DOI:
10.1039/D2BM01416E
(Paper)
Biomater. Sci., 2023,
11, 170-180
Mimic enzymatic preparation of conductive supramolecular-polymeric hydrogels with antibacterial and antioxidant properties for accelerating wound healing†
Received
31st August 2022
, Accepted 11th November 2022
First published on 12th November 2022
Abstract
Supramolecular-polymeric hydrogels by combining low-molecular-weight gelators (LMWGs) with polymers have attracted great attention due to their unique double networks. Polymers are generally introduced into an LMWG matrix, thus enhancing the mechanical performance and broadening of the application fields of supramolecular hydrogels. Herein, a series of supramolecular-polymer hydrogels with inherent multiple properties were fabricated as wound dressings. An enzyme-like supramolecular H/G4 hydrogel co-assembled by hemin and guanosine-quartet motifs was successively integrated with hyaluronic acid (HA) and polyaniline (PANI), yielding a supramolecular-polymeric composite hydrogel (namely H/G4-HA(Cu)/PANI). The introduction of Cu2+-crosslinked hydrazide-grafted HA polymeric networks not only enhanced the viscoelasticity of the H/G4 supramolecular hydrogel but also endowed composite hydrogels with bioactive properties as wound healing dressings. The enzyme-like nanofibril H/G4 hydrogel could catalyse the oxidative polymerization of aniline, thus introducing PANI into gel networks. The porous H/G4-HA(Cu)/PANI exhibited a certain degree of swelling ratio under physiological conditions. H/G4-HA(Cu)/PANI also showed degradability, conductivity and appropriate mechanical properties. Through a full-thickness skin defect model of mice, this haemostatic, antioxidant, antibacterial and drug-free H/G4-HA(Cu)/PANI could accelerate wound healing processes by promoting wound closure, collagen deposition and upregulation of the CD31 expression level, which indicates that H/G4-HA(Cu)/PANI could be a promising wound dressing material.
1. Introduction
Supramolecular hydrogels usually formed via the self-assembly of low-molecular-weight gelators (LMWGs) have been widely used in various areas as functional materials due to their programmable structure–property relationship.1–5 However, traditional LMWG-based supramolecular hydrogels are mechanically weak or brittle, which has severely hindered their application scope. To date, great efforts have been devoted to develop composite hydrogels with enhanced mechanical performance.6,7 Among them, double network (DN) hydrogels have exhibited desirable mechanical properties due to the synergistic effects between the two independent but interpenetrating networks.8 One effective method is the introduction of polymer networks into the LMWG matrix as the second network.9–11 Agarose was firstly reported as a polymer network to combine amino acid-based supramolecular gelators, finally obtaining composite hydrogels.12 Other natural polymers were also used to fabricate supramolecular-polymeric composite hydrogels.13–16 Synthetic polymers17–19 were also used as polymeric networks to fabricate composite hydrogels. All these supramolecular-polymeric DN hydrogels exhibited improved mechanical properties.
Drugs or other active small molecules could co-assemble with LMWG yielding nanofibrous hydrogels, thus realizing controlled release of drugs or other functions.20–24 In our previous work, synthetic polymers were combined with DBSCOOH-diclofenac sodium co-assembled nanofibers via non-covalent or covalent interactions to fabricate mechanically enhanced DN supramolecular-polymeric composite hydrogels, thus achieving controlled release of diclofenac sodium and promoting wound healing.17 Recently, a naturally derived guanosine (G)-based supramolecular hydrogel (namely G4 hydrogel) was fabricated via the self-assembly of G and KB(OH)4, which involves cation-templated formation of G-quartet motifs and nanofibrils.25,26 Hemin could be incorporated into G-quartet networks via co-assembly, obtaining an enzyme-like nanofibrous hydrogel (namely H/G4 hydrogel), and polyaniline was further introduced into a H/G4 scaffold by in situ catalytic deposition in order to prepare conductive hybrid hydrogels as biosensors.27
To date, hydrogels have been applied in various fields.28–34 Among them, the extracellular matrix (ECM)-like property and excellent biocompatibility of hydrogels enable their outstanding application potential in biomedical fields.35–37 Hydrogels as wound dressings have been successfully used to promote tissue regeneration including skin wound repair.38–40 In comparison with traditional wound dressings, hydrogel-based ones could not only protect the damaged wound site from external pathogenic environments and moisturize the wound, but also regulate the wound healing phases, thus accelerating skin tissue remodelling.28,41–43 Infections and oxidative stress are the main obstacles of wound healing.44,45 Antibiotic-laden hydrogels have been developed to treat bacteria-infected wounds.46,47 However, some side effects such as drug resistance could not be ignored in clinical applications. There are a great number of alternative strategies by employing antimicrobial peptides,48 quaternized polymers49 or cationic polyelectrolytes50 to prepare composite hydrogels with inherent antibacterial properties. Meanwhile, other active gel ingredients were also integrated into gel networks to regulate the wound microenvironment, thus promoting wound healing phases.42,51–53
Moreover, supramolecular-polymeric composite hydrogels exhibited unique advantages due to their comprehensive properties of DN. There were various preparation strategies to fabricate supramolecular-polymeric composite hydrogels mainly by the preformation of polymeric networks or the post-polymerization of monomers.6,10,17,54,55 Supramolecular-polymeric composite hydrogels have also been widely applied in many fields, such as biosensors,27 electrolytes,41 delivery vehicles,56 cell culture scaffolds,57 bioinspired lubricants,58 biocatalysts,59 and antioxidant wound dressings.15 However, the incorporation of polymeric networks into composite hydrogels by using supramolecular networks as mimic enzymatic polymerization systems and the preparation of multifunctional supramolecular-polymeric hydrogel wound dressings with antioxidant, antibacterial and conductive properties for accelerating wound repair have not been reported.
Inspired by the above facts, we herein report an enzyme-like H/G4 hydrogel-based composite hydrogel by successively integrating hyaluronic acid (HA) and polyaniline (PANI) together, obtaining a multifunctional hydrogel wound dressing. HA as one of the major components of the ECM has been widely used to fabricate biomedical materials due to its excellent biocompatibility and degradability.60,61 Adipic acid dihydrazide (AH) was then grafted on HA backbones, obtaining the derivative AHHA, which could be crosslinked by copper ions (Cu2+) via a Cu2+-hydrazide coordination interaction62 to obtain a colloidal network (HA-Cu). The mechanical properties of the H/G4 hydrogel were then enhanced by HA-Cu, obtaining the supramolecular-polymeric composite hydrogel (namely H/G4-HA(Cu)). Cu2+ also exhibited pharmacological properties in wound treatment such as antibacterial properties, promoting collagen deposition and angiogenesis.40,63 PANI was finally introduced into the H/G4-HA(Cu) gel system by the addition of aniline and H2O2via mimic enzymatic polymerization of aniline (Fig. 1). Mild and efficient enzymatic polymerization or crosslinking has been widely used to prepare biomedical materials, which exhibits great superiority compared to the traditional radical polymerization induced by toxic initiators.64–67 Moreover, the excellent conductivity, redox activity and antibacterial ability of PANI enable its broad applications in many areas including electronic devices, sensors and tissue scaffolds.27,38,68 The final composite hydrogel exhibited inherent multifunctional effects including appropriate mechanical properties, biocompatibility, and antioxidant and bactericidal capabilities, and thus promoted wound healing in a full-thickness skin defect model of mice.
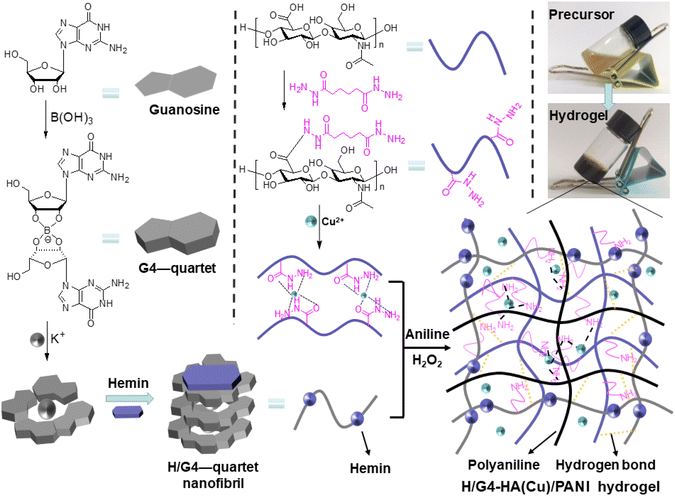 |
| Fig. 1 Schematic illustration of the mimic preparation of the inherent multifunctional supramolecular-polymeric H/G4-HA(Cu)/PANI hydrogel. | |
2. Experimental
2.1. Materials
Sodium hyaluronate (MW 310
000, HA) was purchased from Bloomage Freda Biopharm Co., Ltd. Adipic acid dihydrazide (AH), 1-ethyl-3-(3-(dimethylamino)propyl)carbodiimide hydrochloride (EDC·HCl), N-hydroxysuccinimide (NHS), guanosine, boric acid, hemin, 2-[4-(2-hydroxyethyl)piperazin-1-yl]ethanesulfonic acid (HEPES) and aniline were purchased from Shanghai Macklin Biochemical Co., Ltd. Solvents and other reagents were purchased from Chengdu Chron Chemicals Co., Ltd. All materials were used as received without further purification.
2.2. Synthesis of AH-grafted HA (AHHA)
Adipic acid dihydrazide (AH)-grafted HA was synthesized according to the literature with modifications.62 HA (2.0 g, 5.0 mmol disaccharide repeating units) was dissolved in 120 mL of deionized water, followed by addition of EDC·HCl (0.22 g) and NHS (0.15 g). Subsequently, AH (1.7 g) was added and the reaction proceeded for 24 h at room temperature. After exhaustive dialysis against water for 3 days, AHHA was obtained as a white floccule via lyophilization.
2.3. Preparation of hydrogels
The stock solution: AHHA (60 mg) was firstly dissolved in 3 mL of deionized water, and then guanosine (final concentration: 0.1 M), boric acid (final concentration: 0.05 M) and KOH (final concentration: 0.05 M) were added, followed by heating to 80 °C until a clear solution was obtained. Then, the resulting solution was allowed to cool to ca. 60 °C as the stock solution. Three hydrogels were finally prepared as follows. G4-HA(Cu) hydrogel: 0.5 mL of stock solution was mixed with 0.15 mL of CuSO4 (12.5 wt%) and kept at room temperature for 2 h. H/G4-HA(Cu) hydrogel: hemin (final concentration: 1 × 10–4 M) was firstly dissolved in 0.5 mL of stock solution, and 0.15 mL of CuSO4 (12.5 wt%) was then added and mixed thoroughly. After aging for 2 h at room temperature, the target hydrogel was fabricated. H/G4-HA(Cu)/PANI hydrogel: aniline (0.015 mL) was firstly dissolved in HEPES solution (10 mL, 10 mM, pH = 3), and then aniline solution was added to the above H/G4-HA(Cu) hydrogel in a vial. 2 h later, H2O2 (final concentration: 0.05 M) was added and the mixture was kept at room temperature for 1 h.
2.4. Characterization
The successful graft of AH groups on HA backbones was confirmed by 1H NMR (Bruker Avance III, 600 MHz), and the substitution degree was calculated according to the integral of related peaks. Fourier transform infrared (FTIR) spectra were measured using an FTIR spectrometer (Spectrum 400F, PerkinElmer) with a wavenumber range of 4000–550 cm−1. The morphology of lyophilized hydrogels was characterized by field emission scanning electron microscopy (FESEM) using SU8000 as the FESEM microscope (Hitachi) with a thin layer of platinum sputter-coating. The compressive tests were carried out using an HZ-1004 material test system (Dongguan Lixian Instrument Scientific Co., Ltd) with a crosshead rate of 5 mm min−1 at room temperature, by using cylindrical hydrogels (diameter: 0.4 cm, height: 0.9 cm). The rheological properties were analyzed using a MCR302 rheometer (Anton Paar) with a gap of 0.3 mm and a parallel plate of 25 mm. A fixed strain of 1% and a fixed frequency sweep of 1 rad s−1 were applied in the frequency sweep tests and the amplitude sweep test, respectively. The self-healing test was performed with the strain varying from 1% to 1000%. The haemostatic capability was tested by employing a mouse-tail amputation model. The resistance of hydrogels was measured by using a digital 4-probe tester with a current of 1 mA and a linear probe head (1.0 mm space).
2.5. Swelling ratio and mass change
The wet hydrogels (initial weight: W0) were placed into 30 mL of PBS (0.01 M, pH 7.4) in sealed vials at 37 °C. Upon reaching the pre-set time interval, the hydrogels were taken out from the solution and weighed (Wt, swelling weight). The gel mass was recorded until complete degradation. The mass change (%) was calculated using the following formula: (Wt − W0)/W0 × 100%. The mass change when gel mass reached the equilibrated state was defined as the swelling ratio.
2.6. Adhesive strength
Adhesive properties were evaluated by a lap-shear test.49 200 μL of the hydrogel precursor solution was applied onto the surface of porcine skin (40 mm × 20 mm), and another tissue slice was placed into the solution with an adhesive area of 20 mm × 20 mm. After incubation at room temperature for 2 h, the adhesive strength was measured by a lap-shear test using an HZ-1004 material test system (Dongguan Lixian Instrument Scientific Co., Ltd).
2.7. Antioxidant efficiency
The antioxidant efficiency of the H/G4-HA(Cu)/PANI hydrogel was evaluated by scavenging the ABTS+ free radicals.43 ABTS+ radicals were firstly prepared by mixing 2 mM ABTS with 2.47 mM K2S2O8 in PBS and reacted in the dark for 4 h at room temperature. After diluting (10 times) with PBS to an appropriate concentration, 4 mL of a diluent was mixed thoroughly with the hydrogel samples (20 mg, 40 mg, 80 mg, 160 mg and 320 mg). The diluent ABTS+ radical solution was defined as blank. 30 min later, UV-vis absorption at 734 nm was recorded to calculate the ABTS+ radical scavenging ratio.
2.8. Cytocompatibility evaluation
Hydrogel extract solution was used to evaluate the cytotoxicity of hydrogels. Hydrogels were firstly immersed in a fresh culture medium (25 mg mL−1) for 12 h at 37 °C, and the mixture was centrifuged, obtaining the supernatant as the extract solution. NIH-3T3 cells were then seeded in a 96-well plate at a density of 4000 cells per well. Typically, 5 × 103 cells per well were seeded in a 96-well plate and incubated overnight at 37 °C. After adhering to the plate, the culture medium was replaced with the hydrogel extract solution. 24 h later, the cell viability was evaluated by the CCK-8 (Invigentech) assay and Live/Dead® viability/cytotoxicity kit assay (Shanghai BestBio) using a fluorescence microscope (FV1200, Olympus).
2.9. Haemolytic assessment
The erythrocytes from pig blood were suspended in PBS as the stock solution (1%). Then, the hydrogels (100 μL) were mixed with 3 mL of the erythrocyte stock solution and shaken in an incubator at 37 °C for 30 min. After the hydrogel samples were removed, the suspension was centrifuged at 10
000 rpm for 10 min. The absorbance of the supernatant was recorded at 540 nm using a Cary 100 spectrometer (Agilent). The haemolysis percentage was calculated by employing PBS as the negative control and Triton X-100 as the positive control.
2.10. Antibacterial capacity
Staphylococcus aureus (S. aureus) and Escherichia coli (E. coli) were employed to test the surface antibacterial activity62 and the inhibition zone.44 For the surface antibacterial activity: hydrogels (0.65 mL) were firstly fabricated in a 48-well plate. Then, 100 μL of bacterial suspension (106 CFU per mL) was added onto the surface of the hydrogels and cultured at 37 °C for 18 h under a relatively humidified atmosphere. 100 μL of diluted (105 times) bacterial resuspension was added onto an agar plate. After culturing at 37 °C for 18 h, the colony-forming units (CFU) on the Petri dish were counted. For the inhibition zone: 100 μL of the bacterial suspension (106 CFU per mL) was seeded onto an agar plate, and then filter paper pieces (0.5 cm in diameter) after immersing in a gel precursor were placed and exposed to the bacteria on the agar plates. After culturing at 37 °C for 48 h, the inhibition zones were recorded.
2.11.
In vivo wound healing
Female Kunming rats (5–6 weeks) were employed in the in vivo wound healing experiments. Rats were randomly divided into three groups—the blank group, G4-HA group and H/G4-HA(Cu)/PANI group. G4-HA was prepared according to the preparation method of G4-HA(Cu) without the addition of Cu2+. Then, the mice were anesthetized by intraperitoneal injection of chloral hydrate and shaved on the back. Full-thickness skin round wounds of ca. 8 mm were created on the back. The wounds in the control group were covered with PBS (100 μL per rat), and the wounds in the other two groups were covered with G4-HA (100 μL per rat) and H/G4-HA(Cu)/PANI (100 μL per rat), respectively. Hydrogels were applied on day 7 for the second time. The wound area was recorded (day 0, day 3, day 7 and day 14). On day 14, all the rats were executed and the wound area tissues were collected for further evaluation. All experiments involving animals were ethically and scientifically approved by Henan Normal University and complied with the Practice for Laboratory Animals in China.
2.12. Histological evaluation
The collected tissues were fixed in 4% paraformaldehyde for 24 h, and then embedded in paraffin and cross-sectioned to 4 μm thickness slices, and finally stained with haematoxylin and eosin (Solarbio). All slices were analysed and imaged using a microscope (DS-U3, Nikon). Masson's trichrome staining was employed to evaluate the collagen deposition in the wounds. The regenerated skins from the wound site were also collected for immunofluorescence staining. The fixed and frozen sections were stained with CD31 (Proteintech). The nuclei were stained with 4,6-diamidino-2-phenylindole (DAPI, Solarbio) containing the mounting solution. Slides were observed using a fluorescence microscope (DS-U3, Nikon). All operations were followed according to the manufacturer's instructions.
2.13. Statistical analysis
All data were expressed as mean ± standard deviation. Statistical differences (*p < 0.05, **p < 0.01, and ***p < 0.001) were determined by a two-tailed t-test using Microsoft Excel.
3. Results and discussion
3.1. Preparation of hydrogels
The structure of AHHA was firstly confirmed by 1H NMR (Fig. S1†). The substitution degree (0.25) of AH groups was calculated according to the integral ratio of the methylene group in AH and the methyl group in HA. Guanosine and hemin-guanosine supramolecular hydrogels (G4 and H/G4, respectively) were firstly fabricated according to the literature. G4-HA hydrogel was prepared according to the preparation method of G4-HA(Cu) without the addition of Cu2+. However, they were too fragile to conduct any performance evaluation on them. One effective method is combining low molecular weight gelators (LMWGs) with cross-linked polymers. We chose HA, a major component of the extracellular matrix, to adjust the fragile properties. A Cu2+-crosslinked AHHA hydrogel was finally combined with the G4 or H/G4 hydrogel to fabricate the target supramolecular-polymeric hydrogels. The hydrogels could be fabricated in a one-pot method (Fig. 1). The introduction of Cu2+ will endow the hydrogels with antibacterial properties, and accelerate wound healing properties.63 The FTIR spectra verified the generation of the quinone structure in PANI (1603 cm−1, Fig. 2a). FESEM exhibited 3D porous networks for all these hydrogels (Fig. 2b–d), which could be beneficial for nutrient transport. The further swelling ratio tests showed that all hydrogels could adsorb water to a certain extent (Fig. 2e), which could endow the hydrogels with tissue extrude absorption ability. The hydrogels gradually decreased completely after immersing in PBS. Both swelling and degradation properties are desirable for their in vivo applications.
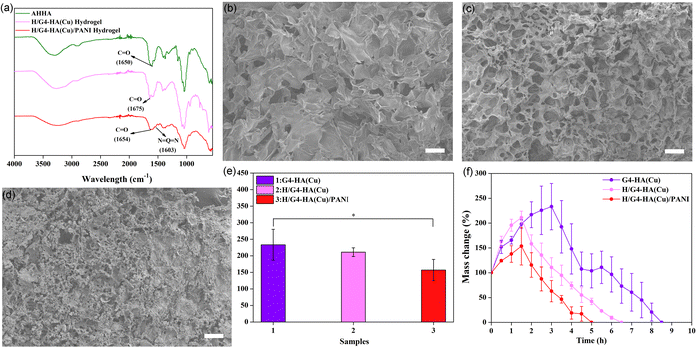 |
| Fig. 2 (a) The FTIR spectra of AHHA, H/G4-HA(Cu) and H/G4-HA(Cu)/PANI. (b–d) FESEM images for G4-HA(Cu), H/G4-HA(Cu) and H/G4-HA(Cu)/PANI, respectively. Scale bar: 100 μm. (e) Swelling ratio of G4-HA(Cu), H/G4-HA(Cu) and H/G4-HA(Cu)/PANI. (f) Mass change ratio of G4-HA(Cu), H/G4-HA(Cu) and H/G4-HA(Cu)/PANI. *p < 0.05. | |
3.2. Mechanical properties
Desirable mechanical properties are imperative for hydrogels used as wound dressing materials. All hydrogels could endure a certain degree of external compression with the compressive moduli of 68.24 kPa, 70.58 kPa and 62.15 kPa for G4-HA(Cu), H/G4-HA(Cu) and H/G4-HA(Cu)/PANI, respectively (Fig. 3a and b). The slight decrease of the modulus for H/G4-HA(Cu)/PANI might be ascribed to the immersing procedure in aniline solution. The rheological properties were further evaluated to obtain the viscoelasticity of hydrogels. As shown in Fig. 3c, the storage modulus (G′) overpassed the loss modulus (G′′) for all hydrogels, indicating their gelatinous properties. The storage modulus value was consistent with the corresponding compressive modulus for each hydrogel. Self-healing performance is another desirable property due to the irregular deformation, abrasion and even damage when bearing external force. Alternate step strain (1%–1000%) tests (Fig. 3d–f) were employed to evaluate the self-healing properties. The large strain was determined according to the strain sweep test (Fig. 3g). All hydrogels could maintain their colloidal properties (G′ > G′′) when a low strain was applied, while hydrogels’ networks were damaged under high strain (G′′ > G′). It is evident that the G′ value returned fast to their initial state after withdrawing the high strain, indicating the excellent self-healing properties, which might be due to multiple non-covalent interactions among the gel networks. Moreover, as an ideal wound dressing, the hydrogel could firmly adhere to the wound site, which is beneficial for preventing bacterial cloning and adsorbing extrude. The adhesive strength of all hydrogels was thus evaluated by a lap-shear test (Fig. 3h and i). Two pieces of porcine skin could be adhered tightly, and the H/G4-HA(Cu)/PNAI hydrogel exhibited the largest adhesive strength (2.82 kPa) compared to the other two groups (2.18 kPa for G4-HA(Cu) and 2.24 kPa for H/G4-HA(Cu)). The reason might be the contribution of the PANI network.
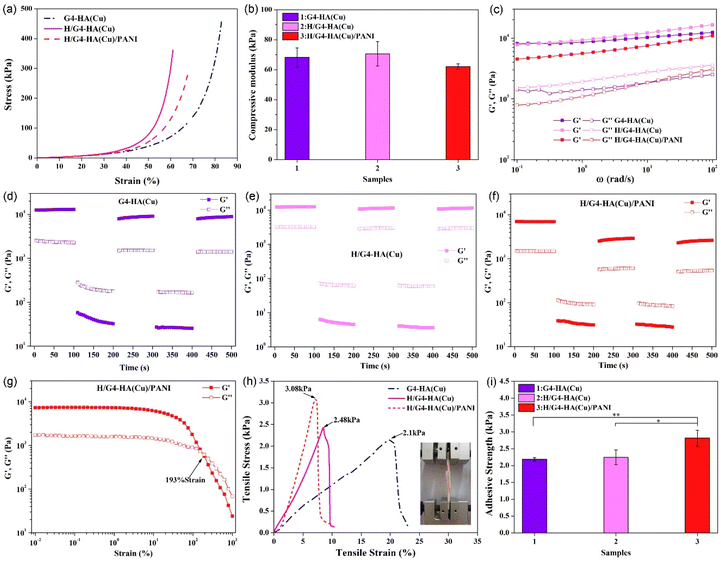 |
| Fig. 3 (a) Compressive strain–stress curves and (b) the compressive moduli of G4-HA(Cu), H/G4-HA(Cu) and H/G4-HA(Cu)/PANI. (c) Frequency sweep tests at a fixed strain (1%). (d–f) The alternate step strain sweep tests for G4-HA(Cu), H/G4-HA(Cu) and H/G4-HA(Cu)/PANI, respectively. (g) The strain amplitude sweep test of H/G4-HA(Cu)/PANI. (h) Tensile strain–stress curves and (i) the adhesive strength of G4-HA(Cu), H/G4-HA(Cu) and H/G4-HA(Cu)/PANI. *p < 0.05 and **p < 0.01. | |
3.3. Evaluation of haemostasis, antioxidant properties, cytocompatibility, haemolysis and conductivity
Haemostasis is the first phase of the entire wound healing process. Besides adhesiveness, haemostatic ability is beneficial for the following wound healing phases. The haemostatic capability was tested by employing a mouse-tail amputation model (Fig. 4a and b). The blood loss of the blank group (807.1 mg) was significantly higher than that of the groups using hydrogels, indicating their potential as haemostatic materials. Moreover, a large reactive species generated in the wound site could delay the healing processes. The antioxidant properties of wound dressings could relieve oxidative stress and ease inflammation.45 Borate esters as one of the typical ROS-responsive bonds would give hydrogels with good antioxidant properties.61 Polyaniline discovered in the mid-19th century has been widely used in various areas including electronic devices, sensors and tissue scaffolds due to its excellent conductivity, redox activity and antibacterial ability.69 The ABTS assay was employed to evaluate the free radical scavenging capability of the H/G4-HA(Cu)/PANI hydrogel. A concentration-dependent scavenging ratio could be observed, and over 80% radicals were scavenged at a 40 mg mL−1 gel concentration (Fig. 4c). The cell viability was further evaluated by using a hydrogel extract solution. Almost all the NIH-3T3 cells exhibited a spindle shape with green fluorescence (live/dead staining) for all hydrogels (Fig. 4d–f and Fig. S2a–c†). The cell viability for both hydrogels was at the same level as the blank group (Fig. 4g), showing good cytocompatibility. Good blood compatibility is also one of the application prerequisites for biomaterials. PBS and Triton were employed as the negative and positive controls, respectively (Fig. 4h). A bright colour could be observed for the Triton group, whereas the other groups exhibited a slight light-yellow colour. The quantitative results exhibited a low haemolysis ratio (less than 5%) for all hydrogel groups. In addition, conductive materials could electrically promote cell proliferation, differentiation and tissue regeneration. The Nyquist curve is shown in Fig. 4i; the radius of the quasi-semicircle for G4-HA was evidently larger than that of the quasi-semicircle for H/G4-HA(Cu)/PANI, which indicated that H/G4-HA(Cu)/PANI exhibited better electrical performance in comparison with G4-HA. The larger the radius, the larger the charge transfer resistance.70 The introduction of Cu2+ and PANI enhanced the conductivity of hydrogels.
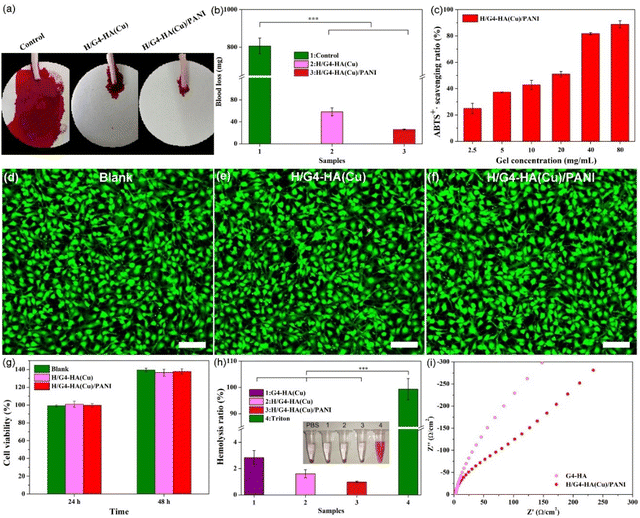 |
| Fig. 4
In vivo mouse-tail amputation model: (a) amputation images and (b) blood loss. (c) The antioxidant performance of H/G4-HA(Cu)/PANI by scavenging ABTS+ radicals. (d–f) Live/dead staining of NIH-3T3 cells after culturing with hydrogel extraction for 48 h. Scale bar: 100 μm. (g) Cell viability. (h) Haemolytic assessment of hydrogels. (i) The representative Nyquist plots of G4-HA and H/G4-HA(Cu)/PANI. ***p < 0.001. | |
3.4. Antibacterial performance
Besides adhesiveness to the wound site acting as a barrier, hydrogel wound dressings possessing inherent antibacterial properties would be desirable in clinical applications. Escherichia coli (E. coli, Gram-negative) and Staphylococcus aureus (S. aureus, Gram-positive) were employed to test the surface antibacterial activity and the inhibition zone. In Fig. 5a and b and Fig. S3,† it can be observed that after contacting with the bacteria, all hydrogels containing Cu2+ exhibited excellent bactericidal capability and killed almost all the E. coli and S. aureus, while the G4-HA hydrogel could only kill a certain number of bacteria due to the relatively weak antibacterial properties of the hydrazide groups. All hydrogels containing Cu2+ also exhibited evident inhibition zones on the agar plates of both E. coli and S. aureus after culturing for 48 h compared to the G4-HA hydrogel (Fig. 5c and Fig. S3†). The largest inhibition zone was observed for H/G4-HA(Cu)/PANI, which might be ascribed to the synergetic antibacterial capability of Cu2+, PANI, and hydrazide groups, especially the release of Cu2+ to the surroundings.63 All the above results indicated the potential of H/G4-HA(Cu)/PANI as promising wound dressing materials.
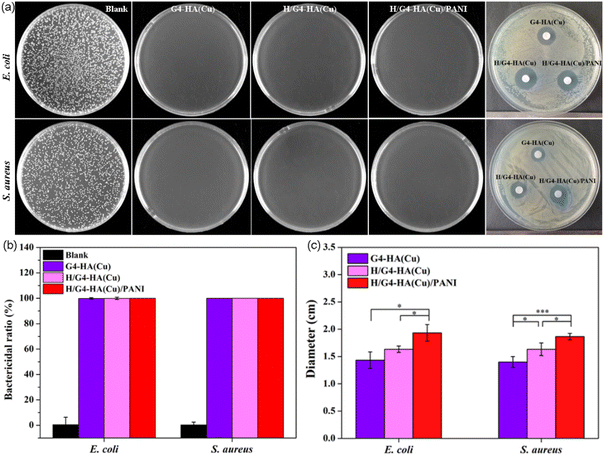 |
| Fig. 5 (a) Images of the bacterial clones that survived and the inhibition zone after coming in contact with the hydrogels. Quantification data of the bactericidal ratio (b) and inhibition zone (c) after treatment with different hydrogels. *p < 0.05 and ***p < 0.001. | |
3.5.
In vivo wound healing evaluation
The wound healing performance of the H/G4-HA(Cu)/PANI hydrogel was further evaluated by a full-thickness skin defect model. PBS was used as the blank group, and G4-HA, without the addition of Cu2+, was applied as the gel control group. In Fig. 6a and b, the wound contraction of the blank, G4-HA and H/G4-HA(Cu)/PANI groups recorded on the 3rd day, 7th day and 14th day, respectively, are shown. On day 3, all groups exhibited a certain degree of wound area reduction, while the H/G4-HA(Cu)/PANI group showed the largest wound closure ratio (37.5%). Both hydrogel groups exhibited a better therapeutic effect than the blank group. On day 7, the same healing trend was observed for all groups as on day 3. The wound closure ratio of H/G4-HA(Cu)/PANI apparently overpassed the other two groups. On day 14, the wounds in the H/G4-HA(Cu)/PANI group healed completely, whereas there were still about 8.25% and 5.5% remaining wound area for the blank group and the G4-HA group, respectively. The results showed that H/G4-HA(Cu)/PANI exhibited a better wound healing effect than the other two groups by tracing the wound contraction closure area, which was the comprehensive effect of the multifunctional inherent properties including antioxidant, bactericidal and haemostatic performances. It was evident that the two hydrogel groups completed the inflammatory phase on day 14, as shown by the hematoxylin and eosin staining (H&E staining) results (Fig. 6c). Inflammatory responses still existed on the wound site in the blank group on day 14, which delayed the wound healing processes, thus resulting the longest wound edge (4394 μm). In contrast, a shorter wound edge was observed for the G4-HA group (1352 μm) and the H/G4-HA(Cu)/PANI group (778 μm), respectively (Fig. 6d). These results were also consistent with the thickness of the granulation tissue (Fig. 6e). The granulation tissue is constituted by fibroblasts and growth factors, and a thicker granulation tissue wound be beneficial for wound healing. The granulation tissue thickness in the H/G4-HA(Cu)/PANI group was as high as 1613 μm in comparison with the other two groups. In correspondence with the migration of fibroblasts, collagen deposition was further evaluated by Masson's trichrome staining. As shown in Fig. 7a and b, the two hydrogel groups exhibited a higher level of collagen deposition compared to the blank group. More denser and regular collagen fibres were observed in the G4-HA and H/G4-HA(Cu)/PANI groups. Moreover, angiogenesis is a vital process for tissue remodelling. Fig. 7c and d show the immunofluorescence staining of CD31, a typical marker of vascular endothelial cells. The two hydrogel groups presented a higher level of the expression of CD31 compared to the blank group on day 14. The higher CD31 expression in the H/G4-HA(Cu)/PANI group compared to the G4-HA group might be due to the introduction of Cu2+, which exhibited multiple pharmacological properties. Overall, the above results showed that H/G4-HA(Cu)/PANI could accelerate wound healing by haemolysis, scavenging free radicals, killing bacteria, exhibiting conductivity, promoting collagen deposition and upregulating the CD31 level.
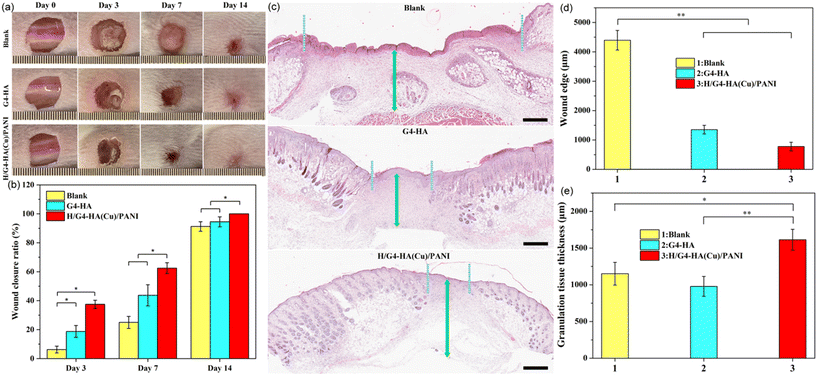 |
| Fig. 6 (a) Images of the wounds on day 0, day 3, day 7 and day 14. (b) The wound closure ratio of the different treatment groups. (c) The representative images of the haematoxylin and eosin (H&E) staining of the regenerated tissue on day 14. The light cyan dotted line represents the wound edge. The green arrow represents the granulation tissue thickness. Scale bar: 500 μm. Quantification data of the wound edge (d) and granulation tissue thickness (e). *p < 0.05 and **p < 0.01. | |
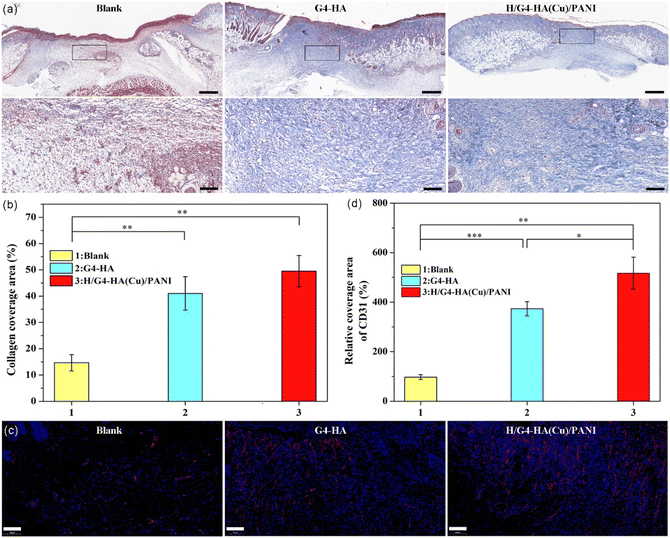 |
| Fig. 7 (a) The representative images of Masson's trichrome staining of the regenerated tissues on day 14. Scale bar: 500 μm, enlarged 100 μm. (b) The relative collagen coverage area (%). (c) Images of the immunofluorescence labelling of the wound tissues on day 14 with CD31. (d) The relative CD31 coverage area (%). Scale bar: 100 μm *P < 0.05, **P < 0.01 and ***P < 0.001. | |
4. Conclusions
A series of supramolecular-polymer hydrogels with inherent multiple properties was fabricated as wound dressings. These hydrogels were synthesized by combinatorially mixing AHHA, guanosine, KOH, Cu2+, hemin, aniline and H2O2. The introduction of Cu2+-crosslinked AHHA polymeric networks not only enhanced the viscoelasticity of the supramolecular guanosine hydrogel, but also endowed composite hydrogels with bioactive properties as wound healing dressings. The addition of hemin endowed the hydrogels with enzyme-like activity, which could catalyse the oxidative polymerization of aniline, thus introducing PANI into the gel networks. The target H/G4-HA(Cu)/PANI exhibited a porous gel matrix and could swell to a certain degree under physiological conditions. The degradability and appropriate mechanical properties of H/G4-HA(Cu)/PANI lay a solid foundation for the following in vivo experiments. Using a full-thickness skin defect model of mice, it was observed that this haemostatic, antioxidant, biocompatible, conductive and antibacterial H/G4-HA(Cu)/PANI hydrogel could accelerate wound healing processes by promoting wound closure and collagen deposition and upregulating the CD31 expression level. All these results indicated that the inherent multifunctional H/G4-HA(Cu)/PANI hydrogels could be utilized as promising wound dressing materials.
Author contributions
Qingcong Wei: conceptualization, experimental design, supervision, funding acquisition, and writing – review and editing. Yuke Wang: methodology, investigation and validation. Liyang Jia: methodology and investigation. Guanglei Ma: review and editing. Xiaofang Shi: review and editing. Weiwei Zhang: funding acquisition and review and editing. Zhiguo Hu: resources and review and editing.
Conflicts of interest
There are no conflicts to declare.
Acknowledgements
This work was financially supported by the following foundation: The Foundation of Henan Educational Committee (no. 20A150023, 21A150029 and 23B430001), and the Key Science and Technology Project of Henan Province (no. 212102310856).
References
- F. Chen, Q. Chen, L. Zhu, Z. Tang, Q. Li, G. Qin, J. Yang, Y. Zhang, B. Ren and J. Zheng, Chem. Mater., 2018, 30, 1743–1754 CrossRef CAS.
- S. Panja and D. J. Adams, Chem. Soc. Rev., 2021, 50, 5165–5200 RSC.
- C. C. Piras, A. G. Kay, P. G. Genever and D. K. Smith, Chem. Sci., 2021, 12, 3958–3965 RSC.
- C. Gu, Y. Peng, J. Li, H. Wang, X. Q. Xie, X. Cao and C. S. Liu, Angew. Chem., Int. Ed., 2020, 59, 18768–18773 CrossRef CAS PubMed.
- J. Hu, Q. Hu, X. He, C. Liu, Y. Kong, Y. Cheng and Y. Zhang, Adv. Healthcare Mater., 2020, 9, 1901329 CrossRef CAS PubMed.
- D. J. Cornwell and D. K. Smith, Mater. Horiz., 2015, 2, 279–293 RSC.
- B. O. Okesola, H. K. Lau, B. Derkus, D. K. Boccorh, Y. Wu, A. W. Wark, K. L. Kiick and A. Mata, Biomater. Sci., 2020, 8, 846–857 RSC.
- J. Yang, K. Li, C. Tang, Z. Liu, J. Fan, G. Qin, W. Cui, L. Zhu and Q. Chen, Adv. Funct. Mater., 2022, 32, 2110244 CrossRef CAS.
- Y. Liang, K. Wang, J. Li, H. Wang, X.-Q. Xie, Y. Cui, Y. Zhang, M. Wang and C.-S. Liu, Adv. Funct. Mater., 2021, 31, 2104963 CrossRef CAS.
- C. Gu, X.-Q. Xie, Y. Liang, J. Li, H. Wang, K. Wang, J. Liu, M. Wang, Y. Zhang, M. Li, H. Kong and C.-S. Liu, Energy Environ. Sci., 2021, 14, 4451–4462 RSC.
- H. Shigemitsu, R. Kubota, K. Nakamura, T. Matsuzaki, S. Minami, T. Aoyama, K. Urayama and I. Hamachi, Nat. Commun., 2020, 11, 3859 CrossRef PubMed.
- J. Wang, Z. Wang, J. Gao, L. Wang, Z. Yang, D. Kong and Z. Yang, J. Mater. Chem., 2009, 19, 7892–7896 RSC.
- F. Netti, M. Aviv, Y. Dan, S. Rudnick-Glick, M. Halperin-Sternfeld and L. Adler-Abramovich, Nanoscale, 2022, 14, 8525–8533 RSC.
- C. C. Piras, P. Slavik and D. K. Smith, Angew. Chem., Int. Ed., 2020, 59, 853–859 CrossRef CAS.
- Q. Wei, J. Duan, G. Ma, W. Zhang, Q. Wang and Z. Hu, J. Mater. Chem. B, 2019, 7, 2220–2225 RSC.
- S. Varela-Aramburu, L. Su, J. Mosquera, G. Morgese, S. M. C. Schoenmakers, R. Cardinaels, A. R. A. Palmans and E. W. Meijer, Biomacromolecules, 2021, 22, 4633–4641 CrossRef CAS PubMed.
- Q. Wei, Y. Chang, G. Ma, W. Zhang, Q. Wang and Z. Hu, J. Mater. Chem. B, 2019, 7, 6195–6201 RSC.
- P. Chakraborty, H. Oved, D. Bychenko, Y. Yao, Y. Tang, S. Zilberzwige-Tal, G. Wei, T. Dvir and E. Gazit, Adv. Mater., 2021, 33, 2008715 CrossRef CAS.
- P. R. A. Chivers and D. K. Smith, Chem. Sci., 2017, 8, 7218–7227 RSC.
- A. K. Patterson, L. H. El-Qarra and D. K. Smith, Chem. Commun., 2022, 58, 3941–3944 RSC.
- D. B. Amabilino, D. K. Smith and J. W. Steed, Chem. Soc. Rev., 2017, 46, 2404–2420 RSC.
- H. Zhang, K. Liu, Y. Gong, W. Zhu, J. Zhu, F. Pan, Y. Chao, Z. Xiao, Y. Liu, X. Wang, Z. Liu, Y. Yang and Q. Chen, Biomaterials, 2022, 287, 121673 CrossRef CAS PubMed.
- V. S. Saji, Mater. Today Adv., 2022, 14, 100239 CrossRef CAS.
- G. Raj, V. K. K. Dommeti, V. Pradeep, A. K. Veetil and R. Varghese, Mater. Chem. Front., 2022, 6, 1533–1542 RSC.
- G. M. Peters, L. P. Skala, T. N. Plank, B. J. Hyman, G. N. M. Reddy, A. Marsh, S. P. Brown and J. T. Davis, J. Am. Chem. Soc., 2014, 136, 12596–12599 CrossRef CAS PubMed.
- Y. Li, L. Su, Y. Zhang, Y. Liu, F. Huang, Y. Ren, Y. An, L. Shi, H. C. van der Mei and H. J. Busscher, Adv. Sci., 2022, 9, 2103485 CrossRef CAS PubMed.
- R. Zhong, Q. Tang, S. Wang, H. Zhang, F. Zhang, M. Xiao, T. Man, X. Qu, L. Li, W. Zhang and H. Pei, Adv. Mater., 2018, 30, 1706887 CrossRef.
- Y. Yuan, D. Fan, S. Shen and X. Ma, Chem. Eng. J., 2022, 433, 133859 CrossRef CAS.
- P. Zan, A. Than, W. Zhang, H. X. Cai, W. Zhao and P. Chen, ACS Nano, 2022, 16, 1813–1825 CrossRef CAS.
- S. Wang, H. Zheng, L. Zhou, F. Cheng, Z. Liu, H. Zhang and Q. Zhang, Biomaterials, 2020, 260, 120314 CrossRef CAS PubMed.
- W. Lu, M. Si, X. Le and T. Chen, Acc. Chem. Res., 2022, 55, 2291–2303 CrossRef CAS PubMed.
- Z. Liu, K. Zhang, G. Huang, B. Xu, Y.-l. Hong, X. Wu, Y. Nishiyama, S. Horike, G. Zhang and S. Kitagawa, Angew. Chem., Int. Ed., 2022, 61, e202110695 CAS.
- C. Lei, Y. Guo, W. Guan, H. Lu, W. Shi and G. Yu, Angew. Chem., Int. Ed., 2022, 61, e202200271 CAS.
- C. Zhang, B. Wu, Y. Zhou, F. Zhou, W. Liu and Z. Wang, Chem. Soc. Rev., 2020, 49, 3605–3637 RSC.
- S. Kim and M. Lee, Chem. Mater., 2020, 32, 9508–9530 CrossRef CAS.
- Y. Chen, J. Meng, Z. Gu, X. Wan, L. Jiang and S. Wang, Adv. Funct. Mater., 2020, 30, 1905287 CrossRef CAS.
- K. Zhang, Q. Feng, Z. Fang, L. Gu and L. Bian, Chem. Rev., 2021, 121, 11149–11193 CrossRef CAS.
- Y. Liang, J. He and B. Guo, ACS Nano, 2021, 15, 12687–12722 CrossRef CAS PubMed.
- M. Dong, B. Shi, D. Liu, J.-H. Liu, D. Zhao, Z.-H. Yu, X.-Q. Shen, J.-M. Gan, B.-l. Shi, Y. Qiu, C.-C. Wang, Z.-Z. Zhu and Q.-D. Shen, ACS Nano, 2020, 14, 16565–16575 CrossRef CAS.
- R. Yang, G. Li, C. Zhuang, P. Yu, T. Ye, Y. Zhang, P. Shang, J. Huang, M. Cai, L. Wang, W. Cui and L. Deng, Sci. Adv., 2021, 7, eabg3816 CrossRef CAS.
- Y. Guan, H. Niu, Z. Liu, Y. Dang, J. Shen, M. Zayed, L. Ma and J. Guan, Sci. Adv., 2021, 7, eabj0153 CrossRef CAS.
- J. Zhang, Y. Zheng, J. Lee, J. Hua, S. Li, A. Panchamukhi, J. Yue, X. Gou, Z. Xia, L. Zhu and X. Wu, Nat. Commun., 2021, 12, 1670 CrossRef CAS.
- Q. Wei, Y. Wang, H. Wang, L. Qiao, Y. Jiang, G. Ma, W. Zhang and Z. Hu, Carbohydr. Polym., 2022, 278, 119000 CrossRef CAS PubMed.
- M. Zhang, X. Qiao, W. Han, T. Jiang, F. Liu and X. Zhao, Carbohydr. Polym., 2021, 266, 118100 CrossRef CAS PubMed.
- X. D. Zhao, D. N. Pei, Y. X. Yang, K. Xu, J. Yu, Y. C. Zhang, Q. Zhang, G. He, Y. F. Zhang, A. Li, Y. L. Cheng and X. S. Chen, Adv. Funct. Mater., 2021, 31, 2009442 CrossRef CAS.
- C. Yang, J. Dawulieti, K. Zhang, C. Cheng, Y. Zhao, H. Hu, M. Li, M. Zhang, L. Chen, K. W. Leong and D. Shao, Adv. Funct. Mater., 2022, 32, 2111698 CrossRef CAS.
- Y. Huang, L. Mu, X. Zhao, Y. Han and B. Guo, ACS Nano, 2022, 16, 13022–13036 CrossRef CAS PubMed.
- H. Suo, M. Hussain, H. Wang, N. Zhou, J. Tao, H. Jiang and J. Zhu, Biomacromolecules, 2021, 22, 3049–3059 CrossRef CAS PubMed.
- Y. Q. Liang, Z. L. Li, Y. Huang, R. Yu and B. L. Guo, ACS Nano, 2021, 15, 7078–7093 CrossRef CAS PubMed.
- C. Zhou, C. Sheng, L. Gao, J. Guo, P. Li and B. Liu, Chem. Eng. J., 2021, 413, 127429 CrossRef CAS.
- W. Zhao, Y. Li, X. Zhang, R. Zhang, Y. Hu, C. Boyer and F. J. Xu, J. Controlled Release, 2020, 323, 24–35 CrossRef CAS PubMed.
- Y. W. Zhang, B. Yuan, Y. Q. Zhang, Q. P. Cao, C. Yang, Y. Li and J. H. Zhou, Chem. Eng. J., 2020, 400, 125984 CrossRef CAS.
- Q. Li, Y. Zhang, X. Huang, D. Yang, L. Weng, C. Ou, X. Song and X. Dong, Chem. Eng. J., 2021, 407, 127200 CrossRef CAS.
- J. Chen, N. Tao, S. Fang, Z. Chen, L. Liang, X. Sun, J. Li and Y.-N. Liu, New J. Chem., 2018, 42, 9651–9657 RSC.
- Y. Mao, T. Su, Q. Wu, C. Liao and Q. Wang, Chem. Commun., 2014, 50, 14429–14432 RSC.
- H. Shigemitsu, R. Kubota, K. Nakamura, T. Matsuzaki, S. Minami, T. Aoyama, K. Urayama and I. Hamachi, Nat. Commun., 2020, 11, 3859 CrossRef PubMed.
- C. C. Piras, P. G. Genever and D. K. Smith, Mater. Adv., 2022, 3, 7966–7975 RSC.
- X. Zhang, J. Wang, H. Jin, S. Wang and W. Song, J. Am. Chem. Soc., 2018, 140, 3186–3189 CrossRef CAS PubMed.
- Q. Wei, S. Jiang, R. Zhu, X. Wang, S. Wang and Q. Wang, iScience, 2019, 14, 27–35 CrossRef CAS PubMed.
- M. F. P. Graça, S. P. Miguel, C. S. D. Cabral and I. J. Correia, Carbohydr. Polym., 2020, 241, 116364 CrossRef PubMed.
- M. Li, X. Shi, B. Yang, J. Qin, X. Han, W. Peng, Y. He, H. Mao, D. Kong and Z. Gu, Carbohydr. Polym., 2022, 296, 119953 CrossRef CAS PubMed.
- J. Qian, L. Ji, W. Xu, G. Hou, J. Wang, Y. Wang and T. Wang, ACS Appl. Mater. Interfaces, 2022, 14, 16018–16031 CrossRef CAS PubMed.
- C. Dong, W. Feng, W. Xu, L. Yu, H. Xiang, Y. Chen and J. Zhou, Adv. Sci., 2020, 7, 2001549 CrossRef CAS PubMed.
- A. Wang, G. Fan, H. Qi, H. Li, C. Pang, Z. Zhu, S. Ji, H. Liang, B.-P. Jiang and X.-C. Shen, Biomaterials, 2022, 289, 121798 CrossRef CAS PubMed.
- Q. Zhang, Y. Lv, M. Liu, X. Wang, Y. Mi and Q. Wang, J. Mater. Chem. B, 2018, 6, 1402–1409 RSC.
- X. Wang and Q. Wang, Acc. Chem. Res., 2021, 54, 1274–1287 CrossRef CAS PubMed.
- X. Wang, S. Chen, D. Wu, Q. Wu, Q. Wei, B. He, Q. Lu and Q. Wang, Adv. Mater., 2018, 30, 1705668 CrossRef PubMed.
- K. Peng, J. Zhang, J. Yang, L. Lin, Q. Gan, Z. Yang, Y. Chen and C. Feng, ACS Appl. Mater. Interfaces, 2022, 14, 39404–39419 CrossRef CAS PubMed.
- A. M. R. Rebelo, Y. Liu, C. Liu, K.-H. Schäfer, M. Saumer and G. Yang, ACS Biomater. Sci. Eng., 2019, 5, 2160–2172 CrossRef CAS PubMed.
- L. Fan, C. Liu, X. Chen, L. Zheng, Y. Zou, H. Wen, P. Guan, F. Lu, Y. Luo, G. Tan, P. Yu, D. Chen, C. Deng, Y. Sun, L. Zhou and C. Ning, Adv. Sci., 2022, 9, 2105586 CrossRef CAS PubMed.
|
This journal is © The Royal Society of Chemistry 2023 |