Influence of water exchange rates on toxicity and bioaccumulation of hydrophobic organic chemicals in sediment toxicity tests†
Received
14th November 2022
, Accepted 25th January 2023
First published on 25th January 2023
Abstract
In standardized sediment toxicity tests, the applied water exchange methods range from static to flow-through conditions and vary between protocols and laboratories even for the same test species. This variation potentially results in variable chemical exposure, hampering the interpretation of toxicity and bioaccumulation. To address these issues, we performed sediment toxicity tests with a mixture of three polycyclic aromatic hydrocarbons (PAHs) and the freshwater epibenthic amphipod Hyalella azteca as model chemicals and organism, respectively. Five standardized water exchange methods were applied: static, semi-static, or flow-through conditions. By measuring total (Cdiss) and freely dissolved concentrations (Cfree) of PAHs with water sampling and direct immersion solid-phase microextraction methods, respectively, we found that Cdiss in overlying water differed by a factor of up to 107 among water exchange conditions, whereas both Cdiss and Cfree in pore water did not differ by more than a factor of 2.6. Similar survival rates, growth rates, and bioaccumulation of PAHs between water exchange methods suggest that H. azteca was predominantly exposed to pore water rather than overlying water. By applying mechanistic kinetic modeling to simulate spatiotemporal concentration profiles in sediment toxicity tests, we discuss the importance of the water exchange rates and resulting temporal and spatial exposure variability for the extrapolation of laboratory sediment toxicity to field conditions, particularly for chemicals with relatively low hydrophobicity and sediments with low organic carbon content.
Environmental significance
Sediment toxicity tests are required by risk regulations for hydrophobic organic chemicals (HOCs), but there are still unknowns regarding sediment toxicity tests, such as the distribution of a test chemical and its relationship to bioaccumulation and toxicity. In this study, to clarify some of these unknowns, we examined the distribution of polycyclic aromatic hydrocarbons, as model HOCs, in sediment toxicity tests and the bioaccumulation in H. azteca under various water exchange conditions. The findings in this study would be useful in interpreting the results of sediment toxicity tests and in extrapolation from laboratory to field.
|
1. Introduction
Sediment toxicity tests are widely used tools to assess the toxicity of contaminated sediment and sediment-associated chemicals. In sediment toxicity tests, benthic organisms are exposed to field-collected contaminated sediment or to clean sediment spiked with a chemical of interest. The chemical distribution in the sediment toxicity tests can be affected by various mechanisms: for example, sorption/desorption to/from sediment particles,1–4 molecular diffusion and colloid- and dissolved organic carbon (DOC)-mediated transport in sediment pores,1,3 advection in overlying water, and resuspension of particles.5 These interplaying and competing kinetic processes can lead to variable and uncertain exposure in time and space,6,7 ultimately hampering the interpretation of observed toxicity and bioaccumulation and their translation into sediment quality guidelines.
Exchange of overlying water is commonly performed in sediment toxicity tests to supply oxygen and remove toxic excretes (e.g., ammonia); however, exchange methods vary depending on protocols and laboratories even for the same test species. Common methods include no exchange (i.e., static),8,9 exchange with clean water at a certain time interval (i.e., semi-static), and continuous or intermittent flow-through exchange10,11 (Table 1). Recently, we observed decreasing concentrations of hydrophobic organic chemicals (HOCs) in overlying water with time under semi-flow-through conditions.6,7 Others reported that, as a result of decreasing concentrations in overlying water, flow-through exposure decreased the observed toxicity of field-collected and cadmium-spiked sediments to an estuarine amphipod Melita plumulosa and a freshwater amphipod Hyalella azteca, respectively, as compared to semi-static and static exposure.12,13 These observations indicate the need to evaluate the effects of water exchange on the chemical distribution and the test results in a systematic, quantitative, and mechanistic manner; however, previous studies did not attempt to provide mechanistic insights and were limited to metals and ammonia.8,14
Table 1 Water exchange conditions tested in this study
|
System |
Exchange frequency |
Exchange volume |
Protocol |
Examples of studies that applied the exchange method for H. azteca |
In the morning and evening, one volume of overlying water was exchanged with clean dechlorinated tap water.
Static condition is recommended, but the semi-static condition is described as an option in case water quality deteriorates.
Two-thirds of the overlying water was exchanged three times for 10 days.
One-third of the overlying water was exchanged twice daily.
Half of the overlying water was exchanged three times daily.
|
Condition 1 |
Static |
No exchange, with aeration |
— |
Environment and Climate Change Canada, 2017b 28 |
p,p′-DDT, endrin,31 and cypermethrin32 |
Condition 2 |
Semi-static |
Three times per week |
2 beaker volumes per daya |
Environment and Climate Change Canada, 2017b 28 |
Antifouling paint particlesc 33 |
Condition 3 |
Semi-static |
Everyday |
2 beaker volumes per daya |
ASTM, 2020;30 USEPA, 2016 10 |
p,p′-DDTd (ref. 34) |
Condition 4 |
Flow-through |
Continuously exchanged |
1 beaker volume per day |
— |
Permethrin and p,p′-DDTe 35 |
Condition 5 |
Flow-through |
Continuously exchanged |
2 beaker volumes per day |
ASTM, 2020;30 USEPA, 2016 10 |
Pyrethroids, chlorpyrifos, and p,p′-DDT36 |
The impact of water exchange is expected to be more pronounced for epibenthic organisms residing on the sediment surface (e.g., amphipods and ostracods)15–17 than endobenthic organisms burrowing in the sediment (e.g., oligochaetes and midge larvae),14 as the water exchange dilutes the chemical concentrations in the overlying water, whereas HOCs are expected to be retained in the sediment.6,7 In fact, epibenthic species were used in the above two studies demonstrating the decrease in the observed toxicity by flow-through exposure.12,13 However, epibenthic organisms occasionally burrow in sediment, and other studies have indicated the importance of pore water rather than overlying water for the toxicity of HOCs to the epibenthic freshwater amphipod H. azteca.6,18 Also, dilution of overlying water triggers the desorption of chemicals for the top layer of sediment and can substantially decrease the local concentration at the sediment–water interface. Thus, the influence of water exchange on toxicity is determined by a complicated interplay between organism's behavior, chemical's sorption properties, and sediment characteristics and should thus be investigated mechanistically.
In addition to the temporal and spatial variability in water concentrations, test chemicals are present in the freely dissolved and particle- and DOC-bound states in both overlying and pore water, depending on their hydrophobicity.6,7 The freely dissolved concentration (Cfree) of organic contaminants is generally considered a suitable metric for the interpretation of toxicity to and bioaccumulation in benthic organisms.19–21 However, the contribution of the dietary route to the overall chemical uptake has been reported to be significant for HOCs that strongly sorb to organic carbon.22,23 The uptake of organic carbon-bound chemicals can increase the observed toxicity to aquatic organisms beyond what would be expected from the freely dissolved fraction alone.24–26 Therefore, to establish a robust link between exposure, bioaccumulation, and toxicity, various types of concentrations such as Cfree and total dissolved concentration (Cdiss = Cfree + concentration bound to DOC) should be monitored in time and space.6
In this study, we performed sediment toxicity tests with a mixture of three polycyclic aromatic hydrocarbons (PAHs; phenanthrene [Phe], pyrene [Pyr], and benzo[a]pyrene [BaP]), covering a wide range of hydrophobicity, and the freshwater epibenthic amphipod H. azteca as a model epibenthic organism under five different water exchange conditions, including static, semi-static, and flow-through conditions. We measured Cdiss (over time) and Cfree (at the end of exposure) in overlying and pore water. Experimental concentrations of PAHs were compared to numerical modeling that simulates spatiotemporal concentration profiles under given exchange conditions. To analyze the relevance of exposure concentration profiles to observed toxicity and bioaccumulation, we measured amphipod lethality, growth, and internal PAH concentrations (Corg) under the respective water exchange conditions. Finally, to assess the exposure gap between laboratory sediment toxicity tests and the sediment–water environment, we simulated spatiotemporal concentration profiles under high water flow conditions common for field streams and compared the results to our experimental data and model simulations in sediment tests.
2. Materials and methods
2.1. Materials and chemicals
BaP (>97.0% purity; CAS: 50-32-8), Phe (>99.0% purity, CAS: 85-01-8), Pyr (>98.0% purity; CAS:129-00-0), acetone (>99.5% purity), acetonitrile (>99.8% purity), and kaolin (practical grade) were purchased from Fujifilm Wako Pure Chemicals Co. Ltd. Quartz sand (0.2–0.8 mm particle: ≥40%, loss on ignition at 900 °C: ≤0.05%) was purchased from Merck & Co. Peat moss originating from Hokkaido, Japan was purchased from Midori Co. The elemental composition of the peat moss was N 1.5% w/w, C 37.6% w/w, and H 4.4% w/w, as determined using an elemental analyzer (Flash EA1112, Thermo Fisher Scientific).
2.2. Test organisms
H. azteca is an epibenthic detrivore that does not burrow into sediment deeply and ingests algae, bacteria, epiphytes, and detritus.27 It was obtained from a brood stock which has been maintained for more than 10 years at the National Institute for Environmental Studies, Japan. The details of culture conditions were reported elsewhere.6 Briefly, the stock culture was maintained in aquaria containing dechlorinated tap water and nylon mesh sheets (mesh size: 500 μm) at 22 °C under a photoperiod of 16 hours of light and 8 hours of darkness, supplied with continuous aeration, and fed with synthesized food Halios (Feed One Co.) twice a week. To evaluate the sensitivity of test organisms, a 96-h water-only test was performed with cadmium chloride as a reference toxicant according to Environment and Climate Change Canada (2017).28 The median lethal concentration (LC50) was 4.1 μg Cd per L (95% confidence interval: 2.7–5.5, based on nominal concentrations), which fell within two standard deviations (SD) of the laboratory's historical LC50 of 4.2 ± 1.4 μg Cd per L (geometric mean ± SD), thus indicating acceptable sensitivity of the population used in this study.
2.3. Exposure test with different water exchange schemes
The tests were performed according to the standardized protocols28,29 with slight modifications. Formulated sediment containing quartz sand (75% dry w/w), peat moss (5% dry w/w), and kaolinite (20% dry w/w) was prepared according to the OECD test guideline 218 (ref. 9) and our previous study.6 Each PAH (i.e., Pyr, Phe, and BaP) was mixed with quartz sand to achieve the nominal concentration of 30 mg kg−1-dry. Dechlorinated tap water was added to the spiked sediment to give a water content of 30% wet w/w. The spiked sediment in a brown glass bottle was shaken with both rolling and reciprocating movements on a roller shaker (BR-12, Thermal Chemical Industry Co. Ltd, Tokyo, Japan) at 6–8 °C at about 10 rpm for 10 days.
Ninety wet grams of the prepared sediment (equivalent to 55 mL), two pieces of 4-cm polydimethylsiloxane (PDMS)-coated glass fibers (see below for the details), and 220 mL of dechlorinated tap water were transferred to a 300 mL glass tall beaker. Five replicate beakers were prepared under each water exchange condition, of which three beakers were used for the toxicity tests and two were prepared to collect pore water by centrifugation and were sacrificed 11 days after the beaker preparation, which corresponds to Day 10 of the sediment toxicity tests. Four additional beakers, common to all conditions, were prepared to collect pore water on Day 0.
In all the water exchange conditions, the overlying water was exchanged with four beaker volumes of dechlorinated tap water within 24 hours after sediment transfer using the flow-through water exchange system to remove easily suspended particles. After this 24-h pre-equilibration (i.e., on Day 0), 10 juvenile amphipods (6–8 days old) were added to the beakers. Overlying water was exchanged over 10 days using four exchange conditions, which were adopted from the standardized protocols,10,28,30 with different exchange frequencies and rates (Table 1). Under Condition 1, overlying water was not exchanged (i.e., static), but continuously aerated with an air pump and a Pasteur pipette placed about 1 cm below the water surface. Under Conditions 2 and 3, water exchange was carried out by dropping 220 mL water into the beaker within 2 min (ESI, Video†) in the morning and evening, resulting in a total of 440 mL per day. This renewal was repeated three times per week and daily under Conditions 2 and 3, respectively. Under Conditions 4 and 5, overlying water was continuously exchanged at rates of 220 mL day−1 and 440 mL day−1 (i.e., one and two beaker volumes per day), respectively, using the water exchange system with needles (27 G for Condition 4 and 25 G for Condition 5; Nipro Corp., Osaka, Japan). The water delivery rate under Conditions 4 and 5 was monitored every other day for all the beakers and confirmed to be within ±30% of the nominal values. The overlying water was not aerated under Conditions 2–5.
Amphipods were fed with 1.75 mL of yeast–cerophyll–trout chow (YCT, Recenttec K.K., Tokyo, Japan) and 3.15 mg of grounded Halios three times per week.28 Exposure beakers were kept at 23 °C under a photoperiod of 16 hours of light and 8 hours of darkness. Over the test duration, water quality parameters in overlying water (i.e., dissolved oxygen, pH, conductivity, temperature, and ammonia) were checked to be within the acceptable ranges for the survival and growth of H. azteca (Table S1†). At the end of exposure (i.e., Day 10), the pH and ammonia concentration in pore water were also measured (Table S2†). On Day 10, surviving amphipods were retrieved using a 250 μm nylon mesh and counted. Missing amphipods were considered dead. Surviving amphipods were transferred to a beaker containing dechlorinated tap water for 1 h to remove gut contents, fixed with liquid nitrogen, and stored at −20 °C until growth and bioaccumulation measurements.
2.4. Concentration measurement
C
free measurement using PDMS fiber.
C
free in the pore and overlying water (i.e., Cfree,pore and Cfree,over) was measured using a passive sampling method with PDMS-coated glass fibers. In this method, the concentrations in the PDMS coating phase (CPDMS) were measured and converted to the corresponding freely dissolved concentrations via the equation, Cfree,pore (or Cfree,over) = CPDMS/KPDMS/w, where KPDMS/w is the PDMS/water partition coefficient. PDMS fibers (10 μm coating thickness, Polymicro Technologies) were cut into lengths of 4 and 7 cm (PDMS volume of 0.53 and 0.92 μL, respectively). The fibers were rinsed twice for 15 min with ethyl acetate and methanol, dried under the fume hood, and stored in Milli-Q water. Log
KPDMS/w for Phe, Pyr, and BaP were measured to be 3.65 ± 0.02, 4.29 ± 0.01, and 5.13 ± 0.01 at 25 °C by the method described in the ESI.† These values were close to the log
KPDMS/w reported in a previous study for PDMS fibers (3.73, 4.28, and 5.22, respectively, at 22.2 °C).37
On Day −1, two pieces per beaker of 4 cm PDMS fibers for Cfree,pore measurement were buried into the sediment. On Day 0, one piece of 7 cm PDMS fiber for Cfree,over measurement was placed in the overlying water, standing on the sediment and leaning against the wall diagonally to the sediment. Since this 7 cm fiber for Cfree,over was partially exposed to the sediment–water interface where Cfree was higher than that in the bulk overlying water, the measured Cfree,over would be somewhat higher than the actual bulk Cfree,over.38 On Day 10, the PDMS fibers were retrieved from the overlying water and sediment, wiped with moist lint-free tissues (Kimwipes, Kimberly-Clark), transferred to a 1.5 mL vial and extracted with 1 mL of acetonitrile. In our previous study, we confirmed that 10 days are enough to achieve equilibrium for the three PAHs between PDMS fibers and water.6 The concentrations of Phe, Pyr, and BaP in the extracts were measured with a high-performance liquid chromatography (HPLC) system, as described in the ESI.†
C
diss measurement.
C
diss was defined as the concentration in water samples filtered with a glass fiber filter (GB-140, pore size: 0.4 μm, Advantec). Over the 10-day sediment toxicity tests, up to 6 mL of overlying water were repeatedly taken from 1 to 2 cm above the sediment surface with a 10 mL glass syringe (Tsubasa Industry) and was filtered with the glass fiber filter. The exact timing of water sampling was shown in the Excel sheet in the ESI.† Under Conditions 2 and 3, overlying water was collected from just below the water surface in addition. On Days 0 and 10, pore water was collected by centrifugation of wet sediment at 1900×g for 15 min (S700T, Kubota) and subsequent 10
000×g for 30 min (CR21, Hitachi), followed by filtration with the glass fiber filter. The filtered overlying and pore water samples were diluted with the same volume of acetonitrile for the concentration determination by HPLC. The same filtered water samples were used for the determination of DOC (Days 0, 1, 2, 7, and 10) and ammonia concentrations (Days 0 and 10).
Growth and Corg measurements.
For the measurement of PAH concentrations in surviving amphipods (Corg), fixed amphipods were dried in a desiccator at room temperature for 24 h. The dry weights of the amphipods were measured, and they were homogenized in 1 mL of acetonitrile with glass beads and a homogenizer (μT-12, Taitec) at 3200 rpm for 3 min. The homogenates were filtered with a PTFE membrane (pore size: 0.45 μm) and analyzed with HPLC. At the start of exposure (Day 0), 40 additional juvenile amphipods (6–8 days old) were collected from the same population as used for the exposure tests, and their dry weight was determined as 0.02 mg per amphipod.
C
sed measurement.
For the measurement of dry-weight-based PAH concentrations in spiked sediment (Csed), up to 10 g-wet of sediment samples were collected on Day −1 and Day 10. The sediment was freeze dried using a freeze dryer (FDU-1200, Eyela), and 0.2 g of the dried sediment was extracted twice with 5 mL of a 1
:
1 mixture of acetone and n-hexane in an ultrasonic bath for 15 min. The extracts were separated from sediment by centrifugation at 1900×g for 15 min, diluted with acetonitrile by a factor of 1000, and analyzed with HPLC. The recovery ratios, defined as the ratio of measured Csed on Day −1 to the nominal value, were 57 ± 4%, 70 ± 6%, and 84 ± 7% for Phe, Pyr, and BaP, respectively. Although a surrogate standard was not used for quantification, we confirmed that the recovery ratios for three PAHs were comparable with that of our previous study using deuterated PAHs as surrogate standards.39 The coefficients of variation (CV) of measured Csed on Day −1 were 6.4% (Phe), 8.8% (Pyr), and 8.3% (BaP) (n = 3), indicating that the spiked sediment was homogeneous.
2.5. Kinetic model simulation
The mechanistic chemical transport model was adapted from our previous study7 and modified to calculate the transport and distribution of HOCs in the spiked-sediment toxicity test with different water exchange conditions. Briefly, the model simulates the desorption of chemicals from spiked sediment particles into pore water, diffusion through pores and through the sediment–water interface, and sorption/desorption of chemicals from DOC. The model divides the test system into three zones: (i) the sediment including sediment particles and pore water, (ii) an unstirred water layer (UWL) of 1 mm thickness at the sediment–water interface, and (iii) the overlying water in which water renewal or aeration applies. The overlying water phase is assumed to be well mixed, which was verified in our previous study with a flow-through system equivalent to Condition 5 (ref. 6) and in semi-static conditions (i.e., Conditions 2 and 3) in this study (Fig. S1†). Since this assumption was not verified under static Condition 1, additional model simulation was performed by changing the thickness of UWL (Fig. S2†). The model parameters Csed, the sediment–water partition coefficient (Kd; Lw/kgsed), the sediment organic carbon–water partition coefficient (KOC; Lw/kgOC), and the DOC–water partition coefficient (KDOC/W; Lw/kgDOC) were measured or estimated from experimental data in this study and implemented into the model (Table S8†). The estimated log
KOC values were 4.2 ± 0.1 (Phe), 5.1 ± 0.1 (Pyr), 6.6 ± 0.1 (BaP), which were comparable with the literature values (4.5 for Phe, 4.8 for Pyr, and 6.0 for BaP).40 The nominal values of water exchange rates were used as model parameters. Under Conditions 2 and 3, overlying water was assumed to be replaced completely at the timing of water exchange (i.e., overlying water concentrations became zero) based on the model analysis with different clearance ratios of overlying water (Fig. S3 and Tables S9–S11†). In addition, to establish a link between laboratory and field exposures, the model was run under a flow-through condition with various water exchange rates ranging from 1 to 2000 volumes per day.
2.6. Data analysis
The bioconcentration factor (BCF) was calculated based on measured Corg (mg per kg-dry) and aqueous concentrations (Caq = Cdiss or Cfree, mg L−1) according to the following equation:
where 0.25 is the ratio of the dry to wet weight of H. azteca.41
The root-mean-square error (RMSE) was calculated using experimental and simulated values and was divided by the mean of experimental values to derive the normalized RMSE
.
3. Results and discussion
3.1. Experimental concentrations in overlying and pore water
Among the measured exposure metrics, concentrations in overlying water were most dependent on the applied water exchange conditions (Fig. 1). Cdiss,over of PAHs increased gradually over time under Condition 1 (i.e., static), whereas those in semi-static exposure systems (i.e., Conditions 2 and 3) increased with time but decreased at the time of exchange, and those in flow-through exposure systems (i.e., Conditions 4 and 5) increased during the initial 1–2 days and then decreased or remained stable. Cdiss,over under Conditions 2–5 were lower than under Condition 1 over the entire test period. The largest difference in Cdiss,over on Day 10 was observed between Conditions 1 and 5, with 5.8 times (Phe), 17 times (Pyr), and >107 times (BaP). Note that no clear time trend was observed for BaP likely due to the difficulty in accurate measurement of Cdiss for highly hydrophobic compounds, except under Condition 1. The DOC concentration in overlying water ranged from 2.6 to 50 mg C L−1 and showed similar time trends to Cdiss,over of Phe and Pyr (Fig. S4†): increasing under Condition 1 and decreasing in semi-static and flow-through systems. The largest difference in DOC on Day 10 was observed between Conditions 1 and 5 with 20 times.
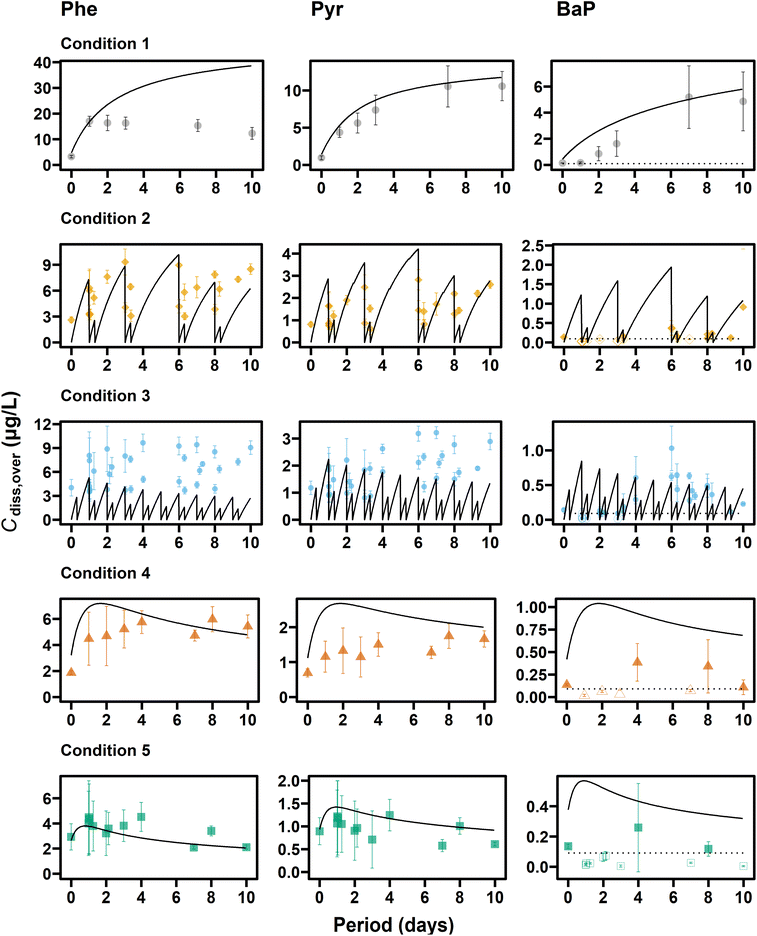 |
| Fig. 1 Time-course changes of total dissolved concentrations of phenanthrene (Phe), pyrene (Pyr), and benzo[a]pyrene (BaP) in overlying water under five different water exchange conditions. Closed and open symbols represent experimental values larger and smaller than the limit of quantification (LOQ), respectively. Dotted lines indicate the LOQ of BaP. Solid lines represent simulated values by the mechanistic model. Error bars represent standard deviations (n = 3 beakers). | |
In contrast to the large differences in Cdiss,over among water exchange conditions, the differences in measured Cdiss,pore were a factor of 1.2, 1.6, and 2.6 at most for Phe, Pyr, and BaP, respectively (Fig. 2). In addition, Cdiss,pore did not change over 10 days under any tested condition (Table S5†). Small influence by water exchange and high stability was also observed for DOC in pore water, with a difference of up to 1.3 times among conditions (Table S3†). These results were consistent with the fact that more than 47% of introduced PAHs and organic carbon remained in sediment phases even in conditions with frequent exchange of overlying water (Table S4†). Note that even when about a half of the added PAH was lost from the sediment phase, the loss predominantly occurred in the upper layer of sediment,4,7 resulting in a stable bulk pore water concentration.
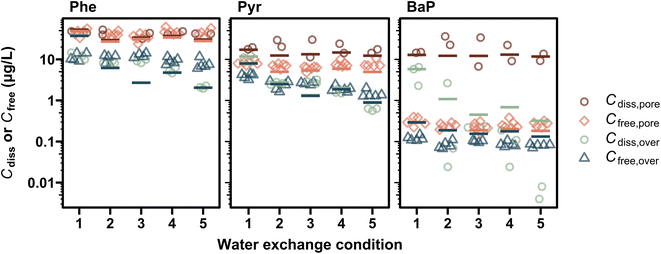 |
| Fig. 2 Experimental concentrations (open symbols) of PAHs under five different water exchange conditions and concentrations simulated by the model (bars). Both experimental and simulated values represent the concentrations at the end of exposure (i.e., Day 10). Different symbols represent different concentration types. Note that simulated total dissolved concentrations of phenanthrene (Phe) in overlying water (Cdiss,over) overlap with simulated freely dissolved concentrations in overlying water (Cfree,over). | |
Differences in Cfree among conditions were absent or small both in overlying and pore water, within a factor of 2.7 and 1.4, respectively (Fig. 2). No difference in Cfree,pore was reasonable, because Csed did not change as described above and because Cfree,pore is controlled by equilibrium partitioning between sediment OC and pore water. Small observed difference in Cfree,over may appear to be inconsistent with the large difference in Cdiss,over between the conditions, but can be explained as follows: for a highly hydrophobic chemical such as BaP (log
KOC: 6.6), Cfree is controlled by desorption equilibrium from DOC-bound fraction. Cdiss,over is influenced by the DOC concentration, which varied, depending on the water exchange conditions, by a factor of up to 20 (Fig. S4†). In contrast, Cfree,over is determined by KDOC/w and the DOC-bound chemical concentration on the DOC-weight basis (i.e., [Cdiss − Cfree]/DOC concentration), which are largely independent of the conditions. For a relatively hydrophilic chemical such as Phe (log
KOC: 4.2), the DOC-bound fraction is negligible at low DOC concentration in overlying water (i.e., <7 mg C L−1, Table S3†) and then Cfree,over should be virtually the same as Cdiss,over, as the model showed (e.g., within a difference of 5% of Cdiss,over for Phe) (Fig. 2 and S5†). The measured Cfree,over of Phe and Pyr, however, was somewhat higher than both time-averaged Cdiss,over and Cdiss,over measured on Day 10 in some cases. The measured Cfree,over might include freely dissolved chemicals in the UWL and pore water phases and might not represent the actual Cfree,over in the bulk overlying water, since the 7 cm PDMS fibers were placed on the sediment surface. Because Cfree in pore water did not differ much among conditions and was higher than Cfree,over, the measured Cfree,over may not reflect the difference in actual bulk Cfree,over. To measure the actual bulk Cfree,over more accurately, it is recommended to place the fibers away from the sediment surface, as suggested in our recent study.38
3.2. Mechanistic analysis using spatiotemporal modeling
Experimental concentration profiles were compared with the concentrations simulated by the model (Fig. 2). The good agreement of Cdiss,pore and Cfree,pore (within a factor of 2 between simulated and experimental values) was not surprising, because Kd and KDOC/w values used in the model were derived from the experimental data in this study. The simulated Cfree,over differed from experimental ones by up to 5 times, but this difference might partially be explained by the inaccurate Cfree,over measurement method as discussed above.
Temporal profiles of Cdiss,over were compared between experimental and simulated values (Fig. 1). In our previous study, temporal trends of Cdiss,over under flow-through conditions (equivalent to Condition 5) were reproduced well by the model.7 In the current study, experimental Cdiss,over increased from Day 0 to 2 due to the change in the water exchange rate from 880 to 220 mL day−1 (Condition 4) and to 440 mL day−1 (Condition 5) on Day 0 and decreased after Day 2. The decreasing trend of Cdiss,over was not clear under Condition 4 compared, which was due to the smaller volume of water exchange under Condition 4. These trends were again reproduced by the model, indicating the applicability of the model to flow-through sediment toxicity tests with different water exchange rates.
Under the static condition (Condition 1), experimental Cdiss,over of Pyr matched well with the simulated values. In contrast, although Cdiss,over of Phe was predicted by the model well within a factor of 4, the experimental data showed a decreasing trend from Day 3, which was not reproduced by the model. The reduction in the Phe concentration could be caused by processes that were not incorporated by the model, such as volatilization and degradation.42 The simulated Cdiss,over was reduced and became closer to the experimental Cdiss,over by increasing the thickness of UWL from 1 mm (NRMSE: 88% for Phe, 31% for Pyr, and 281% for BaP) to 2 mm (NRMSE: 76% for Phe, 20% for Pyr, and 193% for BaP) (Fig. S2†), indicating that the UWL thickness might be larger under the static condition with aeration than the flow-through conditions.
Experimental Cdiss,over values under semi-static Condition 2 were predicted relatively well by the model (NRMSE: 42% for Phe and 50% for Pyr, Tables S9 and S10†), whereas those under Condition 3 with daily water exchange were predicted less well (NRMSE: 70% for Phe and 54% for Pyr). Cdiss,over under Condition 3 decreased at each water exchange, but the concentration just before exchange increased gradually over periods, thereby resulting in larger Cdiss,over under Condition 3 than under Condition 2. This increasing trend was not reproduced by the model and is possibly due to the disturbance of sediment surface by frequent water exchange. Considering the excellent agreement of experimental and simulated DOC in overlying water (Fig. S4†), the poor model reproducibility of Cdiss,over under Condition 3 might be attributed to the presence of re-suspended colloidal particles that were passed through a fiber (0.4 μm pore size), not to DOC.
Through the experimental and simulated results, we can conclude that water exchange rates do not affect pore water concentrations because spiked HOCs are retained in the sediment due to slow diffusion. The overlying water concentration varies depending on the water exchange conditions, and their prediction at different conditions may need to consider the resuspension as well as diffusive transfer.
3.3. Stability of overlying water concentration
Since some epibenthic species such as Corophium volutator have been reported to be more influenced by overlying water than pore water, in particular when exposed to contaminated sediment,15,16 the observed toxicity of spiked-sediment to such species may be affected by water exchange rates. For such species, overlying water concentrations should preferably be stable during exposure for a better interpretation of the test results. The coefficient of variation (CV) of Cdiss,over over 10 days was calculated using the experimental and simulated values under five conditions in this study (Table 2) and was the lowest under flow-through conditions for all three chemicals. The static condition showed the second lowest CV or was comparable with the semi-static conditions because the static condition requires a longer time to reach a steady state. Additional model analyses indicated that the stability of Cdiss,over would increase under static and flow-through conditions, if exposure tests were initiated after a stabilization period of several days (e.g., CV: 6–12% under Condition 1 with 5 days of stabilization, Fig. S6†), as recommended in the chironomid standardized sediment test.9 These results recommend the static condition after stabilization periods and flow-through condition as the stable exposure environments to epibenthic organisms.
Table 2 Variation of total dissolved concentrations of phenanthrene (Phe), pyrene (Pyr), and benzo[a]pyrene (BaP) in overlying water in five different water exchange conditions over 10 days
|
System |
Coefficient of variationa (%) |
Phe |
Pyr |
BaP |
The coefficient of variation was calculated by dividing the standard deviation by the mean of overlying water concentrations over 10 days. Values in parentheses were calculated using the simulated concentrations. NA: not available due to measured concentrations below the LOQ.
|
Condition 1 |
Static |
39 (28) |
57 (27) |
108 (36) |
Condition 2 |
Semi-static |
39 (63) |
44 (62) |
NA (70) |
Condition 3 |
Semi-static |
34 (71) |
39 (69) |
NA (72) |
Condition 4 |
Flow-through |
27 (14) |
26 (11) |
NA (15) |
Condition 5 |
Flow-through |
23 (20) |
24 (14) |
NA (18) |
3.4. Amphipod survival and bioaccumulation
The averaged survival rates of amphipods ranged from 70% to 100% (Fig. 3 and Table S6†) and were not different among water exchange conditions (p > 0.05, one-way ANOVA). Neither the dry weight of a surviving amphipod nor Corg differed statistically significantly among conditions (p > 0.05, one-way ANOVA, Fig. 3 and Tables S6, S7†), with a maximum difference of a factor of 1.5 (dry weight) and 2.1 (Corg). The survival rates observed at Csed tested (i.e., nominally 1.5 × 103 mg per kg-OC for each PAH) were reasonable even considering the additive toxicity of PAHs, because the LC50 values of Phe, Pyr, and BaP for H. azteca were reported to be 1.8 × 104 mg per kg-OC,43 1.1 × 104 mg per kg-OC (our laboratory result of the 14-day test, not published), and 1.0 × 104 mg per kg-OC,6 respectively, by studies using formulated sediment containing peat moss.
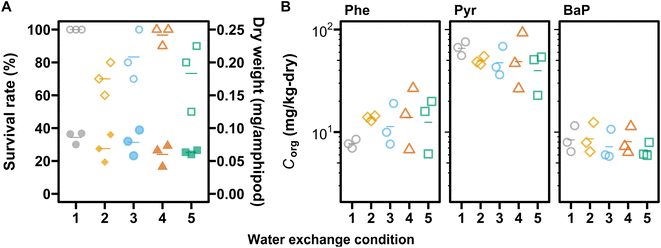 |
| Fig. 3 (A) Survival rate, dry weight, and (B) internal body concentrations (Corg) of three PAHs (phenanthrene [Phe], pyrene [Pyr], and benzo[a]pyrene [BaP]) in five different water exchange conditions. Bars represent arithmetic mean values. (A) Open and closed symbols represent survival rates and dry weight of a surviving amphipod, respectively. | |
The absence of significant differences in survival, growth, and bioaccumulation among water exchange conditions indicates that the variation in Cdiss,over (and possibly Cfree,over) resulting from overlying water exchange might not affect the toxicity and bioaccumulation of the tested chemicals to H. azteca. Together with the visual observation that amphipod did not show obvious avoidance behavior under any conditions, this result suggests that H. azteca was exposed predominantly to pore water as compared to overlying water. To confirm which concentration metric best represented H. azteca bioaccumulation of BaP, we compared BCF values in this study with Cfree-based BCF in water-only tests44 (Fig. 4). The comparison showed that the BCF value in water-only tests (i.e., 3.5 ± 0.1 log units) was lower than BCF values based on Cfree,over (4.3 ± 0.1) and close to those based on Cfree,pore (3.9 ± 0.1). This indicates that the pore water concentration is a more representative indicator for the bioaccumulation of BaP to the epibenthic amphipod H. azteca than the overlying water concentration. These findings are consistent with our previous study on the semi flow-through spiked-sediment toxicity tests of BaP, fluoranthene, and chlorpyrifos with H. azteca.6,38 Note that these results do not negate the role of sediment and DOC ingestion in the uptake of BaP by the amphipod, as Cfree,pore-based BCF did not exactly match that in water-only tests. As shown recently, DOC ingestion can lead to higher bioaccumulation of BaP in H. azteca.45
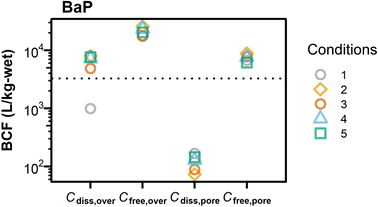 |
| Fig. 4 Comparison of bioconcentration factors (BCF) for BaP measured in spiked-sediment (data points; this study) and water-only tests (dotted line; Schlechtriem et al. 2019).44 Different symbols represent different water exchange conditions. BCF in spiked-sediment tests was calculated based on time-averaged total dissolved concentrations (Cdiss) and based on freely dissolved concentrations (Cfree). BCF values were not normalized to the lipid content. | |
3.5. Model simulation for flow-through conditions at high exchange rates
In contrast to this study, previous studies demonstrated that flow-through conditions reduced the observed toxicity to epibenthic amphipods compared with a static condition.12,13 This apparent inconsistency would be explained by (i) high water exchange rates of 580–720 volumes per day (ref. 12) and of 144 volumes per day (ref. 13) in their studies, which are >70 times higher than those in the present study and the standardized protocols, and (ii) the use of metals and field-collected sediments in their studies. To account for this apparent inconsistency, we performed additional model analysis with flow-through conditions and 2% of fOC. Our simulation indicated that higher exchange rates, at least 2000 volumes per day, could wash out more than 99% of an added hydrophilic chemical (i.e., log
KOC < 2.5) from the beaker during 10 days test (Fig. S8†), thereby considerably reducing uptake and toxicity of the chemical. In contrast, hydrophobic chemicals (i.e., log
KOC > 4) were retained in the beaker, even at higher water exchange rates (i.e., >2000 volumes per day). The model also showed that, for chemicals with log
KOC > 2.5, average pore water concentrations in whole sediment (i.e., depth-averaged concentration) were almost unchanged even at higher water exchange rates, whereas overlying water concentrations were inversely proportional to water exchange rates (Fig. 5). UWL concentrations decreased with increasing exchange rates, in particular for hydrophilic chemicals, but they changed little at exchange rates above 10 volumes per day. These results indicate that higher water exchange rates could reduce the sediment toxicity to benthic organisms by decreasing chemical concentrations at the sediment–water interface, even though depth-averaged pore water concentrations change little.
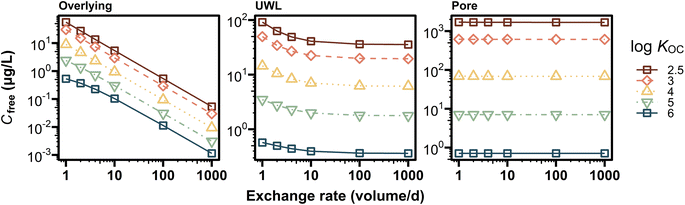 |
| Fig. 5 Relationship between the water exchange rate and simulated freely dissolved concentration (Cfree) in overlying water, the unstirred water layer (UWL), and pore water at the end of 10-day sediment toxicity tests. The simulated concentration was depth-averaged in each phase. Different symbols represent different log KOC values. KDOC/W was set to 0.2 KOC for the model simulation. Initial Csed and UWL thickness were set to 15 mg kg−1 and 1 mm in all simulations, respectively. | |
The additional model analysis highlights the caution in assessing the toxicity of field-collected sediments by laboratory sediment toxicity tests, which are often performed at limited water exchange rates.18,46 Given the limited volume of overlying water in laboratory sediment toxicity tests (this study: 220 mL of volume), the exchange rate of 2000 volumes per day is not high for natural rivers under dry weather conditions. For example, let us consider the case where sediment contamination occurs within a short term (e.g., oil spill, pesticide application) and overlying water becomes clean quickly after the event. In this case, toxicity of less hydrophobic chemicals (i.e., log
Kd < 3) may not be observed in the field because of concentration depletion in the upper layer of sediment, even if laboratory tests with limited water exchange indicate significant toxicity. In contrast, hydrophobic chemicals and organic rich sediment would provide consistent results between laboratory and field studies because of less influence of water flow rates on the chemical exposure.
The model simulations in this study help our quantitative and mechanistic understanding of the influence of water flow rate on chemical persistence in sediment environments. However, the observed loss of a chemical from the test system for 10 days was generally larger than the simulated results. For example, the decrease in Csed of BaP (log
Kd: 4.9) was 36–40% of the initial concentration for 10 days in flow-through conditions, which was much higher than predicted by the model simulation (Fig. S8† and 5). In the future, the model can be refined by accounting for processes such as bioturbation, volatilization, bioaccumulation, and biotransformation. Microbial degradation in the surface sediment might explain the observed deviations for the PAHs tested.47
3.6. Suggestions for sediment toxicity tests and sediment risk assessments
This is the first study to demonstrate, both experimentally and through modeling, that the inflow of clean water to a spiked-sediment test system significantly reduced the concentrations of HOCs in overlying water but had no effect on concentrations in pore water, survival rates, and bioaccumulation in the epibenthic amphipod H. azteca. The absence of influence of water exchange on bioaccumulation was corroborated by the fact that the pore water concentration was a better indicator for the bioaccumulation of BaP than the overlying water concentrations. These findings would allow the use of the results obtained with different water exchange rates,28,30 in sediment risk assessments, provided that pore water determines the toxicity for a given species. That is, these findings indicate that a water exchange system can be used in spiked-sediment toxicity tests to supply oxygen and remove ammonia despite the decrease in chemical concentration in overlying water.
Author contributions
KH and FCF conceived and designed the study with comments from all other authors. KH and NT performed the experiments. FCF and KH performed the modeling analysis. KH wrote the original draft with comments and suggestions from FCF. All authors reviewed and edited the manuscript.
Conflicts of interest
The authors declare no conflicts of interest. The opinions expressed in this manuscript do not necessarily represent the official views of the authors' affiliation.
Acknowledgements
This research was financially supported by the Environment Research and Technology Development Fund (JPMEERF20195002) of the Environmental Restoration and Conservation Agency Provided by the Ministry of Environment of Japan. F. C. F. was funded by the Japan Society for the Promotion of Science (JSPS) Research Fellowship (20F20753). The authors are grateful to Kaori Saito for her help in culturing test organisms and conducting toxicity tests.
References
- E. Eek, G. Cornelissen and G. D. Breedveld, Field measurement of diffusional mass transfer of HOCs at the sediment-water interface, Environ. Sci. Technol., 2010, 44(17), 6752–6759, DOI:10.1021/es100818w.
- U. Ghosh, S. Kane Driscoll, R. M. Burgess, M. T. O. Jonker, D. Reible, F. Gobas, Y. Choi, S. E. Apitz, K. A. Maruya, W. R. Gala, M. Munro and C. Beegan, Passive sampling methods for contaminated sediments: practical guidance for selection, calibration, and implementation, Integr. Environ. Assess. Manage., 2014, 10(2), 210–223, DOI:10.1002/ieam.1507.
- A. Belles, C. Alary, J. Criquet, A. Ivanovsky and G. Billon, Assessing the transport of PAH in the surficial sediment layer by passive sampler approach, Sci. Total Environ., 2017, 579, 72–81, DOI:10.1016/j.scitotenv.2016.10.198.
- A. Dorn, K. Hammel, P. Dalkmann, D. Faber, E. Hellpointner, M. Lamshoeft, M. Telscher, E. Bruns, E. Seidel and H. Hollert, What is the actual exposure of organic compounds on Chironomus riparius? - A novel methodology enabling the depth-related analysis in sediment microcosms, Chemosphere, 2021, 279, 130424, DOI:10.1016/j.chemosphere.2021.130424.
- D. J. Lampert, W. V. Sarchet and D. D. Reible, Assessing the effectiveness of thin-layer sand caps for contaminated sediment management through passive sampling, Environ. Sci. Technol., 2011, 45(19), 8437–8443 CrossRef CAS PubMed.
- K. Hiki, F. C. Fischer, T. Nishimori, H. Watanabe, H. Yamamoto and S. Endo, Spatiotemporal Distribution of Hydrophobic Organic Contaminants in Spiked-Sediment Toxicity Tests: Measuring Total and Freely Dissolved Concentrations in Porewater and Overlying Water, Environ. Toxicol. Chem., 2021, 40(11), 3148–3158, DOI:10.1002/etc.5199.
- F. C. Fischer, K. Hiki, K. Soetaert and S. Endo, Mind the Exposure Gaps—Modeling Chemical Transport in Sediment Toxicity Tests, Environ. Sci. Technol., 2021, 55(17), 11885–11893, DOI:10.1021/acs.est.1c03201.
- U. Borgmann and W. Norwood, Sediment toxicity testing using large water–sediment ratios: an alternative to water renewal, Environ. Pollut., 1999, 106(3), 333–339, DOI:10.1016/S0269-7491(99)00104-9.
-
OECD, OECD Guidelines for the Testing of Chemicals, Section 2. Test No. 218: Sediment-Water Chironomid Toxicity Using Spiked Sediment, OECD, 2004, DOI:10.1787/9789264070264-en.
-
USEPA, Ecological Effects Test Guidelines: OCSPP 850.1735: Spiked Whole Sediment 10-Day Toxicity Test, Freshwater Invertebrates, 2016, vol. EPA-712-C Search PubMed.
- D. C. Zumwalt, F. J. Dwyer, I. E. Greer and C. G. Ingersoll, A water-renewal system that accurately delivers small volumes of water to exposure chambers, Environ. Toxicol. Chem., 1994, 13(8), 1311–1314 Search PubMed.
- Y. Zhang, D. A. Spadaro, J. J. King and S. L. Simpson, Improved prediction of sediment toxicity using a combination of sediment and overlying water contaminant exposures, Environ. Pollut., 2020, 266, 115187, DOI:10.1016/j.envpol.2020.115187.
- A. V. Nebeker, S. T. Onjukka, M. A. Cairns and D. F. Krawczyk, Survival of Daphnia magna and Hyalella azteca in cadmium-spiked water and sediment, Environ. Toxicol. Chem., 1986, 5(10), 933–938, DOI:10.1002/etc.5620051009.
- G. Ankley, D. Benoit, R. Hoke, E. Leonard, C. West, G. Phipps, R. Mattson and L. A. Anderson, Development and evaluation of test methods for benthic invertebrates and sediments: effects of flow rate and feeding on water quality and exposure conditions, Arch. Environ. Contam. Toxicol., 1993, 25(1), 627–635, DOI:10.1007/BF00230705.
- S. T. J. Droge, J. F. Postma and J. L. M. Hermens, Sediment Toxicity of a Rapidly Biodegrading Nonionic Surfactant: Comparing the Equilibrium Partitioning Approach with Measurements in Pore Water, Environ. Sci. Technol., 2008, 42(11), 4215–4221, DOI:10.1021/es702802p.
- F. W. Whiteman, G. T. Ankley, M. D. Kahl, D. M. Rau and M. D. Balcer, Evaluation of interstitial water as a route of exposure for ammonia in sediment tests with benthic macroinvertebrates, Environ. Toxicol. Chem., 1996, 15(5), 794–801 CrossRef CAS.
- A. J. Kirkpatrick, A. Gerhardt, J. T. A. Dick, M. McKenna and J. A. Berges, Use of the multispecies freshwater biomonitor to assess behavioral changes of Corophium volutator (Pallas, 1766) (Crustacea, Amphipoda) in response to toxicant exposure in sediment, Ecotoxicol. Environ. Saf., 2006, 64(3), 298–303 CrossRef CAS PubMed.
- S. B. Hawthorne, N. A. Azzolina, E. F. Neuhauser and J. P. Kreitinger, Predicting Bioavailability of Sediment Polycyclic Aromatic Hydrocarbons to Hyalella azteca Using Equilibrium Partitioning, Supercritical Fluid Extraction, and Pore Water Concentrations, Environ. Sci. Technol., 2007, 41(17), 6297–6304, DOI:10.1021/es0702162.
- M. J. Lydy, P. F. Landrum, A. M. Oen, M. Allinson, F. Smedes, A. D. Harwood, H. Li, K. A. Maruya and J. Liu, Passive sampling methods for contaminated sediments: state of the science for organic contaminants, Integr. Environ. Assess. Manage., 2014, 10(2), 167–178, DOI:10.1002/ieam.1503.
- S. Wang, W. Lao, H. Li and J. You, Measuring bioconcentration factors of sediment-associated fipronil in Lumbriculus variegatus using passive sampling techniques, J. Hazard. Mater., 2020, 393, 122420, DOI:10.1016/j.jhazmat.2020.122420.
- Y. Xu, F. Spurlock, Z. Wang and J. Gan, Comparison of Five Methods for Measuring Sediment Toxicity of Hydrophobic Contaminants, Environ. Sci. Technol., 2007, 41(24), 8394–8399, DOI:10.1021/es071911c.
- Y. Gu, T. Tobino and F. Nakajima, Determining the Relative Importance of Dietborne and Waterborne Toxicity of 4-tert-Butylphenol and 4-tert-Octylphenol to the Benthic Crustacean, Heterocypris incongruens, Environ. Sci. Technol., 2021, 55(12), 7939–7948, DOI:10.1021/acs.est.0c08164.
- J. D. Lebrun, D. Leroy, A. Giusti, C. Gourlay-Francé and J. P. Thomé, Bioaccumulation of polybrominated diphenyl ethers (PBDEs) in Gammarus pulex: relative importance of different exposure routes and multipathway modeling, Aquat. Toxicol., 2014, 154, 107–113, DOI:10.1016/j.aquatox.2014.05.015.
- X. Zhang, X. Xia, H. Li, B. Zhu and J. Dong, Bioavailability of Pyrene Associated with Suspended Sediment of Different Grain Sizes to Daphnia magna as Investigated by Passive Dosing Devices, Environ. Sci. Technol., 2015, 49(16), 10127–10135, DOI:10.1021/acs.est.5b02045.
- F. Fischer, L. Böhm, S. Höss, C. Möhlenkamp, E. Claus and R.-A. Düring,
et al., Passive Dosing in Chronic Toxicity Tests with the Nematode Caenorhabditis elegans, Environ. Sci. Technol., 2016, 50(17), 9708–9716, DOI:10.1021/acs.est.6b02956.
- H. Lin, X. Xia, S. Bi, X. Jiang, H. Wang, Y. Zhai and W. Wen, Quantifying Bioavailability of Pyrene Associated with Dissolved Organic Matter of Various Molecular Weights to Daphnia magna, Environ. Sci. Technol., 2018, 52(2), 644–653, DOI:10.1021/acs.est.7b05520.
- F. Wang, R. R. Goulet and P. M. Chapman, Testing sediment biological effects with the freshwater amphipod Hyalella azteca: the gap between laboratory and nature, Chemosphere, 2004, 57(11), 1713–1724, DOI:10.1016/j.chemosphere.2004.07.050..
-
Environment and Climate Change Canada, Biological test method: test for survival, growth, and reproduction in sediment and water using the freshwater amphipod Hyalella azteca, 2017, RM/33 Search PubMed.
-
USEPA, Methods for Measuring the Toxicity and Bioaccumulation of Sediment-Associated Contaminants with Freshwater Invertebrates, EPA/600/R-99/064, 2000.
-
ASTM, Standard test methods for measuring the toxicity of sediment-associated contaminants with freshwater invertebrates, in Annual Book of Standards, 2020, E1706-20 Search PubMed.
- A. V. Nebeker, G. S. Schuytema, W. L. Griffis, J. A. Barbitta and L. A. Carey, Effect of sediment organic carbon on survival of Hyalella azteca exposed to DDT and endrin, Environ. Toxicol. Chem., 1989, 8(8), 705–718, DOI:10.1002/etc.5620080808.
- S. J. Maund, M. J. Hamer, M. C. G. Lane, E. Farrelly, J. H. Rapley, U. M. Goggin and W. Getle, Partitioning, bioavailability, and toxicity of the pyrethroid insecticide cypermethrin in sediments, Environ. Toxicol. Chem., 2002, 21(1), 9–15, DOI:10.1002/etc.5620210102.
- S. Soroldoni, S. Vieira da Silva, Í. B. Castro, C. de Martinez Gaspar Martins and G. L. Leães Pinho, Antifouling paint particles cause toxicity to benthic organisms: effects on two species with different feeding modes, Chemosphere, 2020, 238, 1–9 CrossRef PubMed.
- G. R. Lotufo, P. F. Landrum and M. L. Gedeon, Toxicity and bioaccumulation of DDT in freshwater amphipods in exposures to spiked sediments, Environ. Toxicol. Chem., 2001, 20(4), 810–825 CrossRef CAS PubMed.
- C. de Perre, A. J. Trimble, J. D. Maul and M. J. Lydy, Ecological bioavailability of permethrin and p,p′-DDT: toxicity depends on type of organic matter resource, Chemosphere, 2014, 96, 67–73, DOI:10.1016/j.chemosphere.2013.07.030.
- D. P. Weston, J. You, A. D. Harwood and M. J. Lydy, Whole sediment toxicity identification evaluation tools for pyrethroid insecticides: III. Temperature manipulation, Environ. Toxicol. Chem., 2009, 28(1), 173–180 CrossRef CAS PubMed.
- B. Muijs and M. T. O. Jonker, Temperature-Dependent Bioaccumulation of Polycyclic Aromatic Hydrocarbons, Environ. Sci. Technol., 2009, 43(12), 4517–4523, DOI:10.1021/es803462y.
- T. Nishimori, K. Hiki, F. C. Fischer, S. Endo, H. Yamamoto and H. Watanabe, Comparing 10- and 28-Day Sediment Toxicity and Bioaccumulation of Fluoranthene in Hyalella azteca Using Passive Sampling Techniques, Environ. Toxicol. Chem., 2022, 41(11), 2679–2687, DOI:10.1002/etc.5460.
- K. Tani, H. Watanabe, M. Noguchi, K. Hiki, T. Yamagishi, N. Tatarazako and H. Yamamoto, Toxicity assessment of typical polycyclic aromatic hydrocarbons to Daphnia magna and Hyalella azteca in water-only and sediment–water exposure systems, Sci. Total Environ., 2021, 784, 147156, DOI:10.1016/j.scitotenv.2021.147156.
-
USEPA, Procedures for the Derivation of Equilibrium Partitioning Sediment Benchmarks (ESBs) for the Protection of Benthic Organisms: PAH Mixtures, EPA/600/R-02/013, 2003.
- M. S. Othman and D. Pascoe, Growth, Development and Reproduction of Hyalella azteca (Saussure, 1858) in Laboratory Culture, JSTOR, 2001, 74(2), 171 Search PubMed , https://www.jstor.org/stable/20106425.
- P. C. M. van Noort and A. A. Koelmans, Nonequilibrium of Organic Compounds in Sediment–Water Systems. Consequences for Risk Assessment and Remediation Measures, Environ. Sci. Technol., 2012, 46(20), 10900–10908, DOI:10.1021/es300630t.
- M. H. Lamy-Enrici, A. Dondeyne and E. Thybaud, Influence of the organic matter on the bioavailability of phenanthrene for benthic organisms, Aquat. Ecosyst. Health Manage., 2003, 6(4), 391–396 CrossRef CAS.
- C. Schlechtriem, S. Kampe, H.-J. Bruckert, I. Bischof, I. Ebersbach, V. Kosfeld, M. Kotthoff, C. Schäfers and J. L’Haridon, Bioconcentration studies with the freshwater amphipod Hyalella azteca: are the results predictive of bioconcentration in fish?, Environ. Sci. Pollut. Res., 2019, 26(2), 1628–1641, DOI:10.1007/s11356-018-3677-4.
- F. C. Fischer, K. Hiki and S. Endo, Free versus Bound Concentration: Passive Dosing from Polymer Meshes Elucidates Drivers of Toxicity in Aquatic Tests with Benthic Invertebrates, Environ. Toxicol. Chem., 2022, 1–10, DOI:10.1002/etc.5473.
- C. G. Ingersoll, N. Wang, J. M. R. Hayward, J. R. Jones, S. B. Jones and D. S. Ireland, A field assessment of long-term laboratory sediment toxicity tests with the amphipod Hyalella azteca, Environ. Toxicol. Chem., 2005, 24(11), 2853, DOI:10.1897/04-393R.1.
- W. S. Gardner, R. F. Lee, K. R. Tenore and L. W. Smith, Degradation of selected polycyclic aromatic hydrocarbons in coastal sediments: importance of microbes and polychaete worms, Water, Air, Soil Pollut., 1979, 11(3), 339–347, DOI:10.1007/BF00296591.
|
This journal is © The Royal Society of Chemistry 2023 |