DOI:
10.1039/D3CE00358B
(Paper)
CrystEngComm, 2023,
25, 4496-4502
Designing scintillating coordination polymers using a dual-ligand synthetic approach†
Received
13th April 2023
, Accepted 3rd July 2023
First published on 11th July 2023
Abstract
Herein we report the synthesis and structural characterization of three new europium containing coordination polymers: [Eu(terpy)(2,6-ndc)1.5]·H2O (Linus Pauling (LP) 1), [Eu(terpy)(1,4-ndc)(1,4-Hndc)] (LP-2), and [Eu(phen)(2,6-ndc)1.5]·DMF (LP-3). The title compounds were prepared hydro- or solvo-thermally and are constructed from either 1,4- or 2,6-naphthalenedicarboxylic acid (ndc) and 1,10-phenanthroline (phen) or 2,2′:6′,2′′-terpyridine (terpy) ligands. The X-ray diffraction data show LP-2 is two-dimensional while LP-1 and LP-3 are three-dimensional. Compounds LP-1 and LP-2 exhibit modest thermal stability up to 400 °C, while LP-3 was notably unstable when removed from its reaction media and prone to degradation. Despite the apparent porosity, nitrogen physisorption measurements conducted on LP-1 and LP-2 revealed lower than anticipated surface area and pore volumes of 1 m2 g−1 and 0.037 cm3 g−1 and 1 m2 g−1 and 0.015 cm3 g−1, respectively. Photoluminescence characterization of all three compounds was performed in the solid state and in all cases the results reveal bright Eu3+-based emission. The excitation spectra show the emissive properties arise from efficient ligand-to-metal resonance energy transfer. Additionally, LP-1 and LP-2 also display emission when exposed to soft X-rays, and as such show promise toward the design of scintillators.
Introduction
Lanthanide bearing coordination polymers (CP) and metal–organic frameworks (MOFs) continue to draw interest from the material science community owing to their promise in applications that span microelectronics, sensors, catalysis, and many others.1–9 This breadth of functionality originates from the rich structural chemistry, and indeed diversity of reported structure types,10–12 combined with the inherent electronic and physical properties of the 4f elements.13 For example, the framework materials containing europium (Eu3+) have garnered much attention for luminescence-based applications. Irradiation of such materials with a variety of excitation sources, such as ultraviolet light, X-rays, and α, β, and γ radiation (radioluminescence),14,15 have resulted in bright metal-ion emission, as is the case for various chemical reactions (chemiluminescence)16,17 and mechanical processess.18–20 These properties have been broadly studied in multiple systems, including sol–gel glass, liquid crystals, and doped ceramics, for applications such as temperature indicators, barcoded materials,21–23 and piezoelectric sensors.24–29 Regardless of the application or underlying mechanism, maximizing the efficiency of the emissive process is of critical importance.
The molar absorptivity of the 4f–4f transitions that give rise to lanthanide luminescence are small because they are formally forbidden by the electronic selection rules.30 Thus, direct excitation of the Eu3+ metal ions (via the 4f–4f transitions) typically results in weak or inefficient luminescence emission. This is commonly overcome in small molecule and framework materials by sensitizing (or directly populating) the emissive state of the lanthanide ion via the antenna effect.31,32 In short, an organic chromophore is leveraged to harvest photon energy that is used to populate the excited state of the metal ion through resonance energy transfer. This approach has been fruitful in preparing numerous lanthanide containing systems with high quantum efficiency.33,34
Recently we reported a series of lanthanide containing frameworks that were constructed of either chelating 1,10-phenanthroline (phen) or 2,2′:6′,2′′-terpyridine (terpy) ‘capping’ ligands and 2,2′-bipyridine-4,4′-dicarboxylic acid (bpdc) ligand ‘linkers’.35 The combination of the phen and bpdc ligands was of particular note because the synthesized material was porous, and exhibited unique thermal properties and highly efficient Eu3+-based emission. While there are a number of reported lanthanide/bpdc containing CPs and MOFs, many are of limited porosity.36–38 The reason is that the spherical coordination environment of the lanthanide elements, when paired with a linear, bidentate ligand, (e.g., bpdc), tends to promote dense or polycatenated structure types.39,40 The dual-ligand approach has been recognized elsewhere as a potential path forward for promoting more open architectures.41 Briefly, the chelating ligand will occupy one side of the metal center, disrupting the spherical coordination motif, while the second linear ‘linker’ ligand provides additional connectivity. The added steric bulk of the chelating ligand also has a secondary role in preventing the formation of dense interconnected frameworks or promoting connectivity via secondary noncovalent interactions.42,43 Aside from these structural implications, the resulting material functionality tends to diversify, given that the properties of each ligand become integrated with those of the metal center.44–47
Herein we have explored the design of high dimensional network solids by leveraging a dual-ligand approach. The choice of the chelating phen and terpy ligands was strategic as both are known to be efficient sensitizers of the Eu3+ emissive state.30 The 1,4- and 2,6-naphthalenedicarboxylic acid (ndc) ligands were chosen not only for their role in luminescence sensitization, but also because similar species have been utilized in materials known to autoluminescent or function as an X-ray scintillator.48 This synthetic approach led to the formation of three novel Eu3+ bearing CPs: LP-1: [Eu(terpy)(2,6-ndc)1.5]·H2O, LP-2: [Eu(1,4-ndc)(1,4-Hndc)(terpy)], LP-3: [Eu(phen)(2,6-ndc)1.5]·DMF that all exhibit bright red Eu3+-based emission. The X-ray diffraction data reveals the structure types are of two (LP-1) and three (LP-2 and LP-3) dimensionalities and contain potential void spaces. The optical data reveal that luminescence properties stem from efficient ligand-to-metal resonance energy transfer. Notably, the study of the materials by powder X-ray diffraction (PXRD) reveal bright Eu3+-based emission throughout the duration of the experiment with no signs of quenching or bleaching.
Synthesis
The title compounds were synthesized hydro- or solvo-thermally within a 23 mL Teflon-lined acid digestion vessel. Europium chloride hexahydrate (EuCl3·6H2O) was combined with terpy and 2,6-ndc (LP-1) or 1,4-ndc (LP-2) in ultrapure water (H2O). LP-3 was synthesized by combining EuCl3·6H2O, phen and 2,6-ndc in dimethylformamide (DMF). The reactions were sealed and heated statically to 110 °C for 70 hours, and thereafter allowed to cool to room temperature. Crystals of LP-1 were harvested from the mother liquor and were washed with ultrapure H2O and concentrated ammonium hydroxide. The material was then rinsed with ethanol and dried. Crystals of LP-2 were washed with ethanol only. Compound LP-3 was unstable in air and was therefore stored in fresh DMF. Single crystals of the title compounds were harvested from the bulk product and prepared for X-ray diffraction analysis (Table S1†). In addition, washed samples of LP-1 and LP-2 were characterized by PXRD, thermogravimetric analysis (TGA) and nitrogen physisorption measurements, attenuated total reflectance Fourier transform infrared spectroscopy (ATR-FTIR), diffuse reflectance (DR), and photoluminescence (PL) spectroscopies. The PL measurements for LP-3 were performed on single crystals that were suspended within DMF. Additional synthetic and experimental details are provided in the ESI.†
Results and discussion
Crystal structure descriptions
Compound LP-1, [(Eu(terpy)(2,6-ndc)1.5)·H2O], crystallizes in the P
space group and features nine-coordinate metal centers, with each metal center bound to three nitrogen and six oxygen atoms to form a distorted tricapped trigonal prismatic geometry, with local C1 symmetry, Fig. 1 and S2.† Each metal center is coordinated by one terpy (κ3) and four total 2,6-ndc ligands. Two of the 2,6-ndc ligands feature bridging monodentate (μ2, κ1) coordination while the others are (κ2) chelating. The average Eu3+–N and Eu3+–O bond distances are 2.617(1) Å and 2.463(1) Å, respectively. The bond distances are typical for Eu3+–N and Eu3+–O bonds located within the Cambridge Crystallographic Data Centre (CCDC 2021.3.0, Mogul).49 The 2,6-ndc ligands bridge the neighboring metal centers together, forming pseudo-dimeric units. Each unit is then linked to a total of four others, giving rise to a chain-like motif that propagates along the [010] direction. The chains are linked together by the 2,6-ndc ligands to form a porous three-dimensional structure type, with a mog type topology.50–52 The pores feature 14 × 10 Å apertures and propagate along [100], and contain solvent water molecules.
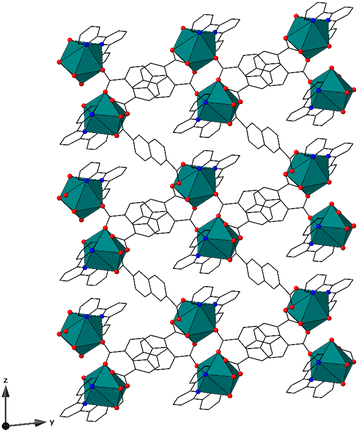 |
| Fig. 1 The structure of LP-1 is three-dimensional and contains small pores that propagate along the [100] direction. The Eu3+ atoms are drawn as teal polyhedra. The O and N atoms are depicted as red and blue spheres, respectively, and the C atoms are represented by black sticks. The H atoms and solvent water molecules are omitted for clarity here and throughout this manuscript. | |
Compound LP-2, [Eu(terpy)(1,4-ndc)(1,4-Hndc)], crystallizes in the P21/n space group and contains nine-coordinate metal centers that feature a distorted tricapped trigonal prismatic geometry with approximate C2 symmetry, Fig. 2 and S3.† Each Eu3+ metal center is bound to three nitrogen and six oxygen atoms. The Eu3+ metal centers are coordinated by one terpy (κ3) and four total 1,4-ndc ligands, the latter in κ2 chelating (two) and κ1 (two) monodentate fashions. The average Eu3+–N and Eu3+–O bond distances are 2.571(2) Å and 2.438(2) Å. Similar to LP-1, the bond distances are unremarkable. The κ2-1,4-ndc ligands connect the metal centers together to form sheets that span the (010) set of planes. The two κ1-coordinating 1,4-ndc ligands are terminal and are oriented toward one another, creating a head-on C–H⋯O hydrogen bond motif. The terpy capping ligands form offset π-interactions with those from adjacent sheets to create a supramolecular three-dimensional network with a sql type topology.50–52 The sheets are arranged such that they create potential void spaces in the [010] direction that feature ∼11.5 × 7 Å apertures. Noncoordinated guest species were not located within the voids, and we note that the pore volume is small, comprising only 3% of the unit cell by volume.
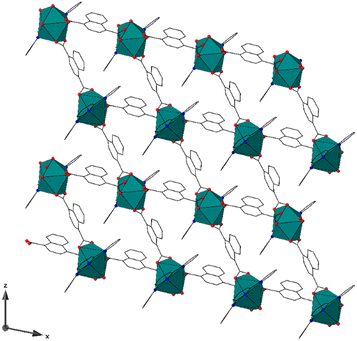 |
| Fig. 2 Compound LP-2 exhibits a two-dimensional structure type that contains potential void spaces along [010]. | |
Compound LP-3, [(Eu(phen)(2,6-ndc)1.5)·DMF], crystallizes in the P
space group and contains eight-coordinate metal centers, with each metal center bound to two nitrogen and six oxygen atoms to form a distorted dodecahedral (bisdisphenoid) geometry with C1 symmetry, Fig. 3 and S4.† Each metal center is coordinated by one phen (κ2) and four 2,6-ndc ligands in μ2,- κ1 monodenate bridging (two) and κ2 chelating modes. The average Eu3+–N and Eu3+–O bond distances are 2.586(7) Å and 2.404(6) Å, respectively, and are within the expected range. The metal centers form dimeric nodes through linkages created by the 2,6-ndc ligands, similar to those in LP-1. These nodes are coordinated to a total of two others and form chain-like motifs that propagate along the [001] direction. The chains are linked together by the 2,6-ndc ligands to form a three-dimensional framework with potential voids (along [001]) that have 13 Å × 9 Å apertures, Fig. 3. The framework features a mog type topology.50–52
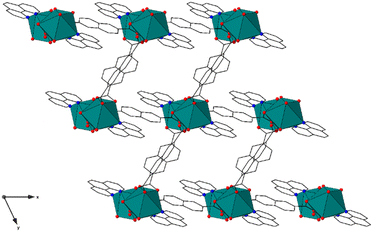 |
| Fig. 3 The structure of LP-3 is three-dimensional. The channels contain noncoordinated DMF guest species, which are not shown for clarity. | |
Surface area analysis
Nitrogen gas physisorption measurements on LP-1 and LP-2 were conducted at 77 K to calculate surface area and pore volume, Fig. S5 and S6.† Powdered samples were analyzed after a 12 hour outgassing cycle at 100 °C under vacuum. The adsorption isotherm of LP-1 reveals the Brunauer–Emmett–Teller (BET) surface area and pore volume are unexpectedly low at 1 m2 g−1 and 0.037 cm3 g−1, respectively. This result is surprising given that the three-dimensional structure of LP-1 features 14 × 10 Å large apertures and potential void space that measures 1363 Å3 per unit cell (as calculated using Platon).53 The analysis of compound LP-2, a two-dimensional structure type with pseudo-pores (with 11.5 × 7 Å apertures) yields a similar result. The surface area and pore volume is 1 m2 g−1and 0.015 cm3 g−1, respectively. While these results were admittedly somewhat unexpected, we suspect the sterically large terpy capping ligands in LP-1 and LP-2 protrude into the pore spaces, and are responsible for the lower than expected surface area values. Attempts to remove the terpy ligands prior to analysis via thermal treatment to test this hypothesis were unsuccessful.
Thermogravimetric analysis
The thermal stability of LP-1 and LP-2 were evaluated in air (21% O2(g)) and Ar(g), Fig. S7.† The thermal decomposition of LP-1 occurs in three distinct steps at temperatures of 290 °C, 435 °C, and 465 °C in air and 290 °C, 435 °C, and 605 °C in Ar(g). In each case, the first mass loss equates to about 5% by weight, which roughly correlates with four water molecules per unit cell, suggesting a mix of solvent and surface adhered species. The second and third decreases in mass are attributed to the decomposition of the terpy and 2,6-ndc ligands, respectively. Alternatively, LP-2 decomposed in a two-step process, Fig. S7.† These steps occur at 365 °C and 450 °C in air and 365 °C and 580 °C in Ar(g). In both experiments, the first change in mass is attributed to the decomposition of the terpy ligands, while the second is the 1,4-ndc linker.
Experiments to explore the controlled (thermal) removal of the terpy ligands in LP-2 was explored because of the modest window of stability (when compared to LP-1) that exists between 360–405 °C. A sample of LP-2 was heated to 380 °C for 1 hour, and thereafter analyzed by PXRD, Fig. S9.† A total loss in material crystallinity was observed, indicating that the capping ligands could not be removed without compromising the crystal structure. This is not entirely surprising given the pore volume is small and not likely large enough to accommodate the loss of the bulky ligands. We point this out because in a past contribution we demonstrated in a similar material that the capping ligands could be post-synthetically removed thermally, with little effect on the crystallinity. This approach was used as a strategy for material activation, and had a measurable impact on the end-use of radionuclide sequestration.35
Diffuse reflectance spectroscopy
The DR spectra of LP-1 and LP-2 were collected on powdered samples at room temperature, Fig. 4. Compound LP-1 exhibits a strong, broad absorption feature below 370 nm that originates from the terpy and 2,6-ndc ligands. Superimposed are the Eu3+-based 4f–4f transitions at 466, 526, 533, and 539 nm. These bands correspond to transitions between the 7F0 and 7F1 ground states and the 5D1–2 excited states, Fig. 4.30,54,55 The DR spectrum of LP-2 is similar and dominated by a strong, broad absorbance band between 390 and 300 nm. Again, sharp Eu3+-based 4f–4f transitions are present at 466, 526, 533, and 539 nm. The ligand-based absorption features were used to select the excitation wavelengths for the complementary PL measurements. The results for LP-1 and LP-2 were used to infer behavior for LP-3, which was not studied by DR.
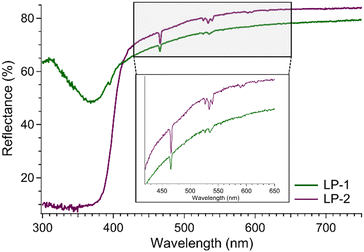 |
| Fig. 4 DR spectra of LP-1 and LP-2 collected at 298 K on powdered samples. | |
Photoluminescence spectroscopy
The title compounds were studied by PL spectroscopy using an excitation wavelength of 370 nm for LP-1 and LP-3, and 390 nm for LP-2. Each material produced Eu3+-based emission with the expected transitions from the 5D0 excited state to the 7F0–4 ground state manifold at 579 nm (7F0), 590 nm (7F1), 619 nm (7F2), 649 nm (7F3), and 695 nm (7F4), Fig. 5 and Table S2.†55 Weak ligand-based emission is observed in LP-2 only, suggesting efficient ligand-to-metal resonance energy transfer, Fig. S13.† Additional experiments on LP-1–LP-3 conducted using an excitation wavelength of 466 nm (the Eu3+ 5D2 ← 7F0 transition) results in a stark decrease to the emission intensity, further highlighting the effect of sensitization, Fig. S12–S14.† The excitation spectra of LP-1–LP-3 were collected while monitoring the 7F2 ← 5D0 transition at 614 nm, Fig. 5 and Table S2.† Compounds LP-1 and LP-3 have similar excitation spectra, exhibiting strong ligand-based features between 320 and 380 nm and Eu3+-based transitions at 395 nm (5L6 ← 7F0), 416 nm (5D3 ← 7F0), 466 nm (5D2 ← 7F0), 526 nm (5D1 ← 7F0), and 535 nm (5D6 ← 7F0).56 The excitation spectra of LP-2 reveals the ligand contribution is red shifted related to LP-1 and LP-2, and covers 324 to 399 nm. The Eu3+-based transitions are again observed at 466 nm (5D2 ← 7F0), 526 nm (5D1 ← 7F0) and 535 nm (5D6 ← 7F1). The red shift of the ligand absorption in LP-2 is likely a result of the protonated 1,4-ndcH ligand in the crystal structure.57 Luminescence lifetime data were collected on LP-1 and LP-2 only, Fig. S15 and S16,† and in each case the decay curves were fit with a monoexponential model. The results are 1.062 × 10−3 s for LP-1 and 1.001 × 10−4 s for LP-2, suggesting the resonance energy transfer pathway in the former is more efficient.
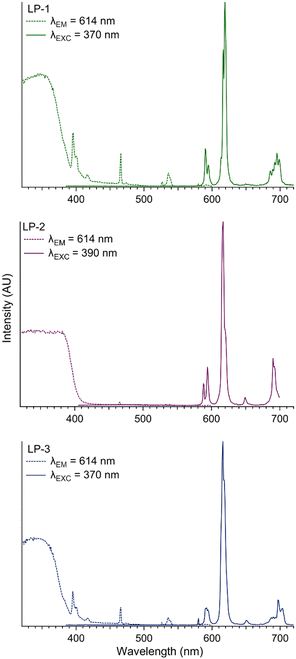 |
| Fig. 5 The luminescence spectra of LP-1, LP-2, and LP-3 collected at 298 K. | |
X-ray scintillation
Compounds LP-1 and LP-2 scintillate upon exposure to soft X-rays (Cu Kα, 8.04 keV). The result is bright pink emission that is visible to the naked eye, Fig. S17.† While reports of scintillating lanthanide containing CPs and framework materials are rising,58–60 they remain underexplored and likely underreported. Published examples of lanthanide CPs that scintillate range in dimensionality and almost always feature Eu3+ or Tb3+ ions paired with aromatic chromophores.35,61–65 For example, Wang et al. reported Tb3+ and Eu3+ isostructural scintillating CPs that were constructed from oxalate and phen ligands.61 In the same study, a similar Eu3+ CP that contained only the oxalate ligand did not exhibit analogous behavior; thus, the phen ligands are thought to serve an essential mechanistic role. It is hypothesized that the interaction of the Eu3+ and Tb3+ metal centers with the X-rays creates a fast electron (i.e., the photoelectric effect) that is then transferred to the aromatic ligands. In turn, the ligands sensitize the emissive state of the metal centers via resonance energy transfer, causing either red (Eu3+) or green (Tb3+) emission. Here we note that reports of scintillating (or autoluminescent) compounds containing phen, terpy, and ndc have been reported.6,35 The electronic origin of this phenomenon was not explored here, yet we note the blueprint for preparing these materials a priori is being developed.
Conclusions
Presented here is the synthesis and structural characterization of three novel Eu3+ containing CPs that were assembled using a dual-ligand synthetic approach. This strategy is proving effective for promoting higher dimensional network solids and preventing the formation of dense, interwoven structure types. Here, aromatic ligand chromophores were leveraged to sensitize the emissive states of the Eu3+ metal centers, and as a result, the title compounds were highly luminescent and showed promise for X-ray detection. For the latter, the ligands are hypothesized to play important roles in the design of scintillating lanthanide containing framework materials. More broadly, these so-called designer frameworks are becoming more relevant given their promise towards applications that extend past fundamental science, and into commercial radiation detection.
Author contributions
The manuscript was written with contributions from all authors.
Conflicts of interest
There are no conflicts to declare.
Acknowledgements
The primary funding mechanism for this study was the Laboratory Directed Research and Development Program at Pacific Northwest National Laboratory, a multiprogram national laboratory operated by Battelle for the Department of Energy. AA, AL, and RGS are all grateful for support from the Linus Pauling Distinguished Postdoctoral Fellowship. M. N. and A. A. acknowledges support from DOE's National Nuclear Security Administration (NNSA) for work conducted at Oregon State University, award number DE-NA0003763. The authors thank Michael A. Sinnwell for assistance in collecting the nitrogen physisorption measurements, guidance in assigning topological descriptors, and Santiago Alvarez for the helpful discussions on symmetry and geometry.
References
- J. A. Smith, M. A. Singh-Wilmot, K. P. Carter, C. L. Cahill and J. A. Ridenour, Cryst. Growth Des., 2019, 19, 305–319 CrossRef CAS.
- Y. Hasegawa and T. Nakanishi, RSC Adv., 2015, 5, 338–353 RSC.
- S. A. Younis, N. Bhardwaj, S. K. Bhardwaj, K.-H. Kim and A. Deep, Coord. Chem. Rev., 2021, 429, 213620 CrossRef CAS.
- T. Gorai, W. Schmitt and T. Gunnlaugsson, Dalton Trans., 2021, 50, 770–784 RSC.
- Y. Zhang, S. Liu, Z.-S. Zhao, Z. Wang, R. Zhang, L. Liu and Z.-B. Han, Inorg. Chem. Front., 2021, 8, 590–619 RSC.
- H. Chen, J. Chen, M. Li, M. You, Q. Chen, M. Lin and H. Yang, Sci. China: Chem., 2022, 65, 2338–2350 CrossRef CAS.
-
B. Li and B. Chen, in Lanthanide Metal-Organic Frameworks, ed. P. Cheng, Springer Berlin Heidelberg, Berlin, Heidelberg, 2015, pp. 75–107, DOI:10.1007/430_2014_159.
- S. Sahoo, S. Mondal and D. Sarma, Coord. Chem. Rev., 2022, 470, 214707 CrossRef CAS.
- Y. Hasegawa and Y. Kitagawa, J. Photochem. Photobiol., C, 2022, 51, 100485 CrossRef CAS.
- B. Li, H.-M. Wen, Y. Cui, G. Qian and B. Chen, Prog. Polym. Sci., 2015, 48, 40–84 CrossRef CAS.
-
S. Fordham, X. Wang, M. Bosch and H.-C. Zhou, in Lanthanide Metal-Organic Frameworks, ed. P. Cheng, Springer Berlin Heidelberg, Berlin, Heidelberg, 2015, pp. 1–27, DOI:10.1007/430_2014_162.
- A. M. Spokoyny, D. Kim, A. Sumrein and C. A. Mirkin, Chem. Soc. Rev., 2009, 38, 1218–1227 RSC.
-
K. P. Carter and C. L. Cahill, in Handbook on the Physics and Chemistry of Rare Earths, ed. J.-C. Bünzli and V. K. Pecharsky, Elsevier, 2015, vol. 47, pp. 147–208 Search PubMed.
- E. A. Seregina, A. A. Seregin and G. V. Tikhonov, High Energy Chem., 2002, 36, 223–228 CrossRef CAS.
- E. Zych, M. Wójtowicz, A. Dobrowolska and L. Kępiński, Opt. Mater., 2009, 31, 1764–1767 CrossRef CAS.
- M. Elbanowski, B. Mąkowska, K. Staninski and M. Kaczmarek, J. Physiol. Pharmacol. Adv., 2000, 130, 75–81 CAS.
- M. Kaczmarek, J. Lumin., 2020, 222, 117174 CrossRef CAS.
- S. V. Eliseeva, D. N. Pleshkov, K. A. Lyssenko, L. S. Lepnev, J.-C. G. Bünzli and N. P. Kuzmina, Inorg. Chem., 2010, 49, 9300–9311 CrossRef CAS PubMed.
- Y. Hirai, T. Nakanishi, Y. Kitagawa, K. Fushimi, T. Seki, H. Ito and Y. Hasegawa, Angew. Chem., 2017, 129, 7277–7281 CrossRef.
- Y. Hasegawa, R. Hieda, K. Miyata, T. Nakagawa and T. Kawai, Eur. J. Inorg. Chem., 2011, 2011, 4978–4984 CrossRef CAS.
- M.-L. Gao, W.-J. Wang, L. Liu, Z.-B. Han, N. Wei, X.-M. Cao and D.-Q. Yuan, Inorg. Chem., 2017, 56, 511–517 CrossRef CAS PubMed.
- Y. Zhang, S. Yuan, G. Day, X. Wang, X. Yang and H.-C. Zhou, Coord. Chem. Rev., 2018, 354, 28–45 CrossRef CAS.
- S. V. Eliseeva and J.-C. G. Bünzli, Chem. Soc. Rev., 2010, 39, 189–227 RSC.
- H. R. Li, J. Lin, H. J. Zhang, L. S. Fu, Q. G. Meng and S. B. Wang, Chem. Mater., 2002, 14, 3651–3655 CrossRef CAS.
- K. Binnemans, P. Lenaerts, K. Driesen and C. Görller-Walrand, J. Mater. Chem., 2004, 14, 191–195 RSC.
- K. Binnemans and C. Görller-Walrand, Chem. Rev., 2002, 102, 2303–2346 CrossRef CAS PubMed.
- P. Yan, Y. Qin, Z. Xu, F. Han, Y. Wang, Z. Wen, Y. Zhang and S. Zhang, ACS Appl. Mater. Interfaces, 2021, 13, 54210–54216 CrossRef CAS PubMed.
- K. Li, E. Sun, Y. Zhang, Z. Song, X. Qi, Y. Sun, J. Li, B. Yang, J. Liu and W. Cao, J. Mater. Chem. C, 2021, 9, 2426–2436 RSC.
- Y. Zhai, J. Du, C. Chen, W. Li and J. Hao, J. Alloys Compd., 2020, 829, 154518 CrossRef CAS.
- K. Binnemans, Coord. Chem. Rev., 2015, 295, 1–45 CrossRef CAS.
- E. G. Moore, A. P. S. Samuel and K. N. Raymond, Acc. Chem. Res., 2009, 42, 542–552 CrossRef CAS PubMed.
- Y. Ma and Y. Wang, Coord. Chem. Rev., 2010, 254, 972–990 CrossRef CAS.
- E. E. S. Teotonio, G. M. Fett, H. F. Brito, W. M. Faustino, G. F. de Sá, M. C. F. C. Felinto and R. H. A. Santos, J. Lumin., 2008, 128, 190–198 CrossRef CAS.
- Y. Hasegawa, S. Tateno, M. Yamamoto, T. Nakanishi, Y. Kitagawa, T. Seki, H. Ito and K. Fushimi, Chem. – Eur. J., 2017, 23, 2666–2672 CrossRef CAS PubMed.
- R. G. Surbella III, D. D. Reilly, M. A. Sinnwell, B. K. McNamara, L. E. Sweet, J. M. Schwantes and P. K. Thallapally, ACS Appl. Mater. Interfaces, 2021, 13, 45696–45707 CrossRef CAS PubMed.
- Z. Min, M. A. Singh-Wilmot, C. L. Cahill, M. Andrews and R. Taylor, Eur. J. Inorg. Chem., 2012, 2012, 4419–4426 CrossRef CAS.
- J.-Y. Wu, T.-T. Yeh, Y.-S. Wen, J. Twu and K.-L. Lu, Cryst. Growth Des., 2006, 6, 467–473 CrossRef CAS.
- R.-F. Li, Y.-W. Zhang, X.-F. Liu, X.-H. Chang and X. Feng, Inorg. Chim. Acta, 2020, 502, 119370 CrossRef CAS.
- A. R. K. Chatenever, L. R. Warne, J. E. Matsuoka, S. J. Wang, E. W. Reinheimer, P. LeMagueres, H. Fei, X. Song and S. R. J. Oliver, Cryst. Growth Des., 2019, 19, 4854–4859 CrossRef CAS.
- X. Guo, G. Zhu, F. Sun, Z. Li, X. Zhao, X. Li, H. Wang and S. Qiu, Inorg. Chem., 2006, 45, 2581–2587 CrossRef CAS PubMed.
- K. P. Carter, C. H. F. Zulato, E. M. Rodrigues, S. J. A. Pope, F. A. Sigoli and C. L. Cahill, Dalton Trans., 2015, 44, 15843–15854 RSC.
- H. He, D. Yuan, H. Ma, D. Sun, G. Zhang and H.-C. Zhou, Inorg. Chem., 2010, 49, 7605–7607 CrossRef CAS PubMed.
- J.-F. Qian, W.-J. Tian, S. Yang, Z.-H. Sun, L. Chen, M.-J. Wei, Z. Wu, M.-Y. He, Z.-H. Zhang and L. Mei, Inorg. Chem., 2020, 59, 17659–17670 CrossRef CAS PubMed.
- H. Dong, L. Zhao, Y. Chen, M. Li, W. Chen, Y. Wang, X. Wei, Y. Zhang, Y. Zhou and M. Xu, Anal. Chem., 2022, 94, 11940–11948 CrossRef CAS PubMed.
- L. Yu, L. Feng, L. Xiong, S. Li, Q. Xu, X. Pan and Y. Xiao, ACS Appl. Mater. Interfaces, 2021, 13, 11646–11656 CrossRef CAS PubMed.
- Y.-Q. Sun, Y. Cheng and X.-B. Yin, Anal. Chem., 2021, 93, 3559–3566 CrossRef CAS PubMed.
- N. A. Ashashi, Z. U. Nisa, R. Singhaal, C. Sen, M. Ahmad, A. Frontera and H. N. Sheikh, ACS Omega, 2022, 7, 41370–41391 CrossRef CAS PubMed.
- L. E. Kreno, K. Leong, O. K. Farha, M. Allendorf, R. P. Van Duyne and J. T. Hupp, Chem. Rev., 2012, 112, 1105–1125 CrossRef CAS PubMed.
- C. R. Groom, I. J. Bruno, M. P. Lightfoot and S. C. Ward, Acta Crystallogr., Sect. B: Struct. Sci., Cryst. Eng. Mater., 2016, 72, 171–179 CrossRef CAS PubMed.
- E. V. Alexandrov, A. P. Shevchenko and V. A. Blatov, Cryst. Growth Des., 2019, 19, 2604–2614 CrossRef CAS.
- V. A. Blatov, A. P. Shevchenko and D. M. Proserpio, Cryst. Growth Des., 2014, 14, 3576–3586 CrossRef CAS.
- M. O'Keeffe, M. A. Peskov, S. J. Ramsden and O. M. Yaghi, Acc. Chem. Res., 2008, 41, 1782–1789 CrossRef PubMed.
- A. L. Spek, Acta Crystallogr., Sect. D: Biol. Crystallogr., 2009, 148–155 CrossRef CAS PubMed.
- W. T. Carnall, P. R. Fields and K. Rajnak, J. Chem. Phys., 1968, 49, 4412–4423 CrossRef CAS.
-
J.-C. G. Bünzli and S. V. Eliseeva, in Lanthanide Luminescence: Photophysical, Analytical and Biological Aspects, ed. P. Hänninen and H. Härmä, Springer Berlin Heidelberg, Berlin, Heidelberg, 2011, pp. 1–45, DOI:10.1007/4243_2010_3.
- Z. Mu, Y. Hu, L. Chen, X. Wang, G. Ju, Z. Yang and R. Chen, Ceram. Int., 2014, 40, 2575–2579 CrossRef CAS.
- E. Badaeva, V. V. Albert, S. Kilina, A. Koposov, M. Sykora and S. Tretiak, Phys. Chem. Chem. Phys., 2010, 12, 8902–8913 RSC.
- Z. Ajoyan, G. A. Mandl, P. R. Donnarumma, V. Quezada-Novoa, H. A. Bicalho, H. M. Titi, J. A. Capobianco and A. J. Howarth, ACS Mater. Lett., 2022, 4, 1025–1031 CrossRef CAS.
- W. Zhu, H. Yu, X. Zhu and H. Li, Inorg. Chem. Commun., 2022, 136, 109182 CrossRef CAS.
- X. Liu, R. Li, X. Xu, Y. Jiang, W. Zhu, Y. Yao, F. Li, X. Tao, S. Liu, W. Huang and Q. Zhao, Adv. Mater., 2023, 35, 2206741 CrossRef CAS PubMed.
- X. Wang, Y. Wang, Y. Wang, H. Liu, Y. Zhang, W. Liu, X. Wang and S. Wang, Chem. Commun., 2020, 56, 233–236 RSC.
- A. Topor, D. Avram, R. Dascalu, C. Maxim, C. Tiseanu and M. Andruh, Dalton Trans., 2021, 50, 9881–9890 RSC.
- Q. Gu, W. Wang, H. Lu, X. Chen, S. Wang and S. Wu, Dalton Trans., 2022, 51, 257–263 RSC.
- J. Gao, J. Lu, B. Li, W. Wang, M. Xie, S. Wang, F. Zheng and G. Guo, Chin. Chem. Lett., 2022, 33, 5132–5136 CrossRef CAS.
- M. J. Neufeld, H. Winter, M. R. Landry, A. M. Goforth, S. Khan, G. Pratx and C. Sun, ACS Appl. Mater. Interfaces, 2020, 12, 26943–26954 CrossRef CAS PubMed.
|
This journal is © The Royal Society of Chemistry 2023 |
Click here to see how this site uses Cookies. View our privacy policy here.