DOI:
10.1039/D2CE01393B
(Paper)
CrystEngComm, 2023,
25, 130-136
Cu(II) 3,5-diiodosalicylate complexes: precursor-dependent formation of mono-, di-, tri- and tetranuclear compounds and 1D coordination polymers†
Received
10th October 2022
, Accepted 22nd November 2022
First published on 22nd November 2022
Abstract
Reactions of CuCl2·2H2O or Cu(NO3)2·6H2O with 3,5-diiodosalicylic acid (DISA) in the presence of different amines result in structurally diverse products. Overall, we isolated 14 complexes of various nuclearity (1, 2, 3 and 4, as well as 1D coordination polymers), all characterized by X-ray diffraction. Magnetic properties were studied for several representatives of this class.
Introduction
It will not be an exaggeration to state that carboxylate complexes constitute one of the most studied classes of coordination compounds.1–5 Currently, the number of corresponding structures deposited to the Cambridge Structural Database (CSD) exceeds 14
000, and these are extremely diverse, representing dozens of different structural motifs.6–12
Within our recent research, we focused on halogen-substituted arenecarboxylates as ligands. On the one hand, these are not uncommon – there are dozens of corresponding complexes. As a single random example, there are 60 (!) compounds with 4-iodobenzoic acid (M = Sn,13,14 U,15–17 Cu,18–20 Ru,21 Mo,22 Mn,23,24 lanthanides,25,26etc.). However, despite 1) the growing interest on halogen bonding (XB), a specific kind of non-covalent interaction involving halogen atoms,27–29 and 2) the fact that it can be assumed that such coordination units can be involved in XB formation, these are however rather rarely considered from this point of view. This idea was successfully exploited, e.g., by Brammer et al.,30–32 but the number of such studies is however rather low.16,22
Observing the range of potential ligands attractive within the abovementioned context, we noticed that the commercially available 3,5-diiodosalicylic acid (DISA), despite it being a rather widespread and affordable reagent, was rarely used for the design of carboxylate complexes. Filling this gap, we recently prepared a series of corresponding heteroleptic derivatives of Zn(II)33 – all belonging to the same type (mononuclear) but featuring different patterns of XB in the solid state. Turning to Cu(II) and using trialkylamines as deprotonating agents, we successfully isolated four mono- and dinuclear complexes, all featuring chelate coordination of fully deprotonated DISA.34 However, once we decided to involve different substituted pyridines which can play a dualistic role (both deprotonation of DISA and coordination to a metal center giving a heteroleptic complex), we found that the diversity of products increases dramatically. Depending on the Cu source (nitrate vs. chloride), reagent ratio and other conditions, mono-, bi-, tri- and tetranuclear discrete complexes as well as 1D coordination polymers can be formed. In this work, we summarize our research in this field, discussing the structural features and (where relevant) magnetic behavior of the complexes.
Experimental section
All reagents were obtained from commercial sources and used as purchased. Solvents were purified according to the standard procedures.
Synthesis of 1
44 mg (0.18 mmol) of Cu(NO3)2·3H2O were dissolved in 6 ml of CH3CN, followed by the addition of a solution of H2DISA (140 mg, 0.36 mmol) and Py (58 μl, 0.72 mmol) in 5 ml of CH3CN. Slow evaporation results in the formation of violet crystals of 1. Yield 87%. For C24H16CuI4N2O6 calcd, %: C, 28.84; H, 1.61; N, 2.80; found, %: C, 28.95; H, 1.71; N, 2.89.
Synthesis of 2
19.7 mg (0.12 mmol) of CuCl2·2H2O were dissolved in 4 ml of CH3CN, followed by the addition of a solution of H2DISA (90 mg, 0.23 mmol) and 2-MePy (45.5 μl, 0.46 mmol) in 5 ml of CH3CN. Violet crystals of 2 formed within 1 day after slow evaporation of the solvent. Yield 85%. For C26H20CuI4N2O6 calcd, %: C, 30.39; H, 1.96; N, 2.72; found, %: C, 30.51; H, 2.07; N, 2.78.
Synthesis of 3
The procedure was the same as that for 1, using 3-MePy (35 μl, 0.36 mmol) instead of pyridine. Slow evaporation led to the formation of purple plate-like crystals of 3.
Synthesis of 4
27.8 mg (0.12 mmol) of Cu(NO3)2·3H2O were dissolved in 4 ml of CH3CN, followed by the addition of a solution of H2DISA (90 mg, 0.23 mmol) and 2-MePy (22.8 μl, 0.23 mmol) in 4 ml of CH3CN. The next day, upon evaporation, green crystals of 4 suitable for XRD were formed, but the samples were always contaminated by an unidentified brown fine crystalline product.
Synthesis of 5
15.3 mg (0.09 mmol) of CuCl2·2H2O were dissolved in 5 ml of CH3CN, followed by the addition of a solution of H2DISA (70 mg, 0.18 mmol) and 3-MePy (35.0 μl, 0.36 mmol) in 5 ml of CH3CN. Upon slow evaporation, blue crystals of 5 formed the next day. Yield 79%. For C38H34Cl2Cu2I4N4O6 calcd, %: C, 33.88; H, 2.55; N, 4.16; found, %: C, 33.97; H, 2.65; N, 4.29.
Synthesis of 6
The procedure was the same as that for 5, using 3,4-MePy (40.4 μl, 0.36 mmol) instead of 3-MePy. Slow evaporation (approx. 3 days) led to the formation of blue crystals of 6. Yield 82%. For C42H42Cl2Cu2I4N4O6 calcd, %: C, 35.96; H, 3.02; N, 4.00; found, %: C, 36.08; H, 3.10; N, 4.11.
Synthesis of 7
The procedure was the same as that for 5, using 4-MePy (17.5 μl, 0.18 mmol) instead of 3-MePy. Immediately after mixing, a blue precipitate appeared, from which blue crystals of 7 formed after 9 days. Yield 73%. For C50H48Cl4Cu3I4N6O6 calcd, %: C, 36.04; H, 2.91; N, 5.05; found, %: C, 35.91; H, 3.00, N, 5.15.
Synthesis of 8
The procedure was the same as that for 5, using 4-EtPy (40.8 μl, 0.36 mmol) instead of 3-MePy. Upon slow evaporation, blue crystals of 8 formed within 1 day. Yield 81%. For Cu3Cl4C56I4O6H60N6 calcd, %: C, 38.42; H, 3.46; N, 4.80; found, %: 3, 38.30; H, 3.45; N, 4.87.
Synthesis of 9
7.7 mg (0.03 mmol) of Cu(NO3)2·3H2O were dissolved in 5 ml of CH3CN, followed by the addition of a solution of H2DISA (25 mg, 0.05 mmol) and Py (5.4 μl, 0.13 mmol) in 5 ml of CH3CN. Slow evaporation for several days led to the formation of dark green crystals of 9 with small grains of a lighter color of 4 (as follows from PXRD data).
Synthesis of 10
6.2 mg (0.03 mmol) of Cu(NO3)2·3H2O were dissolved in 5 ml of CH3CN, followed by the addition of a solution of H2DISA (20 mg, 0.05 mmol) and 3-MePy (5.0 μl, 0.13 mmol) in 5 ml of CH3CN. Small green crystals of 10 formed overnight in a closed vial. For C52H36Cu4I8N4O12 calcd, %: C, 28.69; H, 1.67; N, 2.58; found, %: C, 28.74; H, 1.76; N, 2.50.
Synthesis of 11
15.5 mg (0.06 mmol) of Cu(NO3)2·3H2O were dissolved in 5 ml of EtOH, followed by the addition of a solution of H2DISA (50 mg, 0.13 mmol) and 4-MePy (12.5 μl, 0.13 mmol) in 5 ml of EtOH. Small dark green crystals of 11 formed overnight in a closed vial. For C52H36Cu4I8N4O12 calcd, %: C, 28.69; H, 1.67; N, 2.58; found, %: C, 28.77; H, 1.79; N, 2.64.
Synthesis of 12
15.5 mg (0.06 mmol) of Cu(NO3)2·3H2O were dissolved in 5 ml of CH3CN, followed by the addition of a solution of H2DISA (50 mg, 0.13 mmol) and 4-EtPy (14.6 μl, 0.13 mmol) in 5 ml of CH3CN. Immediately after mixing, a green precipitate appeared, from which green crystals of 12 formed after 2 days. For C56H44Cu4I8N4O12 calcd, %: C, 30.11; H, 1.99; N, 2.51; found, %: C, 30.02; H, 2.06; N, 2.57.
Synthesis of 13
9.3 mg (0.04 mmol) of Cu(NO3)2·3H2O were dissolved in 4 ml of EtOH, followed by the addition of a solution of H2DISA (30 mg, 0.08 mmol) and 2-MePy (15.2 μl, 0.15 mmol) in 4 ml of EtOH. The next day, dark green crystals of 13 formed in a closed vial.
Synthesis of 14
9.3 mg (0.04 mmol) of Cu(NO3)2·3H2O were dissolved in 5 ml of CH3CN, followed by the addition of a solution of H2DISA (30 mg, 0.08 mmol) and 4-MePy (15 μl, 0.15 mmol) in 5 ml of CH3CN. Slow evaporation over a week led to the formation of small green crystals of 14.
X-ray diffraction
Crystallographic data and refinement details for 1–14 are given in Table S1 (ESI†). For 1–6, 8, and 10, the data were collected on a New Xcalibur (Agilent Technologies) diffractometer with MoKα radiation (λ = 0.71073) by doing φ scans of narrow (0.5°) frames at 150 K. Absorption correction was done empirically using SCALE3 ABSPACK (CrysAlisPro, Agilent Technologies, Version 1.171.37.35 (release 13-08-2014 CrysAlis171.NET) (compiled Aug 13 2014, 18:06:01)). For 7, 9, and 11–14, the data were collected on a Bruker D8 Venture diffractometer with a CMOS PHOTON III detector and IμS 3.0 source (Mo Kα radiation, λ = 0.71073 Å) at 150 K. The φ- and ω-scan techniques were employed. Absorption correction was applied using SADABS (Bruker Apex3 software suite: Apex3, SADABS-2016/2 and SAINT, version 2018.7-2; Bruker AXS Inc.: Madison, WI, 2017.). Structures were solved using SHELXT35 and refined by full-matrix least-squares treatment against |F|2 in anisotropic approximation with SHELX 2014/7 (ref. 36) in the ShelXle program.37 H-atoms were refined in geometrically calculated positions. Disordering of the coordinated Me-Py molecule over two closed positions in the crystal structure of 1 is a cause for refinement of all participating atoms in isotropic approximation. In the case of 2, 8, 10, 11 and 13, there is some residual electronic density around the I atoms without any chemical sense, reflecting A- and B-type alerts during PLATON check. In all cases, the residual electronic density does not exceed 10% over the total electronic count. The crystallographic data have been deposed in the Cambridge Crystallographic Data Centre under the deposition codes CCDC 2194777–2194790.
Powder X-ray diffraction (PXRD)
XRD analysis of polycrystals was performed on a Shimadzu XRD-7000 diffractometer (CuK-alpha radiation, Ni – filter, linear One Sight detector, 0.0143° 2θ step, 2 s per step). Plotting of the PXRD patterns and data treatment were performed using X'Pert Plus software (see the ESI†).
Magnetic measurements and TGA data
Details are given in the ESI.†
Results and discussion
The list of synthetic procedures given above represents only the optimized procedures for each compound. Behind them, there was an extended set of experiments which we summarized in Table 1. We performed them by varying several parameters: 1) the ratio of reagents, 2) the source of copper (nitrate vs. chloride) and 3) the pyridine ligand (in some cases, we also varied the solvent, as mentioned in Table 1). Interestingly, in the case of 2-MePy, no Cl-containing complex was isolated, regardless of the conditions. It remains unclear what is the driving force favoring the formation of bi- vs. trinuclear complexes (5 and 6vs.7 and 8) – it can be seen that complexes of only one of these types are formed with a certain Py ligand.
Table 1 Products of reactions between Cu(II) salts, H2DISA and different pyridines
|
L |
Cu salt |
Cu : H2DISA : L |
Products |
Comments |
1 |
Py |
Chloride |
1 : 2 : 2 |
1
|
Initially forms [Cu2PyCl2]. After filtering off, it crystallizes to pure 1 |
2 |
Py |
Chloride |
1 : 2 : 4 |
1
|
See above |
3 |
Py |
Nitrate |
1 : 2 : 2 |
9
|
With minor amounts of 4 |
4 |
Py |
Nitrate |
1 : 2 : 4 |
1
|
— |
5 |
2-MePy |
Chloride |
1 : 2 : 2 |
2
|
— |
6 |
2-MePy |
Chloride |
1 : 2 : 4 |
2
|
— |
7 |
2-MePy |
Nitrate |
1 : 2 : 2 |
4
|
Contaminated by unidentified by-products |
8 |
2-MePy |
Nitrate |
1 : 2 : 4 |
13
|
|
9 |
2-MePy |
Nitrate |
1 : 2 : 2 |
13
|
EtOH as solvent |
10 |
2-MePy |
Nitrate |
1 : 2 : 4 |
13
|
EtOH as solvent |
11 |
3-MePy |
Chloride |
1 : 2 : 2 |
5
|
— |
12 |
3-MePy |
Chloride |
1 : 2 : 4 |
5
|
— |
13 |
3-MePy |
Nitrate |
1 : 2 : 2 |
10
|
— |
14 |
3-MePy |
Nitrate |
1 : 2 : 4 |
3
|
See 7 |
15 |
4-MePy |
Chloride |
1 : 2 : 2 |
7
|
— |
16 |
4-MePy |
Chloride |
1 : 2 : 4 |
7
|
— |
17 |
4-MePy |
Nitrate |
1 : 2 : 2 |
11
|
— |
18 |
4-MePy |
Nitrate |
1 : 2 : 4 |
14
|
See 7 |
19 |
4-MePy |
Nitrate |
1 : 2 : 2 |
11
|
EtOH as solvent |
20 |
4-MePy |
Nitrate |
1 : 2 : 4 |
11
|
EtOH as solvent |
21 |
4-EtPy |
Chloride |
1 : 2 : 2 |
8
|
— |
22 |
4-EtPy |
Chloride |
1 : 2 : 4 |
8
|
— |
23 |
4-EtPy |
Nitrate |
1 : 2 : 2 |
12
|
— |
24 |
4-EtPy |
Nitrate |
1 : 2 : 4 |
12
|
— |
25 |
3,4-MePy |
Chloride |
1 : 2 : 2 |
6
|
— |
26 |
3,4-MePy |
Chloride |
1 : 2 : 4 |
6
|
— |
[Cu(HDISA)2L2]
There are three complexes of the [Cu(HDISA)2L2] type – with L = Py (1), 2-MePy (2) and 3-MePy (3), respectively. The Cu has a square planar coordination environment consisting of two DISA units with a protonated OH group and two pyridines, respectively, in trans-mode (Fig. 1). This structural type was observed earlier in Cu(II) non-substituted38–41 and substituted42–45 salicylates. One O atom of each COO− is involved in coordination, while the Cu⋯O(2) distances can rather indicate semi-coordination (Table 2). In 3, the 3-MePy ligands are disordered.
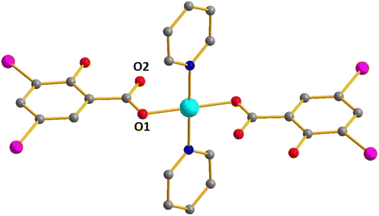 |
| Fig. 1 Structure of 1. Here and below: Cu cyan; N deep blue; I purple; O red; C grey; H atoms are omitted. | |
Table 2 Cu⋯O and Cu–N distances (Å) in 1–3
Compound |
Cu–O(1) |
Cu–O(2) |
Cu–N |
1
|
1.949 |
2.632 |
2.017 |
2
|
1.921–1.931 |
2.851–2.934 |
1.980–1.981 |
3
|
1.987 |
2.535 |
1.974–2.015 |
In 1, there are both I⋯O and I⋯I interactions (3.417 and 3.761 Å, respectively; Fig. 2) involving 5-I and 3-I substituents, respectively. Such contacts are absent in 2.
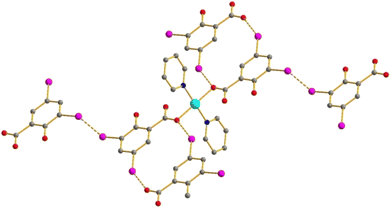 |
| Fig. 2 System of I⋯O and I⋯I contacts (dashed lines) in 1. | |
4 belongs to a very widespread family of paddlewheel-type complexes: the [Cu2(carboxylate)4L2] family.46–49 Four HDISA units (Cu–O = 1.966 Å) connect two Cu atoms into a binuclear core (Cu⋯Cu = 2.668 Å). The –OH groups of HDISA are disordered over two positions with equal occupancies. The terminal positions at Cu sites are occupied by CH3CN (Cu–N = 2.168 Å); also, there are solvate acetonitrile molecules in this structure.
[Cu2L4(HDISA)2Cl2]
These binuclear complexes form in two cases (L = 3-MePy (5) and 3,4-MePy (6)) where CuCl2 was used as the precursor (see above). The Cu atoms are connected by two μ2-bridging chlorides (see Table 3 for all bond lengths). The coordination sphere of each Cu is completed by two pyridine ligands and one HDISA coordinated by carboxylate groups (Fig. 3). This structural type is rare for copper(II) carboxylates; there are only two relevant examples50 (including those found by us in Cu 2-iodobenzoates51) with monodentate N-donor ligands and slightly more with bidentate ones.52–54 There are neither I⋯I nor I⋯O non-covalent interactions in these structures.
Table 3 Cu–O, Cu–N and Cu–Cl bond lengths (Å) in 5–8
Compound |
Cu–O |
Cu–N |
Cu–Cl |
5
|
2.324–2.637 |
1.998–2.010 |
2.025 |
6
|
2.337–2.641 |
1.994–2.006 |
2.026 |
7
|
2.292–2.829 |
1.998–2.031 |
2.019 |
8
|
2.297–2.957 |
1.985–2.011 |
2.026 |
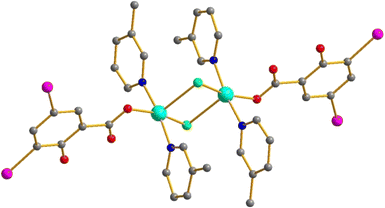 |
| Fig. 3 Structure of 5. Here and below: Cl light green. | |
[Cu3L6(HDISA)2Cl4]
Trinuclear complexes of this family (Fig. 4) can be represented as a derivative of the [Cu2L4(HDISA)2Cl2] type with a {CuL2Cl2} unit “inserted” into the middle of the molecule. This is, to the best of our knowledge, an unprecedented type in Cu carboxylates. We isolated two examples – with L = 4-MePy (7) and 4-EtPy (8). Interestingly, two of the Cu–μ2–Cl bonds are strongly elongated (2.879 and 2.957 Å in 7 and 8, respectively). In 8, there are weak (3.895 Å) I⋯I contacts between the neighboring molecules.
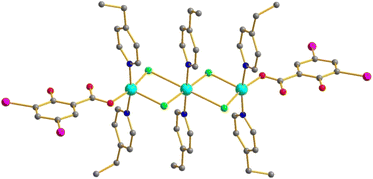 |
| Fig. 4 Structure of 8. | |
[Cu4L4(DISA)4]
These neutral complexes were isolated, with L = Py (9), 3-MePy (10), 4-MePy (11) and 4-EtPy (12). These consist of four copper atoms which build a tetrahedron. The DISA molecules are fully deprotonated; both carboxylate and hydroxo O-atoms are involved in coordination so that each DISA acts as a linker for two neighboring Cu centers. The coordination environment of the latter is therefore distorted square planar. Selection of a representative view of these structures is rather challenging so only the essential units are shown in Fig. 5. A summary of bond lengths and distances in 9–12 is given in Table 4. This motif is rare in Cu carboxylates – similar patterns were earlier encountered only in heteroleptic lactate complexes.55
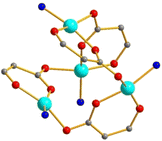 |
| Fig. 5 Structure of 9. Only N atoms of the Py ligands and only O and some C atoms of the DISA ligand are shown. | |
Table 4 Cu–O and Cu–N distances in 9–14
Compound |
Cu–O (2-O) |
Cu–O (carboxylate) |
Cu–N |
9
|
1.889 |
1.908–1.959 |
2.000 |
10
|
1.884 |
1.909–1.968 |
2.007 |
11
|
1.890 |
1.907–1.954 |
1.986 |
12
|
1.890 |
1.911–1.954 |
1.985 |
13
|
1.863 |
1.936–1.958 |
2.008 |
14
|
1.885 |
1.906–2.003 |
1.988 |
{[CuL(DISA)]}n
In the 1D coordination polymers of this type, the DISA units feature the same coordination mode as those in [Cu4L4(DISA)4] (all O atoms are involved, Fig. 6). Actually, this structure can be considered as a polymeric isomer of the previously considered tetranuclear type. The bond lengths in 13 and 14 (L = 2-MePy or 4-MePy, respectively) are listed in Table 4.
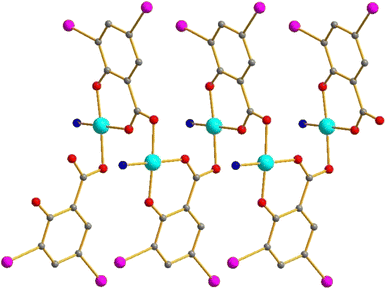 |
| Fig. 6 Structure of 14. Only N atoms of L are shown. | |
Magnetic properties
We selected several complexes – 1, 2, 5, 8, 11 and 13 – for further in-depth investigation of magnetic properties. For complexes 1 and 2, the μeff values at 300 K are 1.89 and 1.82μB, respectively, and change slightly with decreasing temperature. The 1/χ(T) dependencies obey the Curie–Weiss law in the temperature range 50–300 K with the best fit values of C and Θ equal to 0.444 ± 0.001 K cm3 mol−1 and 1.2 ± 0.1 K for 1 and 0.413 ± 0.001 K cm3 mol−1 and −0.65 ± 0.07 K for 2. The μeff values at 300 K and Curie constants are in good agreement with the theoretical spin-only values of 1.86μB and 0.433 K cm3 mol−1 for one Cu(II) ion (S = 1/2, g = 2.15). The Cu(II) ions in complexes 1 and 2 are magnetically isolated in full accordance with X-ray data (Fig. 7). Exchange interactions between spins of Cu(II) ions are negligibly small, and weakly ferromagnetic in the case of 1 (Weiss constant is 1.2 K) and weakly antiferromagnetic in case of complex 2 (Weiss constant is −0.65 K). Temperature dependencies of the effective magnetic moment (μeff) and inverse magnetic susceptibility 1/χ for complexes 1 and 2 are shown in the ESI.†
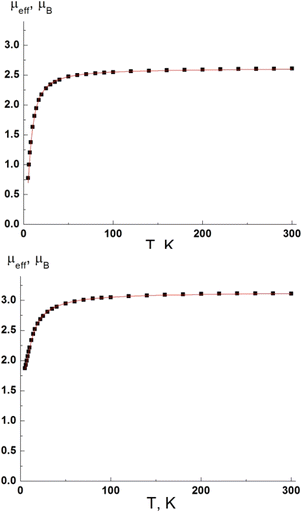 |
| Fig. 7 Temperature dependencies of μeff for the complexes 5 (top) and 8 (bottom). Solid lines are theoretical curves. | |
The μeff values at 300 K for complexes 5 and 8 are 2.61 and 3.11μB, respectively, and change slightly with decreasing temperature down to 100 K. Below 100 K, the μeff decreases and reaches 0.78μB and 1.87μB at 5 K for 5 and 8, respectively. The μeff values at 300 K are in good agreement with the theoretical spin-only values of 2.63μB and 3.23μB for two and three Cu(II) ions in the case of 5 and 8 respectively. Temperature dependencies of μeff are indicative of antiferromagnetic coupling between the spins of Cu(II) ions in 5 and 8. The dimer model (Spin Hamiltonian H = −2JS1S2) was used to analyze the experimental μeff(T) dependence of 5, because two Cu(II) ions are connected by two μ2-bridging chlorides. In complex 8, three Cu(II) ions are connected by four μ2-bridging chlorides, so the trimer model (Spin Hamiltonian H = −2J(S1S2+ S2S3)) was used. The best fit values of the g-factor and exchange coupling parameter J are 2.14 ± 0.01 and −6.9 ± 0.1 cm−1 for complex 5 and 2.10 ± 0.01 and −5.8 ± 0.1 cm−1 for complex 8.
The μeff value for 11 at 300 K is 3.81μB and is in good agreement with the theoretical one of 3.72μB for four Cu(II) ions. The μeff increases with decreasing temperature and reaches 4.94μB at 6 K. A tetramer model (Spin Hamiltonian H = −2J(S1S2 + S2S3 + S3S4 + S1S4)) describes well the experimental μeff(T) dependence with the best fit values of g-factor and exchange coupling parameter J equal to 2.15 ± 0.01 and 7.6 ± 0.1 cm−1. Intermolecular exchange interactions are very weak, zJ′’ = −0.05 ± 0.01 cm−1, but should be taken into account to describe the μeff(T) dependence properly at low temperatures.
For the 1D coordination polymer complex 13, the μeff value at 300 K is 1.81μB and changes slightly with decreasing temperature down to 100 K. Below 100 K, the μeff increases and reaches 2.47μB at 5 K, which indicates the predomination of ferromagnetic exchange interactions between spins of Cu(II) ions in 13. Analysis of the experimental μeff(T) dependence was performed using the uniform chain model (Spin Hamiltonian H = −2J ∑SiSi+1) in the finite chain approximations. The best fit values of g-factor and exchange coupling parameter J are 2.05 ± 0.01 and 5.1 ± 0.2 cm−1.
Overall, the magnetic structures of all investigated complexes are correlated with the structural motifs. Weak ferromagnetic exchange interactions dominate in 11 and 13, whereas in complexes 5 and 8, with μ2-bridging chlorides between Cu(II) ions, exchange interactions are antiferromagnetic in character.
Additionally, we performed TGA for the complexes isolated as pure phases. These data are summarized in the ESI.†
Conclusions
We demonstrated that 3,5-diiodosalicylate and Cu(II) in the presence of auxiliary ligands can undergo self-assembly into complexes of different nuclearity and geometries, including also 1D coordination polymers. Additionally, there often appear systems of halogen bonds in the solid state which, however, do not have a direct influence on the magnetic properties (these are governed by the molecular structures).
Conflicts of interest
There are no conflicts to declare.
Acknowledgements
This research was funded by the Russian Foundation for Basic Research, Grant No. 20-33-70010, and partially supported by the Ministry of Science and Higher Education of the Russian Federation (structural characterization of the samples, 121031700313-8).
Notes and references
- B.-H. Ye, M.-L. Tong and X.-M. Chen, Coord. Chem. Rev., 2005, 249, 545–565 CrossRef CAS.
- T. Loiseau, I. Mihalcea, N. Henry and C. Volkringer, Coord. Chem. Rev., 2014, 266–267, 69–109 CrossRef CAS.
- Y.-Z. Zheng, Z. Zheng and X.-M. Chen, Coord. Chem. Rev., 2014, 258–259, 1–15 CrossRef CAS.
- Y. A. Belousov, A. A. Drozdov, I. V. Taydakov, F. Marchetti, R. Pettinari and C. Pettinari, Coord. Chem. Rev., 2021, 445, 214084 CrossRef CAS.
- M. A. Kiskin and I. L. Eremenko, Russ. Chem. Rev., 2006, 75, 559–575 CrossRef CAS.
- I. A. Lutsenko, M. A. Kiskin, S. A. Nikolaevskii, A. A. Starikova, N. N. Efimov, A. V. Khoroshilov, A. S. Bogomyakov, I. V. Ananyev, J. K. Voronina, A. S. Goloveshkin, A. A. Sidorov and I. L. Eremenko, ChemistrySelect, 2019, 4, 14261–14270 CrossRef CAS.
- S. A. Nikolaevskii, D. S. Yambulatov, A. A. Starikova, A. A. Sidorov, M. A. Kiskin and I. L. Eremenko, Russ. J. Coord. Chem., 2020, 46, 260–267 CrossRef CAS.
- N. V. Gogoleva, M. A. Shmelev, I. S. Evstifeev, S. A. Nikolaevskii, G. G. Aleksandrov, M. A. Kiskin, Z. V. Dobrokhotova, A. A. Sidorov and I. L. Eremenko, Russ. Chem. Bull., 2016, 65, 181–190 CrossRef CAS.
- K. A. Yashkova, S. N. Mel'nikov, S. A. Nikolaevskii, M. A. Shmelev, A. A. Sidorov, M. A. Kiskin and I. L. Eremenko, J. Struct. Chem., 2021, 62, 1378–1384 CrossRef CAS.
- G. N. Kuznetsova, S. A. Nikolaevskii, D. S. Yambulatov, M. A. Shmelev, M. A. Kiskin, A. G. Starikov, A. A. Sidorov and I. L. Eremenko, J. Struct. Chem., 2021, 62, 184–195 CrossRef CAS.
- D. S. Yambulatov, S. A. Nikolaevskii, M. A. Shmelev, K. A. Babeshkin, D. V. Korchagin, N. N. Efimov, A. S. Goloveshkin, P. A. Petrov, M. A. Kiskin, M. N. Sokolov, M. N. Sokolov and I. L. Eremenko, Mendeleev Commun., 2021, 31, 624–627 CrossRef CAS.
- E. S. Bazhina, M. A. Shmelev, A. A. Korlyukov, M. A. Kiskin and I. L. Eremenko, Russ. J. Coord. Chem., 2021, 47, 105–116 CrossRef CAS.
- X.-M. Zhu, Y.-L. Feng, F.-X. Zhang, J.-X. Yu, W.-J. Jiang, Y.-X. Tan, Z.-J. Zhang and D.-Z. Kuang, Chin. J. Inorg. Chem., 2015, 31, 761–766 CAS.
- J. Rani, G. Kaur, Diksha Sushila, R. Yadav, R. Kataria, P. Venugopalan, P. Natarajan, A. Chaudhary and R. Patra, CrystEngComm, 2019, 21, 1150–1158 RSC.
- N. P. Deifel and C. L. Cahill, Chem. Commun., 2011, 47, 6114–6116 RSC.
- J. A. Ridenour, M. H. Schofield and C. L. Cahill, Cryst. Growth Des., 2020, 20, 1311–1318 CrossRef CAS.
- M. Kalaj, K. P. Carter and C. L. Cahill, Eur. J. Inorg. Chem., 2017, 2017, 4702–4713 CrossRef CAS.
- K. Takahashi, N. Hoshino, T. Takeda, K. Satomi, Y. Suzuki, S.-I. Noro, T. Nakamura, J. Kawamata and T. Akutagawa, Dalton Trans., 2016, 45, 3398–3406 RSC.
- M. I. Mohamadin, N. Abdullah, K. M. Lo and S. W. Ng, Acta Crystallogr., Sect. E: Struct. Rep. Online, 2010, 66, m1530 CrossRef CAS PubMed.
- A. D. Burrows, M. F. Mahon, P. R. Raithby, A. J. Warren, S. J. Teat and J. E. Warren, CrystEngComm, 2012, 14, 3658–3666 RSC.
- Q. Guo, J. Dou, L. Wu, D. Li and D. Wang, J. Coord. Chem., 2007, 60, 785–794 CrossRef CAS.
- M. H. Chisholm, C. B. Durr, T. F. Spilker and P. J. Young, Inorg. Chim. Acta, 2015, 424, 300–307 CrossRef CAS.
- M. Hołyńska and S. Dehnen, Z. Anorg. Allg. Chem., 2012, 638, 763–769 CrossRef.
- M. Hołyńska and S. Dehnen, Inorg. Chem. Commun., 2011, 14, 1290–1293 CrossRef.
- J. H. S. K. Monteiro, A. De Bettencourt-Dias, I. O. Mazali and F. A. Sigoli, New J. Chem., 2015, 39, 1883–1891 RSC.
- J. August Ridenour, K. P. Carter and C. L. Cahill, CrystEngComm, 2017, 19, 1190–1203 RSC.
- G. R. Desiraju, P. S. Ho, L. Kloo, A. C. Legon, R. Marquardt, P. Metrangolo, P. Politzer, G. Resnati and K. Rissanen, Pure Appl. Chem., 2013, 85, 1711–1713 CrossRef CAS.
- P. Varadwaj, A. Varadwaj, H. Marques, P. R. Varadwaj, A. Varadwaj and H. M. Marques, Inorganics, 2019, 7, 40 CrossRef CAS.
- G. Cavallo, P. Metrangolo, R. Milani, T. Pilati, A. Priimagi, G. Resnati and G. Terraneo, Chem. Rev., 2016, 116, 2478–2601 CrossRef CAS PubMed.
- P. Smart, G. M. Espallargas and L. Brammer, CrystEngComm, 2008, 10, 1335–1344 RSC.
- P. Smart, Á. Bejarano-Villafuerte, R. M. Hendry and L. Brammer, CrystEngComm, 2013, 15, 3160 RSC.
- P. Smart, Á. Bejarano-Villafuerte and L. Brammer, CrystEngComm, 2013, 15, 3151–3159 RSC.
- M. A. Bondarenko, M. I. Rakhmanova, P. E. Plyusnin, P. A. Abramov, A. S. Novikov, K. Rajakumar, M. N. Sokolov and S. A. Adonin, Polyhedron, 2021, 194, 114895 CrossRef CAS.
- M. A. Bondarenko, A. S. Novikov, T. S. Sukhikh, I. V. Korolkov, M. N. Sokolov and S. A. Adonin, J. Mol. Struct., 2021, 1244, 130942 CrossRef CAS.
- G. M. Sheldrick, Acta Crystallogr., Sect. A: Found. Adv., 2015, 71, 3–8 CrossRef.
- G. M. Sheldrick, Acta Crystallogr., Sect. C: Struct. Chem., 2015, 71, 3–8 Search PubMed.
- C. B. Hübschle, G. M. Sheldrick and B. Dittrich, J. Appl. Crystallogr., 2011, 44, 1281–1284 CrossRef.
- A. L. Abuhijleh and C. Woods, Inorg. Chem. Commun., 2001, 4, 119–123 CrossRef CAS.
- L.-G. Zhu and S. Kitagawa, Z. fur Krist. – New Cryst. Struct., 2002, 217, 579–580 CAS.
- I. Leban, B. Kozlevčar, J. Sieler and P. Šegedin, Acta Crystallogr., Sect. C: Cryst. Struct. Commun., 1997, 53, 1420–1422 CrossRef.
- M. Devereux, D. O'Shea, M. O'Connor, H. Grehan, G. Connor, M. McCann, G. Rosair, F. Lyng, A. Kellett, M. Walsh, D. Egan and B. Thati, Polyhedron, 2007, 26, 4073–4084 CrossRef CAS.
- F. T. Greenaway, A. Pezeshk, A. W. Cordes, M. C. Noble and J. R. J. Sorenson, Inorg. Chim. Acta, 1984, 93, 67–71 CrossRef CAS.
- Z. Ma and B. Moulton, Mol. Pharmaceutics, 2007, 4, 373–385 CrossRef CAS PubMed.
- Y. Wang, X.-M. Lin, F.-Y. Bai, R. Zhang and X.-X. Zhang, Chin. J. Inorg. Chem., 2017, 33, 1667–1677 CAS.
- M. Puchoňová, J. Švorec, Ľ. Švorc, J. Pavlik, M. Mazúr, Ľ. Dlháň, Z. Růžičková, J. Moncoľ and D. Valigura, Inorg. Chim. Acta, 2017, 455, 298–306 CrossRef.
- D. S. Yambulatov, S. A. Nikolaevskii, I. A. Lutsenko, M. A. Kiskin, M. A. Shmelev, O. B. Bekker, N. N. Efimov, E. A. Ugolkova, V. V. Minin, A. A. Sidorov, A. A. Sidorov and I. L. Eremenko, Russ. J. Coord. Chem., 2020, 46, 772–778 CrossRef CAS.
- L. D. Popov, S. A. Borodkin, M. A. Kiskin, A. A. Pavlov, N. N. Efimov, E. A. Ugolkova, V. V. Minin and I. N. Shcherbakov, Russ. J. Coord. Chem., 2022, 48, 75–83 CrossRef CAS.
- I. A. Lutsenko, M. E. Nikiforova, K. A. Koshenskova, M. A. Kiskin, Y. V. Nelyubina, P. V. Primakov, M. V. Fedin, O. B. Becker, V. O. Shender, I. K. Malyants, I. K. Malyants and I. L. Eremenko, Russ. J. Coord. Chem., 2021, 47, 881–890 CrossRef CAS.
- M. A. Uvarova and S. E. Nefedov, Russ. J. Coord. Chem., 2021, 47, 399–408 CrossRef CAS.
- E. Dilek, S. Caglar, S. Çardak, B. Karakoç, B. Caglar and O. Sahin, Arch. Pharm., 2019, 352(6), 1900007 CrossRef.
- M. A. Bondarenko, A. S. Novikov, I. V. Korolkov, M. N. Sokolov and S. A. Adonin, Inorg. Chim. Acta, 2021, 524, 120436 CrossRef CAS.
- H. Jiang, J.-F. Ma and W.-L. Zhang, Acta Crystallogr., Sect. E: Struct. Rep. Online, 2007, 63, m1681 CrossRef CAS.
- C. J. Lin, Y. Q. Zheng, D. Y. Zhang and W. Xu, Russ. J. Coord. Chem., 2014, 40, 932–942 CrossRef CAS.
- G. H. Shahverdizadeh, S. W. Ng, E. R. T. Tiekink and B. Mirtamizdoust, Acta Crystallogr., Sect. E: Struct. Rep. Online, 2012, 68, m242–m243 CrossRef CAS PubMed.
- S. Balboa, J. Borrás, P. Brandi, R. Carballo, A. Castiñeiras, A. B. Lago, J. Niclós-Gutiérrez and J. A. Real, Cryst. Growth Des., 2011, 11, 4344–4352 CrossRef CAS.
|
This journal is © The Royal Society of Chemistry 2023 |