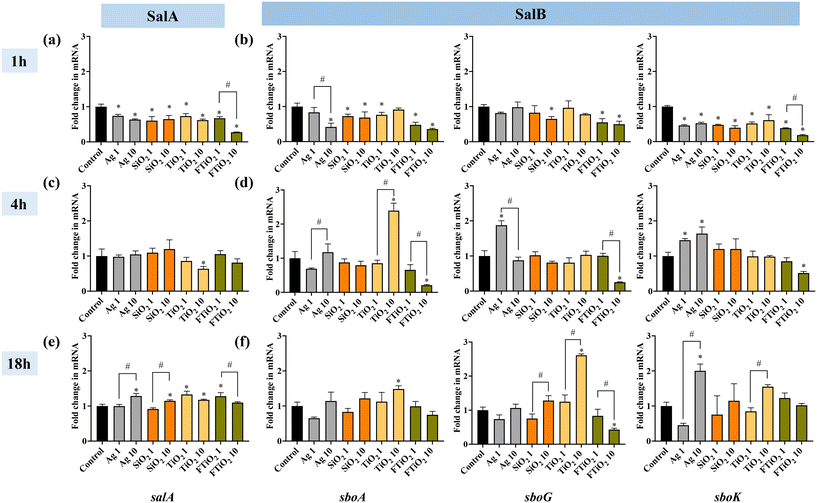 |
| Fig. 6 Bacteriocin gene expression in S. salivarius K12 upon exposure to NPs (1 or 10 mg L−1 of Ag, SiO2 and TiO2 NPs and food-grade TiO2) for 1, 4, or 18 h in aerobic conditions, compared to the respective gene expression in unamended control bacteria. FTiO2 – food-grade TiO2, the numbers in the x-axis labels (1 or 10) indicate the concentrations (mg L−1) of different NPs. (a, c and e) expression of SalA gene and (b, d and f) expression of SalB genes (sboA, sboG, sboK). The data bars are the average values of three biological replicates, error bars indicate standard deviations. * – significantly different from the control; # – within-group differences. | |
3.6 Effects of NPs and food-grade TiO2 on the antagonistic activity of S. salivarius K12
The gene expression analysis indicated upregulation of SalA gene upon NP and food-grade TiO2 exposures over longer time scales and in aerobic conditions. However, changes in gene expression do not always translate to physiological effects. Thus, we next sought to answer the question of whether the transcription-level effects manifested physiologically. Therefore, the antagonistic activity of S. salivarius K12 against the indicator strain M. luteus after exposure to NPs and food-grade TiO2 at 10 mg L−1 was measured. The diameters of the inhibition zones indicated that longer-term exposure (14–22 h) to SiO2 or TiO2 NPs or food-grade TiO2 significantly stimulated the antagonistic activity of S. salivarius K12 (Fig. 7). This was consistent with the upregulation of SalA gene expression upon 18 h incubation with NPs or food-grade TiO2 (Fig. 6e and f). Similarly, consistent with the gene expression regulation, there was no significant change in the antagonistic activity, compared to unamended control, after exposure to NPs or food-grade TiO2 for a shorter time (7 or 10 h), with the exception of SiO2 NPs that stimulated antibacterial activity after a 7 h exposure. Although Ag NPs induced SalA gene expression after 18 h exposure at 10 mg L−1, the antagonistic activity of S. salivarius K12 appeared inhibited by Ag NPs which may be caused by the growth-inhibitory effect of Ag NPs on S. salivarius K12 at this concentration in aerobic conditions (Fig. 7). The inhibition zones of the indicator strain M. luteus on agar were attributed to the antibacterial compounds secreted by S. salivarius K12 and not the NPs that could have been co-transferred on agar with S. salivarius K12. The reason was that the increased inhibition zones were observed in the case of NPs that were not growth-inhibitory (SiO2 and TiO2 NPs and food-grade TiO2) and decreased inhibition zones occurred at the spots of S. salivarius K12 exposed to Ag NP, i.e., growth-inhibitory NPs. Thus, the observed effects on M. luteus were the opposite to the expected NP effects on bacterial growth and NPs were presumed not to contribute to the results of the deferred antagonism assay.
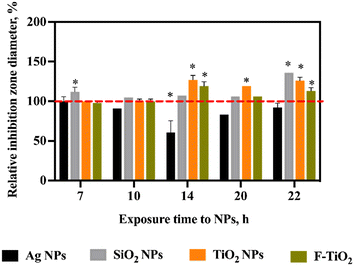 |
| Fig. 7 Antagonistic activity of S. salivarius K12 against the indicator strain M. luteus after exposure of S. salivarius K12 to NPs (10 mg L−1) in artificial saliva for different durations, aerobically, compared to the antagonistic activity of the untreated control bacteria (designated as 100%) (deferred antagonism assay). FTiO2 – food-grade TiO2. | |
Overall, the gene expression changes induced by NPs and food-grade TiO2 were consistent with the effects at the physiological level. Since SalB gene was mostly unaffected (Fig. 6f), it appears that secretion of SalA conferred S. salivarius K12 more potent antagonistic activity compared to unamended control bacteria. The reason for the bacteria to harbor genes for different salivaricins in the genome is to broaden the antagonistic efficiency against different target species because salivaricins differ by their antibacterial spectra.53 Thus, if food-related NPs affect specific types of salivaricins and not others, the antibacterial spectrum of the beneficial bacteria may change and consequently influence the microbial community composition and balance.
4. Conclusions
Here, we demonstrated that artificial saliva with a representative meal can serve as a physiologically relevant test medium for assessing the impacts of food-relevant NPs and food-grade TiO2 on human commensal bacteria. The effects of NPs (specifically Ag NPs) on bacterial growth in artificial saliva were shown to be more pronounced than in the rich growth medium, suggesting that physiologically relevant media and exposure conditions deserve further exploration and possible implementation in the NP safety assessment. Factors such as lack or presence of oxygen, pH and media composition strongly affected the dissolution of Ag NPs and aggregation and protein coating of SiO2 NPs, TiO2 NPs and food-grade TiO2. Importantly, bacterial growth in artificial saliva influenced the physico-chemical transformations of NPs, highlighting the potential role of microbiota in changing the properties, fate and bioeffects of food-added NPs at the entry portal of ingested NPs – the oral cavity.
Despite of not being acutely toxic (growth-inhibitory) to S. salivarius K12, SiO2 and TiO2 NPs and food-grade TiO2 significantly affected the transcription of bacteriocins and the antagonistic activity of the bacteria. The gene expression regulation was time-dependent and different in aerobic and anaerobic conditions. Long-term exposure to NPs and food-grade TiO2 in the aerobic environment stimulated bacteriocin gene expression. Increased bacteriocin production upon exposure to organic NPs has been previously demonstrated with a probiotic bacterium Pediococcus acidilactici from the order Lactobacillales and the underlying mechanism was proposed to be associated with the induction of mild stress by NPs that activated the bacterial defense system and led to increased production of bacteriocin.71 Since, in light of the increasing development of antimicrobial resistance to conventional antibiotics, utilization of bacteriocins as alternative antibiotics has received heightened attention,21 certain NPs could potentially be of interest as prebiotics to stimulate bacteriocin secretion by probiotic bacteria. However, the current study demonstrated that food-relevant NPs can either suppress or stimulate bacteriocin transcription, depending on the conditions, thus, exerting varying and unpredictable impacts on commensal bacteria. Further, gene expression indicated that NP effects on the two types of bacteriocins encoded in the genome of S. salivarius K12 (SalA and SalB) were different. Considering that the spectrum of target species of each bacteriocin does not fully overlap, NPs may impact the competitiveness of S. salivarius K12 in oral microbiota. To better understand the potential impacts of food-related NPs on inter-species communication and bacterial metabolism in human microbiota, future studies, making use of comprehensive transcriptome, proteome and metabolome screening with omics approaches,72,73 are needed.
This study established that food-related engineered NPs and food-grade TiO2 at relevant concentrations for human daily oral exposure to food-grade NPs did not inhibit the growth of oral commensal bacteria but significantly affected bacteriocin gene expression and antagonistic activity of the bacteria, potentially impacting their competitiveness in oral microbiota. Importantly, food-grade TiO2 and engineered TiO2 NPs had opposite effects on the bacteriocin gene expression as well as different physico-chemical properties in artificial saliva, highlighting the importance of including food-grade materials in the risk assessment of NPs.
Conflicts of interest
There are no conflicts to declare.
Acknowledgements
Funding from the National Natural Science Foundation of China (Research Fund for International Excellent Young Scientists, grant number 22150610471, and NSFC grant number 22036005) and the Key Laboratory of Biomedical Effects of Nanomaterials and Nanosafety, Chinese Academy of Sciences (NSKF202001) is acknowledged.
References
- D. J. McClements and H. Xiao, Is nano safe in foods? Establishing the factors impacting the gastrointestinal fate and toxicity of organic and inorganic food-grade nanoparticles, npj Sci. Food., 2017, 1, 6 CrossRef PubMed.
- S. van den Brule, J. Ambroise, H. Lecloux, C. Levard, R. Soulas, P. J. De Temmerman, M. Palmai-Pallag, E. Marbaix and D. Lison, Dietary silver nanoparticles can disturb the gut microbiota in mice, Part. Fibre Toxicol., 2016, 13, 38 CrossRef PubMed.
- R. Werlin, J. H. Priester, R. E. Mielke, S. Kramer, S. Jackson, P. K. Stoimenov, G. D. Stucky, G. N. Cherr, E. Orias and P. A. Holden, Biomagnification of cadmium selenide quantum dots in a simple experimental microbial food chain, Nat. Nanotechnol., 2011, 6, 65–71 CrossRef CAS PubMed.
- R. Peters, E. Kramer, A. G. Oomen, Z. E. Herrera Rivera, G. Oegema, P. C. Tomp, R. Fokkink, A. Rietveld, H. J. P. Marvin, S. Weigel, A. A. C. M. Peijnenburg and H. Bouwmeester, Presence of nano-sized silica during in vitro digestion of foods containing silica as a food additive, ACS Nano, 2012, 6, 2441–2451 CrossRef CAS PubMed.
- M. Younes, P. Aggett, F. Aguilar, R. Crebelli, B. Dusemund, M. Filipič, M. J. Frutos, P. Galtier, D. Gott, U. Gundert-Remy, G. G. Kuhnle, J.-C. Leblanc, I. T. Lillegaard, P. Moldeus, A. Mortensen, A. Oskarsson, I. Stankovic, I. Waalkens-Berendsen, R. A. Woutersen, M. Wright, P. Boon, D. Chrysafidis, R. Gürtler, P. Mosesso, D. Parent-Massin, P. Tobback, N. Kovalkovicova, A. M. Rincon, A. Tard and C. Lambré, Re-evaluation of silicon dioxide (E551) as a food additive, EFSA J., 2018, 16, 5088 Search PubMed.
- H. C. Winkler, T. Notter, U. Meyer and H. Naegeli, Critical review of the safety assessment of titanium dioxide additives in food, J. Nanobiotechnol., 2018, 16, 51 CrossRef.
- J. M. Radziwill-Bienkowska, P. Talbot, J. B. J. Kamphuis, V. Robert, C. Cartier, I. Fourquaux, E. Lentzen, J. N. Audinot, F. Jamme, M. Refregiers, J. K. Bardowski, P. Langella, M. Kowalczyk, E. Houdeau, M. Thomas and M. Mercier-Bonin, Toxicity of food-grade TiO2 to commensal intestinal and transient food-borne bacteria: New insights using nano-SIMS and synchrotron UV fluorescence imaging, Front. Microbiol., 2018, 9, 794 CrossRef PubMed.
- EFSA ANS Panel (EFSA Panel on Food Additives and Nutrient Sources added to Food), Scientific Opinion on the re-evaluation of titanium dioxide (E 171) as a food additive, EFSA J., 2016, 14(9), 4545–4583 Search PubMed.
- S. W. P. Wijnhoven, W. J. G. M. Peijnenburg, C. A. Herberts, W. I. Hagens, A. G. Oomen, E. H. W. Heugens, B. Roszek, J. Bisschops, I. Gosens, D. Van De Meent, S. Dekkers, W. H. De Jong, M. van Zijverden, A. J. A. M. Sips and R. E. Geertsma, Nano-silver – a review of available data and knowledge gaps in human and environmental risk assessment, Nanotoxicology, 2009, 3, 109–138 CrossRef CAS.
- M. Younes, G. Aquilina, L. Castle, K. H. Engel, P. Fowler, M. J. Frutos Fernandez, P. Fürst, U. Gundert-Remy, R. Gürtler, T. Husøy, M. Manco, W. Mennes, P. Moldeus, S. Passamonti, R. Shah, I. Waalkens-Berendsen, D. Wölfle, E. Corsini, F. Cubadda, D. De Groot, R. FitzGerald, S. Gunnare, A. C. Gutleb, J. Mast, A. Mortensen, A. Oomen, A. Piersma, V. Plichta, B. Ulbrich, H. Van Loveren, D. Benford, M. Bignami, C. Bolognesi, R. Crebelli, M. Dusinska, F. Marcon, E. Nielsen, J. Schlatter, C. Vleminckx, S. Barmaz, M. Carfí, C. Civitella, A. Giarola, A. M. Rincon, R. Serafimova, C. Smeraldi, J. Tarazona, A. Tard and M. Wright, Safety assessment of titanium dioxide (E171) as a food additive, EFSA J., 2021, 19, 6585 Search PubMed.
- G. Bredeck, A. A. M. Kampfer, A. Sofranko, T. Wahle, D. Lison, J. Ambroise, B. Stahlmecke, C. Albrecht and R. P. F. Schins, Effects of dietary exposure to the engineered nanomaterials CeO2, SiO2, Ag, and TiO2 on the murine gut microbiome, Nanotoxicology, 2021, 15, 934–950 CAS.
- W. Dudefoi, K. Moniz, E. Allen-Vercoe, M. H. Ropers and V. K. Walker, Impact of food grade and nano-TiO2 particles on a human intestinal community, Food Chem. Toxicol., 2017, 106, 242–249 CrossRef CAS.
- M. I. Setyawati, C. Y. Tay and D. T. Leong, Mechanistic investigation of the biological effects of SiO2, TiO2, and ZnO nanoparticles on intestinal cells, Small, 2015, 11, 3458–3468 CrossRef CAS.
- I. Javed, X. J. Cui, X. Y. Wang, M. Mortimer, N. Andrikopoulos, Y. H. Li, T. P. Davis, Y. L. Zhao, P. C. Ke and C. Y. Chen, Implications of the human gut-brain and gut-cancer axes for future nanomedicine, ACS Nano, 2020, 14, 14391–14416 CrossRef PubMed.
- B. Lamas, N. M. Breyner and E. Houdeau, Impacts of foodborne inorganic nanoparticles on the gut microbiota-immune axis: potential consequences for host health, Part. Fibre Toxicol., 2020, 17, 19 CrossRef PubMed.
- Z. Chen, S. Han, D. Zhou, S. Zhou and G. Jia, Effects of oral exposure to titanium dioxide nanoparticles on gut microbiota and gut-associated metabolism in vivo, Nanoscale, 2019, 11, 22398–22412 RSC.
- Y. Zhang, M. Mortimer and L. H. Guo, Interplay between engineered nanomaterials and microbiota, Environ. Sci.: Nano, 2020, 7, 2454–2485 RSC.
- Q. Zhang, T. Xia and C. Zhang, Chronic exposure to titanium dioxide nanoparticles induces commensal-to-pathogen transition in Escherichia coli, Environ. Sci. Technol., 2020, 54, 13186–13196 CrossRef CAS.
- B. J. Teubl, C. Schimpel, G. Leitinger, B. Bauer, E. Frohlich, A. Zimmer and E. Roblegg, Interactions between nano-TiO2 and the oral cavity: impact of nanomaterial surface hydrophilicity/hydrophobicity, J. Hazard. Mater., 2015, 286, 298–305 CrossRef CAS PubMed.
- K. Zupancic, V. Kriksic, I. Kovacevic and D. Kovacevic, Influence of oral probiotic Streptococcus salivarius K12 on ear and oral cavity health in humans: Systematic review, Probiotics Antimicrob. Proteins, 2017, 9, 102–110 CrossRef CAS PubMed.
- P. Hols, L. Ledesma-Garcia, P. Gabant and J. Mignolet, Mobilization of Microbiota Commensals and Their Bacteriocins for Therapeutics, Trends Microbiol., 2019, 27, 690–702 CrossRef CAS PubMed.
- C. Cosseau, D. A. Devine, E. Dullaghan, J. L. Gardy, A. Chikatamarla, S. Gellatly, L. L. Yu, J. Pistolic, R. Falsafi, J. Tagg and R. E. W. Hancock, The commensal Streptococcus salivarius K12 downregulates the innate immune responses of human epithelial cells and promotes host-microbe homeostasis, Infect. Immun., 2008, 76, 4163–4175 CrossRef CAS.
- J. Fallingborg, Intraluminal pH of the human gastrointestinal tract, Dan. Med. Bull., 1999, 46, 183 CAS.
- I. S. Sohal, Y. K. Cho, K. S. O'Fallon, P. Gaines, P. Demokritou and D. Bello, Dissolution behavior and biodurability of ingested engineered nanomaterials in the gastrointestinal environment, ACS Nano, 2018, 12, 8115–8128 CrossRef CAS PubMed.
- H. L. Zhou, J. Pandya, Y. B. Tan, J. N. Liu, S. F. Peng, J. L. M. Mundo, L. L. He, H. Xiao and D. J. McClements, Role of mucin in behavior of food-grade TiO2 nanoparticles under simulated oral conditions, J. Agric. Food Chem., 2019, 67, 5882–5890 CrossRef CAS.
- L. X. Y. Li, A. U. Khan, X. Zhang, X. T. Qian and Y. W. Wang, Emerging investigator series: chemical transformation of silver and zinc oxide nanoparticles in simulated human tear fluids: influence of biocoronae, Environ. Sci.: Nano, 2021, 8, 2430–2440 RSC.
- S. De Vos, N. Waegeneers, E. Verleysen, K. Smeets and J. Mast, Physico-chemical characterisation of the fraction of silver (nano)particles in pristine food additive E174 and in E174-containing confectionery, Food Addit. Contam., Part A, 2020, 37, 1831–1846 CrossRef CAS.
- Y. Yang, J. J. Faust, J. Schoepf, K. Hristovski, D. G. Capco, P. Herckes and P. Westerhoff, Survey of food-grade silica dioxide nanomaterial occurrence, characterization, human gut impacts and fate across its lifecycle, Sci. Total Environ., 2016, 565, 902–912 CrossRef CAS.
- Y. Yang, K. Doudrick, X. Bi, K. Hristovski, P. Herckes, P. Westerhoff and R. Kaegi, Characterization of food-grade titanium dioxide: the presence of nanosized particles, Environ. Sci. Technol., 2014, 48, 6391–6400 CrossRef CAS PubMed.
- C. A. Schneider, W. S. Rasband and K. W. Eliceiri, NIH Image to ImageJ: 25 years of image analysis, Nat. Methods, 2012, 9, 671–675 CrossRef CAS PubMed.
- P. Roger, J. Delettre, M. Bouix and C. Beal, Characterization of Streptococcus salivarius growth and maintenance in artificial saliva, J. Appl. Microbiol., 2011, 111, 631–641 CrossRef CAS.
- M. Mortimer, N. Devarajan, D. Li and P. A. Holden, Multiwall carbon nanotubes induce more pronounced transcriptomic responses in pseudomonas aeruginosa PG201 than graphene, exfoliated boron nitride, or carbon black, ACS Nano, 2018, 12, 2728–2740 CrossRef CAS PubMed.
- M. Mortimer, D. Li, Y. Wang and P. A. Holden, Physical properties of carbon nanomaterials and nanoceria affect pathways important to the nodulation competitiveness of the symbiotic N-2-Fixing bacterium bradyrhizobium diazoefficiens, Small, 2020, 16, e1906055 CrossRef.
- K. L. Lam, S. Lin, C. R. Liu, X. Y. Wu, S. Tang, H. S. Kwan and P. Cheung, Low-cost method generating In situ anaerobic conditions on a 96-well plate for microbial fermentation in food research, J. Agric. Food Chem., 2018, 66, 11839–11845 CrossRef CAS PubMed.
- M. Mortimer, T. Kefela, A. Trinh and P. A. Holden, Uptake and depuration of carbon- and boron nitride-based nanomaterials in the protozoa Tetrahymena thermophila, Environ. Sci.: Nano, 2021, 8, 3613–3628 RSC.
- K. L. Hockett and D. A. Baltrus, Use of the soft-agar overlay technique to screen for bacterially produced inhibitory compounds, J. Visualized Exp., 2017, 14, 55064 Search PubMed.
- A. Bauernfeind and J. R. Burrows, Suggested procedure allowing use of plastic petri dishes in bacteriocin typing, Appl. Environ. Microbiol., 1978, 35, 970 CrossRef CAS PubMed.
- P. Reid, N. C. K. Heng, J. D. Hale, D. Krishnan, J. Crane, J. R. Tagg and T. J. Milne, A TaqMan-based quantitative PCR screening assay for the probiotic Streptococcus salivarius K12 based on the specific detection of its megaplasmid-associated salivaricin B locus, J. Microbiol.
Methods, 2020, 170, 105837 CrossRef CAS.
- H. P. Horz, A. Meinelt, B. Houben and G. Conrads, Distribution and persistence of probiotic Streptococcus salivarius K12 in the human oral cavity as determined by real-time quantitative polymerase chain reaction, Oral Microbiol. Immunol., 2010, 22, 126–130 CrossRef.
- B. Couvigny, N. Lapaque, L. Rigottier-Gois, A. Guillot, S. Chat, T. Meylheuc, S. Kulakauskas, M. Rohde, M. Mistou and P. Renault, Three glycosylated serine-rich repeat proteins play a pivotal role in adhesion and colonization of the pioneer commensal bacterium, Streptococcus salivarius, Environ. Microbiol., 2017, 19, 3579–3594 CrossRef CAS PubMed.
- P. W. Michael, A new mathematical model for relative quantification in real-time RT–PCR, Nucleic Acids Res., 2001, 29, 45 CrossRef.
- T. Waller, C. Chen and S. L. Walker, Food and industrial grade titanium dioxide impacts gut microbiota, Environ. Eng. Sci., 2017, 34, 537–550 CrossRef CAS.
- M. E. Carlotti, E. Ugazio, S. Sapino, I. Fenoglio, G. Greco and B. Fubini, Role of particle coating in controlling skin damage photoinduced by titania nanoparticles, Free Radical Res., 2009, 43, 312–322 CrossRef CAS.
- A. Jaroenworaluck, W. Sunsaneeyametha, N. Kosachan and R. Stevens, Characteristics of silica-coated TiO2 and its UV absorption for sunscreen cosmetic, Surf. Interface Anal., 2010, 38, 473–477 CrossRef.
- Y. Q. Bi, A. K. Marcus, H. Robert, R. Krajmalnik-Brown, B. E. Rittmann, P. Westerhoff, M. H. Ropers and M. Mercier-Bonin, The complex puzzle of dietary silver nanoparticles, mucus and microbiota in the gut, J. Toxicol. Environ. Health, Part B, 2020, 23, 69–89 CAS.
- L. Laloux, D. Kastrati, S. Cambier, A. C. Gutleb and Y. J. Schneider, The food matrix and the gastrointestinal fluids alter the features of silver nanoparticles, Small, 2020, 16, e1907687 CrossRef PubMed.
- Y. Li, K. Jiang, H. Cao, M. Yuan and F. Xu, Influences of a standardized food matrix and gastrointestinal fluids on the physicochemical properties of titanium dioxide nanoparticles, RSC Adv., 2021, 11, 11568–11582 RSC.
- D. Srinivasan, W. H. Phue, K. Xu and S. George, The type of dietary nanoparticles influences salivary protein corona composition, NanoImpact, 2020, 19, 100238 CrossRef.
- W. Wu, R. Zhang, D. J. McClements, B. Chefetz, T. Polubesova and B. Xing, Transformation and speciation analysis of silver nanoparticles of dietary supplement in simulated human gastrointestinal tract, Environ. Sci. Technol., 2018, 52, 8792–8800 CrossRef CAS.
- Y. Sun, T. Zhen, Y. Li, Y. Wang, M. Wang, X. Li and Q. Sun, Interaction of food-grade titanium dioxide nanoparticles with pepsin in simulated gastric fluid, LWT--Food Sci. Technol., 2020, 134, 110208 CrossRef CAS.
- B. Justus, A. F. M. Arana, M. M. Goncalves, K. Wohnrath, P. M. D. Boscardin, C. C. Kanunfre, J. M. Budel, P. V. Farago and J. de Paula, Characterization and cytotoxic evaluation of silver and gold nanoparticles produced with aqueous extract of Lavandula dentata L. in relation to K-562 cell line, Braz. Arch. Biol. Technol., 2019, 62, e19180731 CrossRef CAS.
- A. Mirabedini, S. M. Mirabedini, A. A. Babalou and S. Pazokifard, Synthesis, characterization and enhanced photocatalytic activity of TiO2/SiO2 nanocomposite in an aqueous solution and acrylic-based coatings, Prog. Org. Coat., 2011, 72, 453–460 CrossRef CAS.
- A. Barbour, P. Wescombe and L. Smith, Evolution of Lantibiotic Salivaricins: New weapons to fight infectious diseases, Trends Microbiol., 2020, 28, 578–593 CrossRef CAS PubMed.
- H. D. Murray, D. A. Schneider and R. L. Gourse, Control of rRNA expression by small molecules
is dynamic and nonredundant, Mol. Cell, 2003, 12, 125–134 CrossRef CAS PubMed.
- V. Aruoja, S. Pokhrel, M. Sihtmae, M. Mortimer, L. Madler and A. Kahru, Toxicity of 12 metal-based nanoparticles to algae, bacteria and protozoa, Environ. Sci.: Nano, 2015, 2, 630–644 RSC.
- O. Bondarenko, K. Juganson, A. Ivask, K. Kasemets, M. Mortimer and A. Kahru, Toxicity of Ag, CuO and ZnO nanoparticles to selected environmentally relevant test organisms and mammalian cells in vitro: a critical review, Arch. Toxicol., 2013, 87, 1181–1200 CrossRef CAS.
- W. Huang, F. Tao, F. Li, M. Mortimer and L.-H. Guo, Antibacterial nanomaterials for environmental and consumer product applications, NanoImpact, 2020, 20, 100268 CrossRef.
- Y. Qing, L. Cheng, R. Li, G. Liu, Y. Zhang, X. Tang, J. Wang, H. Liu and Y. Qin, Potential antibacterial mechanism of silver nanoparticles and the optimization of orthopedic implants by advanced modification technologies, Int. J. Nanomed., 2018, 13, 3311–3327 CrossRef CAS.
- Z. M. Xiu, Q. B. Zhang, H. L. Puppala, V. L. Colvin and P. J. J. Alvarez, Negligible particle-specific antibacterial activity of silver nanoparticles, Nano Lett., 2012, 12, 4271–4275 CrossRef CAS.
- D. Barros, A. Pradhan, V. M. Mendes, B. Manadas, P. M. Santos, C. Pascoal and F. Cassio, Proteomics and antioxidant enzymes reveal different mechanisms of toxicity induced by ionic and nanoparticulate silver in bacteria, Environ. Sci.: Nano, 2019, 6, 1207–1218 RSC.
- O. Bondarenko, A. Ivask, A. Kakinen, I. Kurvet and A. Kahru, Particle-cell contact enhances antibacterial activity of silver nanoparticles, PLoS One, 2013, 8, e64060 CrossRef CAS PubMed.
- J. Liu, Z. Wang, F. D. Liu, A. B. Kane and R. H. Hurt, Chemical transformations of nanosilver in biological environments, ACS Nano, 2012, 6, 9887–9899 CrossRef CAS.
- K. Juganson, M. Mortimer, A. Ivask, K. Kasemets and A. Kahru, Extracellular conversion of silver ions into silver nanoparticles by protozoan Tetrahymena thermophila, Environ. Sci.: Processes Impacts, 2013, 15, 244–250 RSC.
- X. Tian, X. Jiang, C. Welch, T. R. Croley, T.-Y. Wong, C. Chen, S. Fan, Y. Chong, R. Li, C. Ge, C. Chen and J.-J. Yin, Bactericidal effects of silver nanoparticles on Lactobacilli and the underlying mechanism, ACS Appl. Mater. Interfaces, 2018, 10, 8443–8450 CrossRef CAS PubMed.
- B. Collin, O. Tsyusko, D. L. Starnes and J. Unrine, Effect of natural organic matter on dissolution and toxicity of sulfidized silver nanoparticles to Caenorhabditis elegans, Environ. Sci.: Nano, 2017, 3, 728–736 RSC.
- M. Sikder, M. N. Croteau, B. A. Poulin and M. Baalousha, Effect of nanoparticle size and natural organic matter composition on the bioavailability of polyvinylpyrrolidone-coated platinum nanoparticles to a model freshwater invertebrate, Environ. Sci. Technol., 2021, 55, 2452–2461 CrossRef CAS PubMed.
- N. von Goetz, C. Lorenz, L. Windler, B. Nowack, M. Heuberger and K. Hungerbuhler, Migration of Ag- and TiO2-(Nano)particles from textiles into artificial sweat under physical stress: experiments and exposure modeling, Environ. Sci. Technol., 2013, 47, 9979–9987 CrossRef CAS PubMed.
- G. Kaci, D. Goudercourt, V. Dennin, B. Pot, J. Dore, S. D. Ehrlich, P. Renault, H. M. Blottiere, C. Daniel and C. Delorme, Anti-inflammatory properties of Streptococcus salivarius, a commensal bacterium of the oral cavity and digestive tract, Appl. Environ. Microbiol., 2014, 80, 928–934 CrossRef PubMed.
- A. Garcia-Rodriguez, F. Moreno-Olivas, R. Marcos, E. Tako, C. N. H. Marques and G. J. Mahler, The role of metal oxide nanoparticles, Escherichia coli, and Lactobacillus rhamnosus on small intestinal enzyme activity, Environ. Sci.: Nano, 2020, 7, 3940–3964 RSC.
- O. Hyink, P. A. Wescombe, M. Upton, N. Ragland, J. P. Burton and J. R. Tagg, Salivaricin A2 and the novel lantibiotic salivaricin B are encoded at adjacent loci on a 190-kilobase transmissible megaplasmid in the oral probiotic strain Streptococcus salivarius K12, Appl. Environ. Microbiol., 2007, 73, 1107–1113 CrossRef CAS PubMed.
- W. S. Kim, J. Y. Lee, B. Singh, S. Maharjan, L. Hong, S. M. Lee, L. H. Cui, K. J. Lee, G. Kim, C. H. Yun, S. K. Kang, Y. J. Choi and C. S. Cho, A new way of producing pediocin in Pediococcus acidilactici through intracellular stimulation by internalized inulin nanoparticles, Sci. Rep., 2018, 8, 5878 CrossRef.
- M. Mortimer, Y. Wang and P. A. Holden, Molecular mechanisms of nanomaterial-bacterial interactions revealed by omics - the role of nanomaterial effect level, Front. Bioeng. Biotechnol., 2021, 9, 683520 CrossRef PubMed.
-
M. Mortimer, W. Fang, X. Zhou, M. Vodovnik and L.-H. Guo, in Advances in Toxicology and Risk Assessment of Nanomaterials and Emerging Contaminants, ed. M. Mortimer and L. H. Guo, Springer Singapore, Singapore, 2022, vol. 34, pp. 61–94 Search PubMed.
|
This journal is © The Royal Society of Chemistry 2023 |