Assessment of aldehyde contributions to PTR-MS m/z 69.07 in indoor air measurements
Received
12th April 2023
, Accepted 13th July 2023
First published on 25th July 2023
Abstract
Proton transfer reaction-mass spectrometry (PTR-MS) has been widely used for monitoring outdoor and indoor volatile organic compounds. For outdoor air, mass-to-charge-ratio m/z 69.07 is usually assigned to isoprene. Isoprene is also a major component of human breath and therefore abundant in occupied indoor environments. Mass 69.07 as an indicator of indoor isoprene can suffer interference resulting from fragmentation of aldehydes [V. Ruzsanyi, et al., Multi-capillary-column proton-transfer-reaction time-of-flight mass spectrometry, J. Chromatogr. A, 2013, 1316, 112–118], which are also abundant indoors, especially when ozone is elevated [C. J. Weschler, Roles of the human occupant in indoor chemistry, Indoor Air, 2016, 26, 6–24]. As part of the Indoor Chemical Human Emission and Reactivity (ICHEAR) campaign we examined this effect in human-occupied chamber studies, in the absence and presence of ozone. We find that such interferences do occur when ozone reacts with both human skin oil and cotton-based clothing. In the presence of humans and 35 ppb ozone, PTR-mass 69.07 was three times higher than the isoprene mixing ratio measured independently by GC-MS. To investigate this effect, we measured the fragmentation patterns of aldehydes and examined the contribution of different aldehydes to m/z 69.07 in the ICHEAR experiments. Nonanal, and its contribution to m/z 69.07, could be quantified reliably for clothing and human dermal emissions under the experimental conditions. In contrast, decanal is difficult to quantify, since compounds other than decanal fragment to m/z 157.16, its MH+ peak, which also makes it difficult to estimate decanal's contribution to m/z 69.07.
Environmental significance
PTR-ToF-MS is widely used for volatile organic compounds measurement. Mass-to-charge ratio m/z 69.07 is usually assigned to isoprene, which is emitted from vegetation. It is also a major component of human breath and therefore present in occupied indoor environments. Aldehydes can fragment onto the same exact mass leading to interference of the indoor isoprene measurement. This work studies the contribution of C5–C10 saturated aldehydes on PTR m/z 69.07 in occupied indoor environments, and investigates if the measurement of these aldehydes can be used to correct for their interference on the PTR isoprene mass. Nonanal and its contribution could be quantified reliably under the experimental conditions, while the quantification of the other aldehydes and their contribution to m/z 69.07 is more complicated.
|
1 Introduction
The measurement of volatile organic compounds (VOCs) in air is often performed using proton transfer reaction-mass spectrometry (PTR-MS) and gas chromatography-mass spectrometry (GC-MS). Generally, these techniques can generate high quality in situ measurement data down to ppt (parts per trillion) detection limits with a time resolution of minutes (GC) or seconds (PTR). PTR-MS uses a rather soft ionization technique, which usually results in little fragmentation of the analytes after protonation so that the signal is usually detected at the analyte compound mass plus the mass of one proton. But, depending on the chemical structure of the analyte and the settings of the instrument, fragmentation can occur. Therefore, care must be taken to minimize measurement artifacts.
Ozone can play an important role in measurement artifacts indoors (e.g. Ernle et al.1). It is ubiquitously present in outside tropospheric air and is transferred to indoor environments through passive or active ventilation. Indoors, ozone reacts rapidly, both on surfaces and in the gas phase, with compounds containing a double bond. Consequently, indoor concentrations tend to be 3–5 times lower than co-occurring outdoor concentrations.2 Humans are known to be a strong sink for indoor ozone, in particular through reactions with constituents of skin oils such as squalene and sapienic acid.3–5 Such reactions have the potential to interfere with mass spectrometric measurements of key reactive indoor compounds such as isoprene by generating additional signal on the target masses either directly or through fragmentation within the measurement device (as is the case for nonanal and decanal).
Isoprene is one of the most abundant reactive VOCs measured indoors when people are present.6 It is present in exhaled human breath7,8 and reacts rapidly with OH radicals and much less rapidly with ozone (O3). The isoprene loss due to OH and O3 depends on the reaction rate coefficient and the oxidant concentration. For typical indoor O3 concentrations, and for the conditions used in our experiments, the isoprene loss due to OH and O3 differs by at least one order of magnitude. Due to its large contribution to total indoor OH reactivity,9 isoprene needs to be accurately measured in studies of indoor OH dynamics. For a PTR-MS operating in the standard H3O+ mode, m/z 69.07 is usually assigned to isoprene.10 Early iterations of PTR-MS were equipped with a quadrupole detector allowing only 1 amu mass resolution, so that any species generating signals between 69 and 70 amu were potential interferents with the accurate quantification of isoprene. Prior outside air studies noted the potential of cyclopentene, furan, 2-methyl-3-buten-ol11 and even the minor constituent carbon suboxide (C3O212) to contribute to m/z 69. More recent versions of the PTR-MS have been equipped with a time of flight mass spectrometer capable of higher mass resolution allowing identification of the exact molecular formula; they are therefore less prone to interference through fragmentation. Nevertheless, compounds that can fragment onto the exact mass of protonated isoprene (m/z 69.07) can compromise the measurement.
Buhr et al.7 investigated volatile flavour compounds and found that C5 to C9 saturated aldehydes fragment onto mass 69.07, while their isomeric ketones do not. Ruzsanyi et al.13 studied fragmentation patterns with a multi-capillary-column PTR-ToF-MS and reported that the most abundant ion for C8 to C10 saturated aldehydes as well as for 3-methylbutanal was m/z 69.07. According to Gueneron et al.14 and Yuan et al.15 several cycloalkane species also produce fragments of the same mass.
In recent years, PTR-MS has been widely applied in indoor air research that involves VOC measurements.6,16–19 Aldehydes are common VOCs indoors.20 When ozone is present in a human occupied indoor environment, nonanal and decanal can be formed from the oxidation of cooking oils and human skin oils, which coat indoor surfaces, furnishings and clothing.5,21,22 Both aldehydes can contribute to m/z 69.07. Therefore, it is important to understand to what extent m/z 69.07 can originate from fragments when using PTR-MS (H3O+) to measure isoprene in occupied environments.
This study aims to assess the importance of potential interference effects in occupied indoor environments using data from both a PTR-MS and a GC-MS system. We have measured potential interferents from human beings under controlled climatic conditions in the absence and presence of ozone. A separate fragmentation test of several aldehydes was performed with the PTR-ToF-MS used during the ICHEAR campaign. The experiments were designed to (a) identify aldehydes that contribute to PTR m/z 69.07 in the ICHEAR studies, (b) quantify their contributions to this mass fragment and (c) determine if PTR-ToF-MS measurements of aldehydes and their fragments can be used to correct for their interference in indoor isoprene measurements.
2 Materials and methods
2.1 PTR-ToF-MS
VOC measurements were performed with the PTR-Tof-MS 8000 (IONICON Analytik, Austria). It was operated with standard operational settings in the ICHEAR study and the fragmentation experiment. The applied settings were: drift tube pressure pdrift 2.2 mbar, energy level in drift tube E/N 137 Td, and a drift temperature Tdrift of 60 °C. The fragmentation test was performed under a similar temperature (ca. 21 °C) than the ICHEAR experiments (25 °C, 31 °C), but different relative humidity (dry condition for fragmentation experiment, RH 25% and 65% during ICHEAR). The instrument was operated at 1 s time resolution. For the fragmentation experiment C5 to C10 aldehydes, which were all detected within the course of the ICHEAR experiment,6,9 were measured with PTR-ToF-MS in order to find the fragment masses of those compounds. Due to a lack of calibration gas standards, the VOC mixing ratio was calculated with the theoretical method described by Cappellin et al.23 where the measured counts were corrected by mass-dependent duty cycle. As C5 to C10 aldehydes are target compounds in this study, we used the proton transfer rate coefficient of those aldehydes (k-rate) proposed in Cappellin et al.23 for more accurate quantification. The uncertainty of the theoretic calculation is mainly from the calculated k, which is considered to be 10–15% from quantum chemical calculations.23 For the isoprene quantification during the ICHEAR experiments, using PTR-ToF-MS, the total uncertainty is 6%, including signal precision and calibration accuracy (gas standard uncertainty, flow measurement accuracy and calibration curve fitting uncertainty). Limits of detection (LOD, 3σ) were <0.043 ppb, with a total uncertainty of 15% during ICHEAR. For the fragmentation test, raw ion signals (counts per second, cps) were normalized by the sum of the primary reagent ion (H3O+) and the first water cluster ((H2O)2H+) and reported as normalized counts per second (ncps). The same normalization approach was also performed for the ICHEAR experiments for quantification. The total measurement uncertainty for the fragmentation test was usually ≤34%.
2.2 Fast GC-MS
Isoprene was measured with a custom-built fast GC-quadrupole-MS system previously described by Bourtsoukidis et al.24 The instrument includes a three-step cryogenic pre-concentration unit, which first collects the analytes before rapidly injecting them from the last trap into the GC-system (heating rate 100 °C s−1). The instrumental setup is equipped with an internal calibration system, which is used to perform calibrations with up to five different standard gas levels. A 4–5 point calibration was performed every day. With a chromatographic separation time of 2.5 min and a total GC-cycle time of 3 min the system is considered a quasi-online measurement device. Due to the high time resolution, the fast GC-MS currently cannot measure larger aldehydes (≥C7). Isoprene was detected in single ion monitoring (SIM) mode on m/z 68 and was nicely separated from adjacent peaks. The total measurement uncertainty was usually ≤10%. The propagation of uncertainty was calculated including the uncertainties of the calibration curve, the measured mixing ratio, the volumes in GC and calibration system, the isoprene mixing ratio in the calibration gas cylinder, and from the peak integration.
2.3 Experimental setup for the fragmentation test
A synthetic air flow was connected to a mass flow controller (MFC) which was set to 1 slpm. Shortly after the MFC, an injection T-piece was installed. It was made of Nylon connections with a septum, through which the needle of a gas syringe could be inserted. 5 to 25 μL gas phase headspace samples of the aldehydes were injected manually in the PTR-ToF-MS at least five times. Different volumes were chosen for different aldehydes due to their different vapor pressures. The goal was to get a reasonably high signal. Chemicals used for the aldehyde fragmentation test are shown in Table 1. This fragmentation test was performed under dry conditions and the results were used to determine the contribution of fragmentation to m/z 69.07 for the chamber experiments. A later set of fragmentation experiments were performed under different RH conditions (RH30 and RH60) for reference but not applied to the calculations because the detection efficiency for heavier compounds cannot be compared due to the replacement of the detector of the PTR-ToF-MS.
Table 1 Chemicals used for aldehyde fragmentation with PTR-ToF-MS. Concentrations were between 95 and 99%
Chemical compound |
Supplier |
Pentanal |
Merck |
Hexanal |
Aldrich |
Heptanal |
Aldrich |
Octanal |
Aldrich |
Nonanal |
Aldrich |
Decanal |
Alfa Aesar |
2.4 ICHEAR setup
Four human subjects were sitting in a stainless steel chamber with an air change rate of 3.2 h−1. The temperature and relative humidity set points in these experiments were ca. 25 °C/25% and 31 °C/65%. After three hours of measurement without ozone present, ozone was introduced with a target concentration in the occupied chamber of 35 ppb. The subjects were asked to use a provided set of fragrance-free personal care products and laundered, new cotton clothing. VOC emissions were measured with PTR-ToF-MS and GC-MS. In order to simplify the analysis, experiments without breath emissions (and therefore without isoprene) were first examined from the ICHEAR data set. These experiments included the investigation of four new and worn shirts, with and without ozone as well as experiments with human dermal and clothing emissions. The latter were obtained by having the exhalations from four human volunteers wearing masks transferred into a neighboring chamber so that the occupied chamber air was only influenced by dermal emissions. To investigate the humidity dependence, worn shirts were additionally measured in one experiment with three levels of RH (set points 30, 50, and 70%), each lasting at least 1.5 h. An overview of the here used ICHEAR experiments can be found in Table 2. From this data we identified possible aldehydes which fragment on PTR-ToF mass 69.07. More detailed information on the ICHEAR setup can be found in Bekö et al.25
Table 2 Overview of the experiments performed during the ICHEAR campaign
Experiment |
Ozone |
Temp./°C |
RH |
ICHEAR dermal |
No |
25 |
25 |
|
31 |
65 |
Yes |
25 |
25 |
|
31 |
65 |
ICHEAR shirts new |
No |
25 |
25 |
Yes |
25 |
25 |
ICHEAR shirts worn |
No |
25 |
25 |
Yes |
25 |
25, 30, 50, 70 |
ICHEAR whole body |
No |
25 |
25 |
Yes |
25 |
25 |
2.5 Calculation of aldehyde signals for ICHEAR experiments
The laboratory determined fragment ratios measured with the PTR-ToF-MS were compared with ratios obtained from ICHEAR data and used to calculate the contribution from aldehyde emission to measured mass 69.07 during ICHEAR. Additionally, an experiment with human whole-body emission (dermal and breath) has been added as a more complicated example. In PTR-MS the mass used for carbonyl detection is the mass of the protonated carbonyl compound (MH+). This mass actually includes the sum of aldehydes and ketones (e.g. pentanal and pentanone). Due to the fact that aldehydes are much more likely to lose a water molecule during ionization than ketones, the parental mass minus 18 is usually considered as a fragment of that aldehyde, which needs to be considered in quantification unless there are other interferences on this mass.7 In this study these masses will be called MH+-H2O. The fragment fractions f reported in this study are an average from five replicate measurements (see Fig. 1a exemplarily for nonanal). Peak integration was used to calculate the fraction of the specific fragment mass (see Fig. 1b for a more detailed plot of the nonanal fragment masses after injection): integration was performed between the two time points that define twice the detection limit (LOD = 3 × noise) of the m/z ratio with the lowest fraction (but ≥5% fragment fraction; for nonanal this is the grey line, m/z 125.136). The fragment fraction ffrag is described by the ratio of counts on the fragment (sfrag) and the sum of counts on all designated fragments (sCHO, see eqn (1)) of the same aldehyde (see eqn (2)). |  | (1) |
where sfragi is the signal (ncps) for fragment i of n fragments. For example, we chose n = 6 for the six observed fragments of nonanal (143.14, 125.14, 83.09, 69.07, 55.05 and 41.04). |  | (2) |
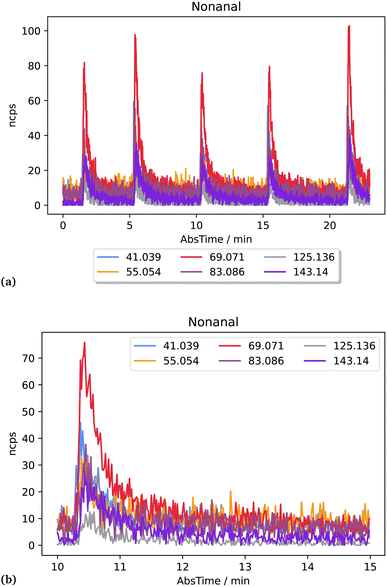 |
| Fig. 1 Signals of masses detected after injection of nonanal headspace samples (a) and a zoomed-in plot of one signal (b). | |
Using the fragment fraction ffrag of one aldehyde, the signal for mass 69.07 from a specific aldehyde (sm69) can be calculated with eqn (3) using the signal of the protonated aldehyde MH+ (sMH+) and the fraction of mass 69.07 (fm69) and MH+ (fMH+) as shown in eqn (3). The calculated m/z 69.07 signal can be used to estimate the contribution of the specific aldehyde to the total measured m/z 69.07 signal.
| 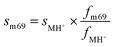 | (3) |
2.6 Evaluation of the fragment fractions
The fragment ratio RCHO has been calculated with eqn (4). It describes the ratio of the aldehyde's parental ion signal (sMH+) and the signal of its second mass fragment (sMH+-H2O). |  | (4) |
The ratio is used to estimate whether or not there is an interference on MH+ or MH+-H2O. It is not used in the calculation of the contribution of an aldehyde to m/z 69.07. If the ratio of those two fragment signals from the same aldehyde is the same in real world measurements than in the fragmentation test (where a single measurement of the aldehyde was performed without other compounds interfering), it can be assumed that there is no interference on neither MH+ nor MH+-H2O. In case of interference of other compounds, it is unlikely that the ratio of the two fragments will stay the same. This ratio for the ICHEAR experiments discussed in the present paper has been compared to the ratio from the fragmentation test (see Table 4, Section 3.2). Additionally, the coefficient of determination R2 has been used to estimate the reliability of RCHO. The Pearson correlation coefficient r was obtained for the correlation of the signals on MH+ with MH+-H2O for each single ICHEAR experiment. The average of all the squared correlation coefficients (average of the coefficients of determination R2) is used for the estimation of the reliability of RCHO. If the correlation is strong (coefficient of determination R2 ≥ 0.90) and the ratio is the same over several experiments including the fragmentation test, it can be assumed that there is no interference on the masses used for aldehyde detection. Please note that for experimental setups with different RH (e.g. ICHEAR shirts worn), an average ratio has been used.
3 Results and discussion
3.1 Aldehyde fragmentation fractions
Table 3 shows the fragment fractions for C5 to C10 saturated aldehydes obtained from the fragmentation test of the present study and other studies using PTR-MS. If Buhr et al.7 or Ruzsanyi et al.13 observed fragments which were not observed in the present study, only masses with fractions ≥10% are listed. Ruzsanyi et al.13 reported only the most abundant fragments. The fractions of mass 69.07 are similar, while generally the studies of Buhr et al.7 and Ruzsanyi et al.13 report higher fractions for masses larger than 69.07 amu. The probability of fragmentation increases with the E/N value. Ruzsanyi et al.13 measured at E/N 140 Td which is comparable to our value of 137 Td. Unfortunately, Buhr et al.7 did not report their E/N value. According to Schwarz et al.,26 the water concentration in the ion source also influences the fragmentation. Additionally the ratio of the water cluster to protonated water (m/z 37/m/z 19) should be comparable when comparing fragmentation patterns. This information was not available for the studies of Buhr et al. and Ruzsanyi et al. Therefore, care must be taken when interpreting the values in Table 3.
Table 3 Measured fragment masses and their fractional contribution to the total, ±standard deviations, for C5 to C10 aldehydes as measured in the fragmentation test (this study) or reported in the literature
Aldehyde |
Reference |
Fragment masses [amu] and percent of total |
MH+ |
MH+-H2O |
Decanal |
|
157.16
|
139.15
|
97.11
|
83.09
|
69.07
|
55.05
|
|
|
this study |
12.9 ± 4.4 |
2.8 ± 1.1 |
11.2 ± 2.0 |
33.1 ± 3.9 |
10.8 ± 2.1
|
29.1 ± 3.8 |
|
|
Ruzsanyi et al. |
4 |
— |
12 |
66 |
18
|
— |
|
|
Nonanal |
|
143.14
|
125.14
|
85.08
|
83.09
|
69.07
|
55.05
|
41.049
|
|
This study |
12.9 ± 3.9 |
5.4 ± 2.0 |
— |
13.8 ± 3.9 |
34.7 ± 4.2
|
15.1 ± 3.2 |
18.1 ± 4.5 |
|
Buhr et al. |
19 |
11 |
— |
15 |
36
|
3 |
3 |
|
Ruzsanyi et al. |
22 |
— |
14 |
23 |
40
|
— |
— |
|
Octanal |
|
129.13
|
111.12
|
69.07
|
55.05
|
41.04
|
|
|
|
This study |
8.3 ± 1.7 |
17.7 ± 3.6 |
46.2 ± 2.8
|
9.8 ± 1.3 |
18.0 ± 3.6 |
|
|
|
Buhr et al. |
11 |
38 |
40
|
2 |
3 |
|
|
|
Heptanal |
|
115.11
|
97.11
|
69.07
|
55.05
|
41.04
|
|
|
|
This study |
4.8 ± 1.1 |
47.2 ± 2.5 |
4.8 ± 0.6
|
40.8 ± 2.8 |
2.4 ± 0.6 |
|
|
|
Buhr et al. |
5 |
61 |
4
|
26 |
— |
|
|
|
Hexanal |
|
101.10
|
83.09
|
69.07
|
59.05
|
57.07
|
55.05
|
45.03
|
41.03
|
This study |
4.1 ± 1.1 |
37.4 ± 6.7 |
1.5 ± 1.2
|
5.0 ± 3.6 |
1.7 ± 1.3 |
40.2 ± 5.9 |
7.3 ± 5.2 |
2.7 ± 1.3 |
Buhr et al. |
— |
63 |
—
|
— |
— |
29 |
— |
— |
Pentanal |
|
87.08
|
69.07
|
45.03
|
41.04
|
|
|
|
|
This study |
5.1 ± 1.3 |
58.7 ± 3.6
|
7.4 ± 0.7 |
25.6 ± 3.0 |
|
|
|
|
Buhr et al. |
— |
71
|
4 |
15 |
|
|
|
|
Table 4 Ratio RCHO of the signals of MH+ over MH+-H2O, as well as average coefficient of determination (R2) between MH+ and MH+-H2O, for C5–C10 aldehydes as measured in the fragmentation test and ICHEAR experiments (see Table 2). Average values are displayed with their standard deviation
Experiment |
R
pentanal
|
R
hexanal
|
R
heptanal
|
R
octanal
|
R
nonanal
|
R
decanal
|
Fragmentation
|
0.10 ± 0.01
|
0.11 ± 0.00
|
0.10 ± 0.01
|
0.47 ± 0.02
|
2.38 ± 0.15
|
4.68 ± 0.57
|
ICHEAR shirts new |
0.10 ± 0.07 |
0.13 ± 0.06 |
0.28 ± 0.12 |
0.65 ± 0.25 |
2.12 ± 0.67 |
— |
ICHEAR shirts worn |
0.08 ± 0.03 |
— |
0.16 ± 0.02 |
0.48 ± 0.02 |
2.36 ± 0.35 |
9.48 ± 0.29 |
ICHEAR dermal |
0.12 ± 0.00 |
0.16 ± 0.01 |
0.19 ± 0.02 |
0.31 ± 0.14 |
2.19 ± 0.07 |
8.36 ± 0.69 |
ICHEAR whole body |
0.09 ± 0.09 |
— |
0.19 ± 0.20 |
0.48 ± 1.50 |
2.31 ± 6.13 |
9.62 ± 20.66 |
Average
|
0.10 ± 0.02
|
0.15 ± 0.02
|
0.19 ± 0.04
|
0.45 ± 0.14
|
2.26 ± 0.10
|
9.06 ± 0.74
|
Average R2 |
0.65 ± 0.25 |
0.94 ± 0.02 |
0.57 ± 0.33 |
0.81 ± 0.14 |
0.94 ± 0.07 |
0.89 ± 0.07 |
3.2 Comparison of fragment fractions
Our own tests indicate that the fraction of mass at MH+ and MH+-H2O does increase with relative humidity, while their ratio (RCHO as defined in eqn (4)) showed no clear humidity dependence. Therefore, the RCHO from the fragmentation test can be compared with RCHO from ICHEAR. For hexanal, heptanal and decanal the average RCHO from ICHEAR are not in line with RCHO from the fragmentation test. This indicates that there are fragments from other compounds on MH+ or MH+-H2O. The highest difference of RCHO from ICHEAR compared to the fragmentation test is obtained for decanal and heptanal, where Rdecanal is 4.68 ± 0.57 and Rheptanal is 0.10 ± 0.01 for the fragmentation test while it is 9.06 ± 0.74 and 0.19 ± 0.04 for the ICHEAR data respectively. Possible candidates responsible for this difference are isomeric compounds as well as C10H20OH+ and C7H14OH+ fragments from larger compounds. It is more likely that other compounds fragment on mass 157.16 and 115.11 under the experimental conditions of ICHEAR as indicated by the high RCHO values. Thus, assigning m/z 157.16 to decanal alone would result in an artifactually high concentration. Possible fragments on m/z 157.16 can be ketones with ten carbon atoms, as well as menthol27 and dihydromyrcenol,28 which are used as fragrance ingredients in soaps and detergents. For heptanal, fragments could potentially come from ketones with seven carbon atoms.
The difference in RCHO between ICHEAR and the fragmentation test is below the standard deviation for pentanal, octanal and nonanal. But, the coefficients of determination R2 for pentanal, heptanal and octanal are lower (between 0.57 and 0.81) than R2 of the other aldehydes, indicating a rather weak correlation of MH+ with MH+-H2O.
The only compound where RCHO from the ICHEAR experiments is in line with the fragmentation experiment and the coefficient of determination is ≥0.90 is nonanal (ICHEAR Rnonanal 2.26 ± 0.10 compared to fragmentation test Rnonanal 2.38 ± 0.15, R2 = 0.94 ± 0.07). This means that for nonanal interference of other compounds on MH+ or MH+-H2O (m/z 143.14, m/z 125.14) is unlikely. In summary, we consider the results of nonanal reliable, while those of the other C5–C10 aldehydes are likely to be defective.
The fragmentation test was not performed under perfect conditions as there were possible impurities in the head space sample from other chemicals. For quality assurance, zero air was injected after several injections of each aldehyde. These syringe background samples have not shown a substantial increase in the monitored aldehyde masses. The fragmentation test delivered quite similar results for the five injections made per compound with the standard deviation usually below 25% and the fragment fractions are comparable to those reported by other groups.7,13 Therefore, the fragmentation test is considered to be accurate enough for the results presented in Table 3 to be considered reliable.
3.3 Measured and calculated signals of nonanal in indoor air
During the ICHEAR campaign, we measured emissions in the absence and presence of ozone, from (i) human body excluding breath (dermal), (ii) new t-shirts, (iii) worn t-shirts and (iv) human bodies including breath (whole body). Results from these separate experiments, for steady-state conditions, are shown in Fig. 2. The yellow bars show the percent of the total measured signal for m/z 69.07 due to nonanal as calculated using eqn (3). Generally, for dermal and shirt experiments in the absence of ozone, nonanal contributes between 40 and 60% to the measured signal resulting from all gas-phase compounds with fragments at m/z 69.07. For the experiment on human whole body emission, nonanal contributes only about 5%. In contrast, in the presence of ozone, nonanal contributes between 55% and 100% to the measured signal from all compounds at m/z 69.07 for dermal and shirts experiments and ca. 35% for the whole body conditions. In other words, during periods of elevated ozone, nonanal is the dominant contributor to the mass-to-charge-ratio which is commonly used to quantify isoprene under conditions excluding human breath and it is still contributing more than one 3rd to the total m/z 69.07 during measurement of human whole body emissions.
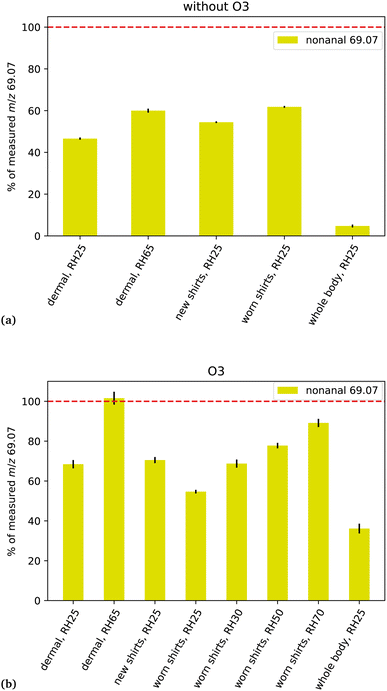 |
| Fig. 2 For different ICHEAR experiments (see Table 2), the yellow bars indicate the percent of the total measured signal for m/z 69.07 due to nonanal as calculated using eqn (3). (a) In the absence of ozone; (b) in the presence of ozone. The small black lines indicate the standard deviation. | |
3.3.1 Influence of relative humidity.
The nonanal signal increases with relative humidity. In the case of emissions from human bodies excluding breath (dermal experiments in Fig. 2), m/z 69.07 from nonanal was a larger fraction of the total measured m/z 69.07 at 65% RH than at 25% RH. Both, for absent and present ozone. This is consistent with observations that humidity suppresses formation of stable secondary ozonides in triolein;29 triolein and related unsaturated fats are an important source of ozone-derived nonanal. Relatedly, Arata et al.30 observed increased concentrations of carbonyls for the squalene ozone reaction with increased humidity. It is also consistent with the results summarized in the critical review by Coffaro and Weisel16 of ozone/squalene chemistry. Besides changes in ozonolysis chemistry with RH, the humidity dependence of a PTR-MS system can also play a role. It is known that signals from a PTR-MS using H3O+ ions are humidity dependent for some VOCs. Trefz et al.31 reported increasing signal intensities for aldehydes as humidity increased. This is in line with our own tests, which showed increasing intensities for high fragment masses (including MH+) and lower intensities for smaller fragment masses of the same compound. It is unclear to what extent the observed relative humidity effects are attributable to ozonolysis chemistry or less fragmentation with increasing humidity. In the ICHEAR study, the measured signals of nonanal were not corrected for the humidity dependence due to a lack of single component gas standards. Therefore, aldehydes' RH-dependency could lead to an overestimation of m/z 69.07 from nonanal. Both, RH-dependent ozonolysis experiments and the aldehydes' RH-dependency should be investigated to better estimate the relative contribution of these two effects. Note: the calculated contribution of nonanal to m/z 69.07 may be high, since the ratio of the fragment fractions (fm69/fMH+) used for the eqn (3) calculation are from the fragmentation test, which was performed under dry conditions. Although Rnonanal does not show a humidity dependence, fm69/fm143.14 decreases with RH. Based on our tests, the expected maximum decrease of this fraction is ca. 30%.
3.3.2 Human dermal and clothing emission.
Focusing on the clothing experiments in which isoprene from breath emissions is absent (Fig. 2a), it can be seen that with and without ozone, m/z 69.07 from nonanal at RH ≤ 30% accounts for roughly 50–60% of the measured m/z 69.07. For the experiment on human dermal emission at RH 25%, which excludes breath emissions by design, the contribution from nonanal is ca. 45% at the lower RH and 60% at the higher RH. In Fig. 2b it can be seen clearly that the presence of 35 ppb of ozone leads to the emission of aldehydes in all experimental setups. Nonanal and decanal are primary products of the reaction of skin oil and clothing fabric with ozone. The mixing ratios of those products depend on the difference between inlet and outlet ozone mixing ratio (100–35 ppb = 65 ppb). Therefore, if we assume a much smaller difference that may occur during the evening, such as 10 ppb of O3, the contribution of the aldehydes would be approximately seven times lower than what we found in this study. In measurements of soiled t-shirts emissions absent and present ozone, Rai et al.21 found that nonanal and decanal emissions increased with ozone, RH and the soiling level of t-shirts.
3.3.3 Human whole body emission (dermal and breath).
The fraction of fragmentation signal on m/z 69.07 from nonanal without ozone present is significantly lower for the whole body experiment compared to other experiments. This is mainly due to isoprene, a component of human breath, which contributes to m/z 69.07 in the whole body emissions experiments, but not in the dermal-only or clothing experiments. A timeline from the whole body (both, dermal and breath emissions) experiment, without and with ozone, is shown in Fig. 3. In the morning (09:30–12:30), the red line (measured m/z 69.07) is mainly from isoprene in breath. The contribution of nonanal (yellow) to the m/z 69.07 signal is negligible. Aldehydes are not expected to be present in large amounts without ozone. In the afternoon (13:00–16:00) somewhat similar isoprene mixing ratios as in the morning would be expected, and this was the case for measurements by the fast GC-MS system. However, in the case of PTR-MS measurements, after ozone was switched on the green line (measured m/z 69.07 minus contribution from nonanal) is roughly 20 ncps higher than the morning signal. The results from the whole body experiment, which includes isoprene from breathing, show that the interpretation of the m/z 69.07 signal becomes more difficult with an increasing number of VOC sources, especially when ozone is present.
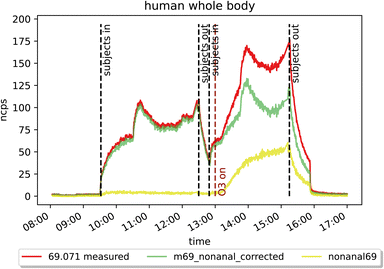 |
| Fig. 3 Signals of measured m/z 69.07 (red), calculated m/z 69.07 from nonanal (yellow) and m/z 69.07 corrected by the contribution of nonanal (green) from the ICHEAR whole body experiment (dermal and breath emission). | |
Ozonolysis of VOCs from different sources including skin oils, cooking oils and colorants for fabric produces many different compounds including aldehydes.19,21,22,32 This leads to additional compounds which were not considered in this study that may also fragment onto m/z 69.07, MH+-H2O or MH+. Ozonolysis of compounds coming from the clothing fabric (cotton in the present case) can result in emission of nonanal and we can quantify nonanal's contribution to m/z 69.07. However, our results show, that if we consider only nonanal, we are missing other sources of m/z 69.07 fragments. Numerous studies have reported the production of decanal from the reaction of O3 with skin oil.5 For this reason, decanal is also found on soiled clothing.19,22,32 In the present study we measured ions which are usually assigned to decanal (MH+m/z 157.16 and MH+-H2O m/z 139.15), but our experiments have shown interference from other compounds on those masses. Hence, we cannot reliably quantify the contribution of decanal to m/z 69.07.
3.4 Comparison of GC-isoprene with PTR-m/z 69.07
Results from human whole body emission experiments in the presence of ozone show large discrepancy in isoprene emission measured by GC-MS and PTR-Tof-MS even after accounting for the interference from nonanal on PTR isoprene mass (m/z 69.07) as can be seen in Fig. 4. In the morning, when O3 was not present in the chamber, the signals agree well, while after ozone was switched on at around 13:00 (reaching ∼35 ppb), they differ substantially. The PTR-ToF-MS detects roughly 40% higher “isoprene” mixing ratio compared to the GC. Before subtracting the amount of m/z 69.07 which was produced from nonanal, the PTR-ToF-MS measured more than double the GC measured mixing ratio when O3 was present. For our conditions, 1 ppb nonanal contributes to m/z 69.07 equivalent to approximately 1.45 ppb isoprene. However, there are still missing contributors for a reliable quantification of isoprene in the presence of ozone (e.g. decanal as discussed in Section 3.3.3). The inlet and outlet O3 mixing ratios in the ICHEAR experiments result in a difference of about 65 ppb. For a hypothetical night-time indoor–outdoor difference of 10 ppb, the interference would be approximately 1/7th of that measured in this study. This would still result in ca. 0.5 ppb of “false isoprene” as the real isoprene mixing ratio was 3.5 ppb with four people in the chamber during the human whole body experiment.
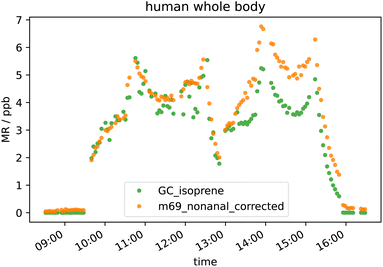 |
| Fig. 4 Mixing ratios (MR) of isoprene from the ICHEAR whole body experiment measured with GC-MS (green circles) and PTR-ToF-MS (orange circles). The PTR-ToF-MS values have been corrected for the interference produced from nonanal. | |
4 Conclusions
Our experiments have shown that for an occupied indoor environment, isoprene cannot be quantified reliably with PTR-MS in H3O+ mode, since there are other compounds which can fragment on m/z 69.07. This is the case with humans as the primary emission source – other emission sources such as furniture, cooking or cleaning products were not present in this study. The same applies if aldehydes or cycloalkanes from other sources are expected to be present in the sample air, since these compounds are known to fragment on the mass commonly attributed to isoprene in the PTR-MS community. If outdoor ozone concentrations are much lower, resulting in lower indoor product formation rates, the interference on m/z 69.07 due to products of ozone chemistry would be lower. Therefore, for the quantification of isoprene indoors, it is recommended to use PTR-MS with NO+ as the primary reagent ion or a different, more specific measurement technique such as GC-MS, which pre-separates the chemicals prior to mass spectrometric detection. However, for low ozone conditions and absent aldehyde emission sources, the contribution of compounds other than isoprene to m/z 69.07 may be negligible.
From the data it can be seen that for nonanal, the ratio Rnonanal (i.e. MH+ over MH+-H2O) from ICHEAR experiments agrees with the fragmentation test. Hence, those masses could be used for quantification of nonanal under the ICHEAR conditions and they can be used to quantify the contribution of nonanal to PTR m/z 69.07. In contrast, decanal from the ICHEAR data set shows an Rdecanal value that is almost double the value obtained from direct injection of the analyte. This leads to the conclusion that there might be a significant amount of C10 ketones produced or other compounds such as menthol or dihydromyrcenol that fragment on mass 157.16 within the context of indoor human emissions. Hence, the contribution of decanal to m/z 69.07 in indoor air measurements cannot be accurately quantified. The ratios RCHO for hexanal and heptanal are also not in line with the ratio from the fragmentation test experiment and can therefore not be used to calculate these aldehydes' contribution to m/z 69.07 due to other compounds interfering on MH+ or MH+-H2O. The ratio of pentanal and octanal fit the fragmentation test, but the standard deviation for Roctanal is very high (±0.13 for an average of Roctanal of 0.45) and the coefficient of determination indicates a weak correlation for both, pentanal and octanal. The majority of the human-derived interferences to m/z 69.07 stem from the ozonolysis of human skin oil and cotton clothing (prewashed in fragrance free detergent) that generates nonanal. Most likely, significant additional interference was provided by decanal which was almost exclusively generated from ozone reactions with skin oil.
Further experiments and calculations should be performed to better determine under which conditions the aldehyde contribution to m/z 69.07 can be quantified with PTR-MS in H3O+ mode. The humidity dependence of fragmentation needs to be studied in detail to better understand the change in fragmentation pattern induced by change in humidity. This information would further improve the accuracy of the calculated contribution of nonanal to m/z 69.07, as well as the quantification of nonanal itself. Additionally, it would be helpful to estimate an ozone guideline value below which isoprene can still be measured reliably with PTR-MS in H3O+ mode since this is a standard measurement technique used for indoor measurements.
Data availability
The data are published at https://doi.org/10.5281/zenodo.8169026 (Ernle and Wang, 2023).
Author contributions
LE and NW designed the experiment, performed the measurements and analyzed the data. LE wrote the manuscript draft, NW, GB, GCM, PW, CJW and JW reviewed and edited the manuscript.
Conflicts of interest
There are no conflicts of interest to declare.
Acknowledgements
The ICHEAR experiment was funded by the Alfred P. Sloan Foundation.
References
- L. Ernle, M. A. Ringsdorf and J. Williams, Influence of ozone and humidity on PTR-MS and GC-MS VOC measurements with and without Na2S2O3 ozone scrubber, Atmos. Meas. Tech., 2023, 16(5), 1179–1194 CrossRef CAS.
- W. W. Nazaroff and C. J. Weschler, Indoor ozone: Concentrations and influencing factors, Indoor Air, 2022, 32, e12942 CAS.
- C. Arata, N. Heine, N. Wang, P. K. Misztal, P. Wargocki, G. Bekö, J. Williams, W. W. Nazaroff, K. R. Wilson and A. H. Goldstein, Heterogeneous ozonolysis of squalene: gas-phase products depend on water vapor concentration, Environ. Sci. Technol., 2019, 53, 14441–14448 CrossRef CAS PubMed.
- C. J. Weschler, Roles of the human occupant in indoor chemistry, Indoor Air, 2016, 26, 6–24 CrossRef CAS PubMed.
- C. J. Weschler and W. W. Nazaroff, Human skin oil: a major ozone reactant indoors, Environ. Sci.: Atmos., 2023, 3(4), 640–661 CAS.
- N. Wang, L. Ernle, G. Bekö, P. Wargocki and J. Williams, Emission Rates of Volatile Organic Compounds from Humans, Environ. Sci. Technol., 2022, 56, 4838–4848 CrossRef CAS PubMed.
- K. Buhr, S. van Ruth and C. Delahunty, Analysis of volatile flavour compounds by Proton Transfer Reaction-Mass Spectrometry: fragmentation patterns and discrimination between isobaric and isomeric compounds, Int. J. Mass Spectrom., 2002, 221, 1–7 CrossRef CAS.
- J. D. Fenske and S. E. Paulson, Human breath emissions of VOCs., J. Air Waste Manage. Assoc., 1999, 49, 594–598 CrossRef CAS PubMed.
- N. Wang, N. Zannoni, L. Ernle, G. Bekö, P. Wargocki, M. Li, C. J. Weschler and J. Williams, Total OH reactivity of emissions from humans: In situ measurement and budget analysis, Environ. Sci. Technol., 2020, 55, 149–159 CrossRef PubMed.
- A. M. Yáñez-Serrano, I. Filella, J. LLusià, A. Gargallo-Garriga, V. Granda, E. Bourtsoukidis, J. Williams, R. Seco, L. Cappellin and C. Werner,
et al., GLOVOCS-Master compound assignment guide for proton transfer reaction mass spectrometry users, Atmos. Environ., 2021, 244, 117929 CrossRef.
- J. Williams, U. Pöschl, P. Crutzen, A. Hansel, R. Holzinger, C. Warneke, W. Lindinger and J. Lelieveld, An atmospheric chemistry interpretation of mass scans obtained from a proton transfer mass spectrometer flown over the tropical rainforest of Surinam, J. Atmos. Chem., 2001, 38, 133–166 CrossRef CAS.
- S. Keßel, D. Cabrera-Perez, A. Horowitz, P. R. Veres, R. Sander, D. Taraborrelli, M. Tucceri, J. N. Crowley, A. Pozzer and C. Stönner,
et al., Atmospheric chemistry, sources and sinks of carbon suboxide, C3O2, Atmos. Chem. Phys., 2017, 17, 8789–8804 CrossRef.
- V. Ruzsanyi, L. Fischer, J. Herbig, C. Ager and A. Amann, Multi-capillary-column proton-transfer-reaction time-offlight mass spectrometry, J. Chromatogr. A, 2013, 1316, 112–118 CrossRef CAS PubMed.
- M. Gueneron, M. H. Erickson, G. S. VanderSchelden and B. T. Jobson, PTR-MS fragmentation patterns of gasoline hydrocarbons, Int. J. Mass Spectrom., 2015, 379, 97–109 CrossRef CAS.
- B. Yuan, C. Warneke, M. Shao and J. A. De Gouw, Interpretation of volatile organic compound measurements by proton-transfer-reaction mass spectrometry over the deepwater horizon oil spill, Int. J. Mass Spectrom., 2014, 358, 43–48 CrossRef CAS.
- B. Coffaro and C. P. Weisel, Reactions and Products of Squalene and Ozone: A Review, Environ. Sci. Technol., 2022, 56, 7396–7411 CrossRef CAS PubMed.
- D. K. Farmer, M. E. Vance, J. P. Abbatt, A. Abeleira, M. R. Alves, C. Arata, E. Boedicker, S. Bourne, F. Cardoso-Saldaña and R. Corsi,
et al., Overview of HOMEChem: House observations of microbial and environmental chemistry, Environ. Sci.: Processes Impacts, 2019, 21, 1280–1300 RSC.
- M. Rizk, F. Guo, M. Verriele, M. Ward, S. Dusanter, N. Blond, N. Locoge and C. Schoemaecker, Impact of material emissions and sorption of volatile organic compounds on indoor air quality in a low energy building: Field measurements and modeling, Indoor Air, 2018, 28, 924–935 CrossRef CAS PubMed.
- A. Wisthaler, G. Tamás, D. P. Wyon, P. Strøm-Tejsen, D. Space, J. Beauchamp, A. Hansel, T. D. Märk and C. J. Weschler, Products of ozone-initiated chemistry in a simulated aircraft environment, Environ. Sci. Technol., 2005, 39, 4823–4832 CrossRef CAS PubMed.
- H. J. Salonen, A.-L. Pasanen, S. K. Lappalainen, H. M. Riuttala, T. M. Tuomi, P. O. Pasanen, B. C. Bäck and K. E. Reijula, Airborne concentrations of volatile organic compounds, formaldehyde and ammonia in Finnish office buildings with suspected indoor air problems, J. Occup. Environ. Hyg., 2009, 6, 200–209 CrossRef CAS PubMed.
- A. Rai, B. Guo, C.-H. Lin, J. Zhang, J. Pei and Q. Chen, Ozone reaction with clothing and its initiated VOC emissions in an environmental chamber, Indoor Air, 2014, 24, 49–58 CrossRef CAS PubMed.
- C. J. Weschler, A. Wisthaler, S. Cowlin, G. Tamás, P. Strøm-Tejsen, A. T. Hodgson, H. Destaillats, J. Herrington, J. Zhang and W. W. Nazaroff, Ozone-initiated chemistry in an occupied simulated aircraft cabin, Environ. Sci. Technol., 2007, 41, 6177–6184 CrossRef CAS PubMed.
- L. Cappellin, T. Karl, M. Probst, O. Ismailova, P. M. Winkler, C. Soukoulis, E. Aprea, T. D. Märk, F. Gasperi and F. Biasioli, On quantitative determination of volatile organic compound concentrations using proton transfer reaction time-of-flight mass spectrometry, Environ. Sci. Technol., 2012, 46, 2283–2290 CrossRef CAS PubMed.
- E. Bourtsoukidis, F. Helleis, L. Tomsche, H. Fischer, R. Hofmann, J. Lelieveld and J. Williams, An aircraft gas chromatograph–mass spectrometer System for Organic Fast Identification Analysis (SOFIA): design, performance and a case study of Asian monsoon pollution outflow, Atmos. Meas. Tech., 2017, 10, 5089–5105 CrossRef CAS.
- G. Bekö, P. Wargocki, N. Wang, M. Li, C. J. Weschler, G. Morrison, S. Langer, L. Ernle, D. Licina and S. Yang,
et al., The Indoor Chemical Human Emissions and Reactivity (ICHEAR) project: Overview of experimental methodology and preliminary results, Indoor Air, 2020, 30, 1213–1228 CrossRef PubMed.
- K. Schwarz, W. Filipiak and A. Amann, Determining concentration patterns of volatile compounds in exhaled breath by PTR-MS, J. Breath Res., 2009, 3, 027002 CrossRef CAS PubMed.
- P. Spanel and D. Smith, SIFT studies of the reactions of H3O+, NO+ and O2+ with a series of alcohols, Int. J. Mass Spectrom. Ion Processes, 1997, 167, 375–388 CrossRef.
- D. I. Campbell, J. K. Dalgleish, I. Cotte-Rodriguez, S. Maeno and R. Graham Cooks, Chemical analysis and chemical imaging of fragrances and volatile compounds by lowtemperature plasma ionization mass spectrometry, Rapid Commun. Mass Spectrom., 2013, 27, 1828–1836 CrossRef CAS PubMed.
- Z. Zhou, S. Zhou and J. P. Abbatt, Kinetics and condensedphase products in multiphase ozonolysis of an unsaturated triglyceride, Environ. Sci. Technol., 2019, 53, 12467–12475 CrossRef CAS PubMed.
- C. Arata, P. K. Misztal, Y. Tian, D. M. Lunderberg, K. Kristensen, A. Novoselac, M. E. Vance, D. K. Farmer, W. W. Nazaroff and A. H. Goldstein, Volatile organic compound emissions during HOMEChem, Indoor Air, 2021, 31, 2099–2117 CrossRef CAS PubMed.
- P. Trefz, J. K. Schubert and W. Miekisch, Effects of humidity, CO2 and O2 on real-time quantitation of breath biomarkers by means of PTR-ToF-MS, J. Breath Res., 2018, 12, 026016 CrossRef PubMed.
- B. K. Coleman, H. Destaillats, A. T. Hodgson and W. W. Nazaroff, Ozone consumption and volatile byproduct formation from surface reactions with aircraft cabin materials and clothing fabrics, Atmos. Environ., 2008, 42, 642–654 CrossRef CAS.
|
This journal is © The Royal Society of Chemistry 2023 |