DOI:
10.1039/D2AY01786E
(Paper)
Anal. Methods, 2023,
15, 48-55
An instrument-free visual quantitative detection method based on clock reaction: the detection of thrombin as an example†
Received
1st November 2022
, Accepted 23rd November 2022
First published on 24th November 2022
Abstract
Instrument-free visual quantitative detection in chemical and biochemical analysis is of great significance in practical applications especially in point-of-care testing and in places where resources are limited. In this paper, we report the development of a time-based instrument-free visual quantitative detection method by employing a clock reaction, a type of chemical reaction displaying characteristic clocking behavior. The feasibility of the method was illustrated by the quantitative detection of thrombin in buffer solution using the lapse of time as the readout signal. The linear range of detection was from 1.3 to 43 nM (r2 = 0.990, n = 3) with a LOD of 0.9 nM, which is lower than the physiological concentrations of thrombin in the resting and activated blood, which range from low nanomolar to low micromolar, respectively. This method was also validated by detecting thrombin in the serum and a good recovery of nearly 100 ± 8.0% was obtained. To the best of our knowledge, this work is the first report that uses the characteristic time of a clock reaction as the readout signal in instrument-free colorimetry for quantitative bioanalysis.
1. Introduction
A common phenomenon across analytical methods is that quantitative detection often requires instruments. For example, optical quantitative detection requires optical instruments1–3 and electrical quantitative detection requires electrical instruments.4 These instruments are often expensive and complicated to operate, requiring trained personnel to use them. For biochemical and biomedical applications, this contradicts the World Health Organization's (WHO) criteria that tests are affordable, sensitive, specific, user-friendly, rapid, robust, equipment-free, and deliverable to end-users (so-called ASSURED guideline) for point-of-care (POC) diagnosis in places with limited resources. Therefore, the development of an instrument-free quantitative detection method is particularly important to meet the high demand. However, to the best of our knowledge, instrument-free visual quantitative detection is an underdeveloped area. At present, the reported methods can be divided into two categories: scale-ruler-based and time-based instrument-free visual quantitative detection, respectively.
Scale-ruler-based instrument-free visual quantitative detection can be further divided into two categories: the distance of colored agents (usually red ink) moving in the channel, and the distance of the color change region. The first method of classification mainly uses the volumetric bar-chart chip, with the basic principle being that different analyte concentrations will lead to different concentrations of gas products (usually oxygen). The gas is stored in a limited space, and the more gas, the greater the pressure, which pushes the red ink farther. This type of work is the most developed, and a lot of work has been published.5–9 The basic principle of the second category is that when the analyte moves in the channel (usually a paper-based microfluidic channel), it will react with substrate pre-loaded in the channel to form insoluble and colored precipitates and will accumulate in the channel. The higher the concentration of the analyte, the more insoluble colored precipitates will accumulate in the channel, and the longer the colored band will be on the paper. Subsequently, more work based on this principle has been reported, and the objects of detection have become increasingly abundant.10–12
Compared with the scale-ruler-based instrument-free visual quantitative detection, time-based detection is much less studied and rarely reported. So far, the reported methods of this approach can also be divided into two categories according to their different mechanisms. One category uses the time required for the colored substance to move from the start point to the endpoint. The other category uses the relationship between the analyte concentration and the time interval between the switch of two colors. The first category of methods detects the time difference caused by the different wetting properties of the channel.13–16 In the second category of methods, the analyte concentration is detected by using the analyte to affect the rate of a certain reaction involving color change and recording the time required for the color change. There are two main de-coloration reactions used. The first is the reduction of p-nitrophenol by sodium borohydride, with the color changing from yellow to colorless.17,18 The second de-coloration reaction is the reduction of methylene blue by sodium borohydride and then re-oxidation by oxygen. The color changes from blue to colorless and then back to blue.19–22
The development of time-based instrument-free visual quantitative detection is a much less developed method. In this paper, we choose to use time as the signal readout for instrument-free visual quantitative detection by making use of a clock reaction. Clock reactions, chemical reactions displaying clocking behavior, have been known for over a century.23–27 Landolt first discovered clock reactions through a sulfite/iodate reaction.28,29 Specifically, the iodine is formed repeatedly in a fixed time interval, defined as Landolt time, to which the clock can be calibrated; thus, it is called a clock reaction. It was later found that Landolt time is affected by many factors, including the concentration of reactants, the type, and concentration of catalysts, temperature, etc.30 Thrombin plays an essential role in blood coagulation as well as in some physiological and pathological processes. Various diseases are related to the concentration of thrombin in human blood.2,3,31 Therefore, this paper chooses the instrument-free quantitative visual detection of thrombin by constructing the relationship between analyte concentration and Landolt time as an example (referred to as cycle time in the following). Although there have been reported the instrument-free visual quantitative detection of thrombin by recording the time of reducing p-nitrophenol,17 to the best of our knowledge, this is the first work using clock reaction to achieve instrument-free visual quantitative detection of biomolecules. At the same time, the detailed scheme of the clock reaction applied in instrument-free visual quantitative detection was firstly discussed in this work.
2. Experimental section
2.1 Materials
Gold(III) chloride trihydrate, sodium borohydride, methylene blue, thrombin from bovine plasma, tris-(hydroxymethyl)-aminomethane, sodium chloride, and human serum, are all from Sigma-Aldrich and used as received without further purification. Sodium citrate (Fisher Scientific), thrombin aptamer (Sangon Biotech), tris-(2-carboxyethyl)-phosphine hydrochloride (Bio-Basic), hydrochloric acid (VWR chemicals), acetic acid glacial (VWR chemicals), and sodium acetate (Fisher Scientific), are received from respective suppliers and were used without any further treatment.
2.2 Instruments
The UV-vis spectra were recorded by using a UV-1800 spectrometer (Shimadzu, Japan). The cycle time was recorded by timer. The AuNPs were measured with JEOL 2010F Analytical Transmission Electron Microscope (TEM). Ultrapure water from a NANOpure (Barnstead) source was used throughout the experiments. Excessive thrombin aptamer that is not modified on the AuNPs was removed by centrifuge 5418 R (Eppendorf). The constant temperature condition of 37 °C was provided by the incubator (Memmert).
2.3 Preparation and characterization of AuNPs
The fabrication of AuNPs was based on the popular approaches using citrate reduction of HAuCl4 in water.32 Briefly, 72.73 mL aqueous solution with 1 mmol L−1 HAuCl4 was added into a round-bottom flask equipped with a reflux condenser and then heated to boil under gentle stirring. 38.8 mmol L−1 sodium citrate (7.27 mL) was added rapidly to the solution. The solution was heated under reflux for another 15.0 min. The color changed from pale yellow to deep red. The solution was stirred continuously and cooled down to room temperature. Finally, the AuNPs were obtained and stored at 4 °C for further use. The achieved AuNPs were analyzed with JEOL 2010F Analytical Transmission Electron Microscope (TEM). As can be seen from the TEM image (Fig. S1A†) the as-synthesized AuNPs are quite uniform in size and shape with an average size of ca. 13 nm. The UV-vis spectra of the synthesized AuNPs were shown in Fig. S1B,† the peak wavelength was 520 nm.
2.4 Preparation of AuNPs modified with thrombin aptamer
20.0 μL of 100 μM thrombin aptamer, 5.6 μL of 200 mM, pH 5.20 acetate buffer, and 3.3 μL of 1.0 mM TCEP were mixed for one hour at room temperature. 300.0 μL of 2.0 nM AuNPs was then added to the mixture at room temperature. After 16 hours, 30.0 μL of 200 mM, pH 8.20 Tris–HCl, and 30.0 μL of 1.0 M NaCl were added into the mixture with gentle handshaking at room temperature for 24 hours. The achieved mixture was then centrifuged at 14
000 rpm (23 °C) for 10.0 min. Then, the supernatant was discarded, and after that, the precipitate was redissolved by 300.0 μL of pH 7.10 Tris–HCl, and 30.0 μL of 1 M NaCl. Finally, the achieved AuNPs modified with labeling antibodies are stored dark place at room temperature for further use.
2.5 The procedure for the optimization experiments
Optimization of the concentration of modified AuNPs. The conditions are 50.0 μL of 43 nM, or 0 nM thrombin, and 50.0 μL of different concentrations of modified AuNPs were incubated for 30 min at 37 °C. 300.0 μL of 10 μM MB (dissolved by pH 8.70 Tris–HCl buffer) was injected into the mixture. Finally, 100.0 μL of ω = 0.019% NaBH4 was added for observation of the cycle time.
Optimization of pH. The conditions are 50.0 μL of 43 nM or 0 nM thrombin, and 50.0 μL of 0.074 nM modified AuNPs were incubated for 30 min at 37 °C. 300.0 μL of 10 μM MB (dissolved by different pH Tris–HCl buffer) was injected into the mixture. Finally, 100.0 μL of ω = 0.019% NaBH4 was added for observation of the cycle time.
Optimization of the concentration of NaBH4. The conditions are 50.0 μL of 43 nM or 0 nM thrombin, and 50.0 μL of 0.074 nM modified AuNPs were incubated for 30 min at 37 °C. 300.0 μL of 10 μM MB (dissolved by pH 8.70 Tris–HCl buffer) was injected into the mixture. Finally, 100.0 μL of different concentrations of NaBH4 were added for observation.
Optimization of MB concentration. The conditions are 50.0 μL, 43 nM or 0 nM thrombin, and 50.0 μL of 0.074 nM modified AuNPs were incubated for 30 min at 37 °C. 300.0 μL, different concentrations of MB (dissolved by pH 8.70 Tris–HCl buffer) were injected into the mixture. Finally, 100.0 μL, ω = 0.019% NaBH4 was added for observation.
2.6 The procedure of illustrating the detection performance
50.0 μL of different concentrations of thrombin dissolved in water and serum or possible interferences and 50.0 μL of 0.074 nM modified AuNPs were incubated for 30.0 min at 37 °C. Then, 300.0 μL of 10 μM MB+ (dissolved by pH 8.70 Tris–HCl buffer) was injected into the mixture and showed blue color (Fig. S1C†). Finally, 100.0 μL of ω = 0.019% NaBH4 was added for observation and start the timer. After the color changes from navy blue to colorless and back to light blue (0.68 μM MB+, absorbance is 0.05), stop the timer and record the time of the color changes from blue to colorless and back to light blue (defined as cycle time).
3. Results and discussion
3.1 The procedure of the method
The experimental procedure is illustrated in Fig. 1. When AuNPs modified with thrombin aptamer are mixed with thrombin, the thrombin will be specifically identified by the thrombin aptamer and form a G-quadruplex structure.33 The loose structure of the thrombin aptamer will become a rigid structure that is a G-quadruplex structure, which masks the partial surface of AuNPs. The higher concentration of thrombin, the more surface area of AuNPs will be masked, which will affect the cycle time of the clock reaction. Therefore, the higher concentration of thrombin, the more surface area of AuNPs will be masked, and a shorter cycle time will be achieved. Finally, we can detect the concentration of thrombin through cycle time.
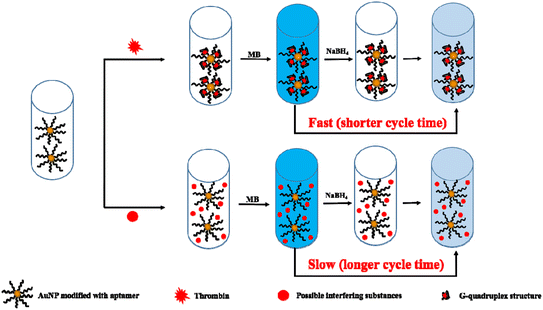 |
| Fig. 1 Procedure illustrating the instrument-free visual quantitative detection of thrombin based on clock reaction. | |
3.2 The principle concept of the method
|
BH4−(aq) + H+(aq) + 3H2O(l) + 4MB+(aq) → 4rMB(aq) +H3BO3(aq)
| (1) |
|
O2(aq) + 2rMB(aq) → 2MB+(aq) + 2H2O(l)
| (2) |
|
| (3) |
|
BH4−(aq) + 2H2O(l) → BO2−(aq) + 4H2(g)
| (4) |
|
MB+(aq) + H2(g) → rMB(aq)
| (5) |
As shown in Fig. 2, when NaBH4 is added to the solution, the rates of reactions (1), (4), and (5), are boosted because of the existence of AuNPs. The dissolved oxygen will be degassed and consumed, and [O2(aq)] will finally approach zero, which shuts down the reaction (2). The color will then quickly change from navy blue to colorless (less than 3 min). In this procedure, rate (2) > rate (3) and rate (1) + rate (5) > rate (2). However, reactions (1), and (4) consume NaBH4. As time goes on, [BH4−] will decrease to the critical point where the reaction (1) does not belong to the pseudo-first-order reaction in [MB+] because the condition for [BH4−] ≫ [MB+] does not exist.34 Hence, rate (1) will be positively correlated with [BH4−], [MB+], and the catalytic performance of the AuNPs. Therefore, as NaBH4 is consumed, rates (1), and (5) will decrease, resulting in rate (3) > rate (2) and rate (2) > rate (1) + rate (5). Under this condition, it will produce MB+, and the solution become blue. As described in this process, the time for changing blue color to colorless and back to light blue color (cycle time) depends on the duration of the condition that is the rate (2) > rate (3) and rate (1) + rate (5) > rate (2), which is related to [BH4−], [O2(aq)], [O2(air)] and the catalytic performance of AuNPs, and the diffusion rate of O2 from air to solution. However, [O2(aq)], [O2(air)] and the diffusion rate of O2 (the air–liquid area is certain) from air to solution are certain in this process. Therefore, cycle time mainly depends on [BH4−], and the catalytic performance of AuNPs. The higher concentration of thrombin, the more surface area of AuNPs will be masked, the lower catalytic performance of AuNPs, the shorter duration time of the condition rate (2) > rate (3) and rate (1) + rate (5) > rate (2), the shorter cycle time. Finally, we can detect the concentration of thrombin based on the cycle time.
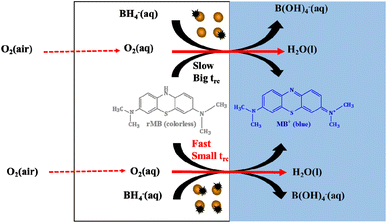 |
| Fig. 2 The scheme of the catalytic regulation of cycle time by varying the passivation states of AuNPs. | |
3.3 Optimization of the key assay conditions
As the catalyst of the clock reaction, the concentration of modified AuNPs will affect the cycle time. Therefore, we explored the effect of the concentration of modified AuNPs on cycle time. As shown in Fig. 3A, the cycle time for 43 nM and 0 nM thrombin are both positively correlated with the concentration of modified AuNPs. As illustrated in the scheme of the method, the higher concentration of AuNPs will result in the higher catalytic performance of AuNPs, the longer duration time of the condition rate (7) + rate (2) > rate (4), and rate (1) + rate (6) > rate (2), the longer cycle time. Consideration of the sensitivity and efficiency of this method, that is the difference in cycle time between 43 nM and 0 nM thrombin, 0.074 nM AuNPs show the highest sensitivity for this experiment. Thus, 0.074 nM AuNPs were chosen for the experiment.
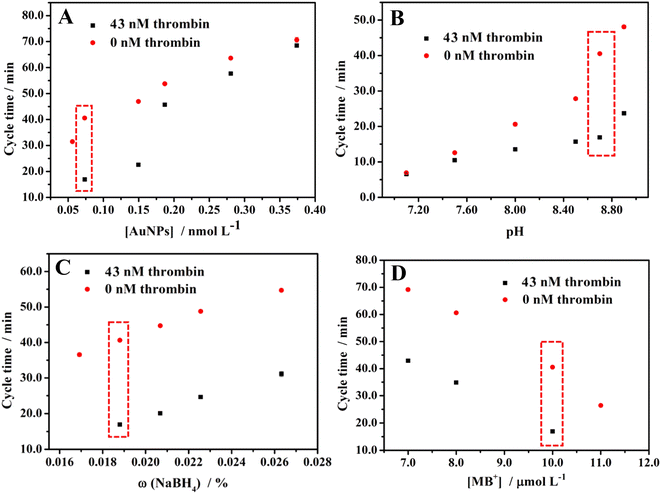 |
| Fig. 3 Effects of (A) the concentration of modified AuNPs, (B) pH, (C) NaBH4 concentration, and (D) MB+ concentration on the cycle time. | |
The pH will affect the hydrolysis rate of NaBH4, which affects the cycle time. Therefore, the effect of pH on cycle time was explored. As shown in Fig. 3B, the cycle time for 43 nM and 0 nM thrombin are both positively correlated with pH. The higher pH means the slower hydrolysis of NaBH4, which leads to arrive the condition rate (7) < rate (4) and rate (1) + rate (6) < rate (2) later, in other words, the longer cycle time. After considering the sensitivity and efficiency of this method, finally, pH 8.70 was selected for detection.
As the reactant of the clock reaction, the concentration of NaBH4 will affect the cycle time. Therefore, explored the effect of the concentration of NaBH4 on the cycle time. As shown in Fig. 3C, the cycle time for 43 nM and 0 nM thrombin are both positively correlated with the concentration of NaBH4. The higher concentration of NaBH4 results in a longer cycle time. Considering the sensitivity and efficiency of this method, we chose ω = 0.019% NaBH4 as the optimal experiment condition.
As with the NaBH4, we also explored the effect of the concentration of MB+ on cycle time. Fig. 3D shows that the cycle time for 43 nM and 0 nM thrombin are both negatively correlated with the concentration of MB+. With a higher concentration of MB+, the NaBH4 is consumed faster, then the cycle time will be shorter. Therefore, 10.0 μM MB+ was selected for the experiments after considering the sensitivity and efficiency of this method.
3.4 The instrument-free visual quantitative detection of thrombin
After obtaining the optimal conditions, this method was applied to detect thrombin. The results are shown in Fig. 4. Fig. 4A shows the cycle time of each thrombin concentration observed with the naked eye and records their color at the start and endpoints. The cycle times are 16.9, 19.6, 23.6, 29.3, 32.7, 38.4, and 40.5 min obtained with the naked eye for 43, 21, 11, 5.3, 2.7, 1.3, and 0 nM, respectively. The limit of detection (LOD: the thrombin concentration corresponding to a signal that is three times the standard deviation above zero calibrators) of this assay was measured, where the concentration of thrombin was increased from 0 to 43 nM and the cycle time ranged from 40.5 to 16.9 min. The calibration curve of the cycle time against thrombin concentration was linear from 1.3 to 43 nM (r2 = 0.990, n = 3) with an LOD of 0.9 nM (Fig. 4B). The LOD of this method is lower than the physiological concentrations of thrombin in resting and activated blood, which ranges from low nanomolar to low micromolar, respectively.35,36 This indicates the actual application value of this method. As instrument-free visual quantitative detection of thrombin, Table 1 summarizes the unique advantage of this method compared with other methods. The color changes for each concentration of thrombin during the clock reaction were also recorded with a UV-vis spectrometer, the results are shown in Fig. 4C. The cycle time are 17.0, 19.5, 23.8, 28.6, 32.3, 38.8, and 40.7 min obtained with UV-vis spectrometer for 43, 21, 11, 5.3, 2.7, 1.3, and 0 nM, respectively. After comparing the cycle time obtained by the naked eye and the cycle time obtained by UV-vis spectrometer, it was found that they are close. This demonstrates that the cycle times observed with the naked eye are reliable.
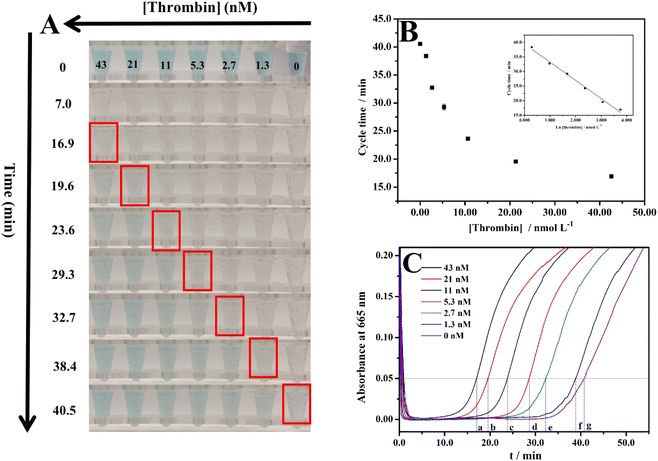 |
| Fig. 4 (A) Color changes of MB+ in the clock reaction along with the time for different concentrations of thrombin; (B) corresponding plot of time observed by the naked eye vs. the concentration of thrombin. Inset: calibration plot of thrombin; (C) absorbance at 665 nm (a characteristic peak of MB+, showed in Fig. S1C†) changes of the systems along with the time—a, b, c, d, e, f, and g represent the times when the absorbance at 665 nm is 0.05. | |
Table 1 Comparison with other thrombin colorimetric detection methods
Material |
Linear range (nM) |
LOD (nM) |
Instrument-free visual quantitative detection |
Ref. |
AgNPs |
0.02–5 |
0.02 |
No |
37
|
Fe3O4@AuNPs |
5–30.4 |
3 |
No |
38
|
AuNPs |
10–5000 |
7.5 |
No |
39
|
AuNPs |
0.0001–0.01 |
0.00004 |
No |
40
|
Fe3O4 |
0.108–27000 |
0.027 |
No |
41
|
PtNPs |
0.005–1 |
0.00167 |
No |
42
|
GO–AuPt NPs |
0.3–100 |
0.15 |
No |
43
|
Ag/Pt nanoclusters |
1–50 |
2.6 |
No |
44
|
AgNPs |
0.4–1.0 |
0.2 |
No |
45
|
AuNPs |
0.0001–100 |
0.00002 |
No |
46
|
AuNPs |
1.3–43 |
0.9 |
Yes |
This work |
3.5 The selectivity of the method in the presence of potential interferents
We tested the selectivity of this method toward thrombin, investigated the cycle times for the various possible interferences, and compared them with the cycle time for thrombin alone. This included bovine serum albumin (BSA), immunoglobulin G (IgG), lysozyme (Lys), uric acid (UA), glucose, and ascorbic acid (AA). We also studied the cycle time of thrombin mixed with interference and then compared them with the cycle time for thrombin alone. Fig. 5A shows the colors of the 5.3 nM interfering substance, and 5.3 nM thrombin after 0, 7.0, 29.3, and 41.4 min of reaction. The corresponding cycle times were listed in Fig. 5B and Table S1.† The cycle times for the interfering substances are longer than the cycle time for thrombin and are close to the cycle time for the blank experiment. These results illustrate that the single interfering substance is similar to the blank result and will not affect thrombin detection. To further illustrate the high selectivity of this method, we also explored a binary system that compared the cycle time for thrombin along with the cycle time for thrombin mixed with interfering substances. The results are listed in Fig. 5C and D, and Table S1.†Fig. 5C shows the colors of 5.3 nM thrombin, and 5.3 nM thrombin + 5.3 nM interfering substance after 0, 7.0, and 31.0 minutes of reaction. Fig. 5D and Table S1† show the cycle time for 5.3 nM thrombin and 5.3 nM thrombin + 5.3 nM interfering substance. After comparing the cycle time for 5.3 nM thrombin and the cycle time for 5.3 nM thrombin + 5.3 nM interfering substance, it was found that they are close, which demonstrates even the coexistence of interfering substances and thrombin will not affect the test results. In summary, these results demonstrate the high selectivity of the method.
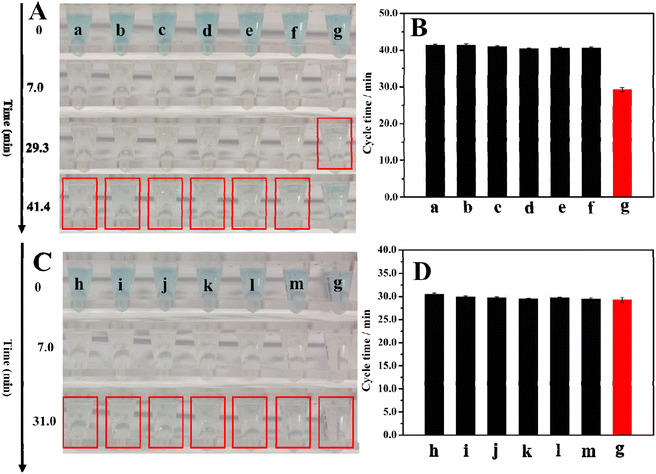 |
| Fig. 5 (A) Color changes of MB+ in the clock reaction along with the time for 5.3 nM BSA (a), 5.3 nM IgG (b), 5.3 nM Lys (c), 5.3 nM UA (d), 5.3 nM glucose (e), 5.3 nM AA (f), and 5.3 nM thrombin (g); (B) histogram of cycle time towards 5.3 nM BSA (a), 5.3 nM IgG (b), 5.3 nM Lys (c), 5.3 nM UA (d), 5.3 nM glucose (e), 5.3 nM AA (f), and 5.3 nM thrombin (g); (C) color changes of MB+ in the clock reaction along with the time for 5.3 nM BSA + 5.3 nM thrombin (h), 5.3 nM IgG + 5.3 nM thrombin (i), 5.3 nM Lys + 5.3 nM thrombin (j), 5.3 nM UA + 5.3 nM thrombin (k), 5.3 nM glucose + 5.3 nM thrombin (l), 5.3 nM AA + 5.3 nM thrombin (m), and 5.3 nM thrombin(g); (D) histogram of cycle time towards 5.3 nM BSA + 5.3 nM thrombin (h), 5.3 nM IgG + 5.3 nM thrombin (i), 5.3 nM Lys + 5.3 nM thrombin (j), 5.3 nM UA + 5.3 nM thrombin (k), 5.3 nM glucose + 5.3 nM thrombin (l), 5.3 nM AA + 5.3 nM thrombin (m), and 5.3 nM thrombin(g). | |
3.6 The validation of this method for applications in human serum
After obtaining the standard curve and proving the high selectivity of the method, to demonstrate its practicality, we used the method to detect the real samples (detection of thrombin in human serum). The results are listed in Table S2.† Every test was repeated three times. The recoveries are in the range of 92.7% to 108%, which demonstrates that this developed method is potentially applicable for the detection of thrombin in human serum.
4. Conclusions
In conclusion, we reported an instrument-free visual quantitative detection based on clock reaction. The feasibility of the method was demonstrated by applying it to the instrument-free visual quantitative detection of thrombin in both aqueous solution and in human serum. What's more, the principle of our method of using clock reaction for the instrument-free visual quantitative detection of bioanalyte was illustrated for the first time. For thrombin, the detection limit of this method was found to be 0.9 nM and the detection range from 1.3 to 43 nm. The LOD of this method is lower than the physiological concentrations of thrombin in the resting and activated blood, indicating that it is potentially a viable method for practical application. This method was further validated by detecting thrombin in human serum, and a very good recovery was achieved, indicating its feasibility for practical applications. The detection performance has been compared with some published articles on thrombin detection. The instrument-free visual quantitative detection of this method has a unique advantage, which can be used in a variety of circumstances where the access to a laboratory infrastructure is not available.
Conflicts of interest
The authors declare that they have no known competing financial interests or personal relationships that could have appeared to influence the work reported in this paper.
Acknowledgements
This research was supported by the Department of Chemistry of HKUST, University Grants Committee of Hong Kong (No. 16307721).
References
- R. Elghanian, J. J. Storhoff, R. C. Mucic, R. L. Letsinger and C. A. Mirkin, Science, 1997, 277, 1078–1081 CrossRef.
- J. Yoon, N. Choi, J. Ko, K. Kim, S. Lee and J. Choo, Biosens. Bioelectron., 2013, 47, 62–67 CrossRef.
- M. Shen, Y. Wang and X. Kan, J. Mater. Chem. B, 2021, 9, 4249–4256 RSC.
- R. Ida and G. Wu, J. Am. Chem. Soc., 2008, 130, 3590–3602 CrossRef PubMed.
- Y. Song, Y. Zhang, P. E. Bernard, J. M. Reuben, N. T. Ueno, R. B. Arlinghaus and L. Qin, Nat. Commun., 2012, 3, 1–9 Search PubMed.
- T. Li, G. Ou, X. Chen, Z. Li, R. Hu, Y. Li, Y. Yang and M. Liu, Anal. Chim. Acta, 2020, 1130, 20–28 CrossRef PubMed.
- X. Liu, Y. Wang and Y. Song, Biosens. Bioelectron., 2018, 117, 644–650 CrossRef.
- Y. Li, J. Xuan, Y. Song, W. Qi, B. He, P. Wang and L. Qin, ACS Nano, 2016, 10, 1640–1647 CrossRef CAS PubMed.
- Y. Song, X. Xia, X. Wu, P. Wang and L. Qin, Angew. Chem., Int. Ed., 2014, 10, 12451–12455 Search PubMed.
- R. F. Zuk, V. K. Ginsberg, T. Houts, J. Rabbie, H. Merrick, E. F. Ullman and D. J. Litman, Clin. Chem., 1985, 31, 1144–1150 CrossRef.
- D. M. Cate, W. Dungchai, J. C. Cunningham, J. Volckens and C. S. Henry, Lab Chip, 2013, 13, 2397–2404 RSC.
- T. Tian, Y. An, Y. Wu, Y. Song, Z. Zhu and C. Yang, ACS Appl. Mater. Interfaces, 2017, 9, 30480–30487 CrossRef PubMed.
- G. G. Lewis, M. J. Ditucci and S. T. Phillips, Angew. Chem., Int. Ed., 2012, 51, 12707–12710 CrossRef PubMed.
- G. G. Lewis, J. S. Robbins and S. T. Phillips, Chem. Commun., 2014, 50, 5352–5354 RSC.
- Y. Zhang, J. L. Fan, J. F. Nie, S. W. Le, W. Y. Zhu, D. Gao, J. N. Yang, S. B. Zhang and J. P. Li, Biosens. Bioelectron., 2015, 73, 13–18 CrossRef CAS PubMed.
- G. G. Lewis, J. S. Robbins and S. T. Phillips, Anal. Chem., 2013, 85, 10432–10439 CrossRef CAS.
- B. H. Kim, I. S. Yoon and J. S. Lee, Anal. Chem., 2013, 85, 10542–10548 CrossRef CAS.
- X. Wei, Z. Chen, L. Tan, T. Lou and Y. Zhao, Anal. Chem., 2017, 89, 556–559 CrossRef CAS.
- S. K. Lee, M. Sheridan and A. Mills, Chem. Mater., 2005, 17, 2744–2751 CrossRef CAS.
- T. Wu and Z. Ma, Microchim. Acta, 2017, 184, 577–582 CrossRef CAS.
- T. Wu, J. Shan and Z. Ma, ACS Sustainable Chem. Eng., 2017, 5, 4976–4981 CrossRef CAS.
- L. Zheng, H. Yu, Y. Yue, F. Wu and Y. He, ACS Appl. Mater. Interfaces, 2017, 9, 11798–11802 CrossRef.
- A. K. Horváth and I. Nagypál, ChemPhysChem, 2015, 16, 588–594 CrossRef.
- R. T. P. E. Sant'Anna, V. Monteiro, J. R. T. Pereira and R. B. Faria, PLoS One, 2013, 8, 8–11 CrossRef PubMed.
- G. Lente, G. Bazsa and I. Fábián, New J. Chem., 2007, 31, 1707 RSC.
- M. Galajda, G. Lente and I. Fábián, J. Am. Chem. Soc., 2007, 129, 7738–7739 CrossRef PubMed.
- P. Oliveira and R. B Faria, J. Am. Chem. Soc., 2005, 127, 18022–18023 CrossRef PubMed.
- H. Landolt, Chem. Ber., 1885, 18, 56–57 CrossRef.
- H. Landolt, Chem. Ber., 1886, 19, 1317–1365 CrossRef.
- A. K. Horváth, I. Nagypál and G. Cseko, J. Phys. Chem. A, 2008, 112, 7868–7872 CrossRef PubMed.
- R. Du, L. Zhu, J. Gan, Y. Wang, L. Qiao and B. Liu, Anal. Chem., 2016, 88, 6767–6772 CrossRef PubMed.
- J. Turkevich, P. C. Stevenson and J. Hillier, Discuss. Faraday Soc., 1951, 11, 55–57 RSC.
- C. Riccardi, E. Napolitano, C. Patella, D. Musumeci and D. Montesarchio, Pharmacol. Ther., 2021, 217, 107649–107700 CrossRef CAS PubMed.
- B. R. Ganapuram, M. Alle, R. Dadigala, A. Dasari, V. Maragoni and V. Guttena, Int. Nano Lett., 2015, 5, 215–222 CrossRef CAS.
- Y. Xiao, A. A. Lubin, A. J. Heeger and K. W. Plaxco, Angew. Chem., Int. Ed., 2005, 117, 5592–5595 CrossRef.
- M. Lee and D. R. A. Walt, Anal. Biochem., 2000, 282, 142–146 CrossRef CAS.
- Y. Zhao, X. Liu, J. Li, W. Qiang, L. Sun, H. Li and D. Xu, Microfluidic chip-based silver nanoparticles aptasensor for colorimetric detection of thrombin, Talanta, 2016, 150, 81 CrossRef CAS.
- C. Riccardi, E. Napolitano, C. Platella, D. Musumeci and D. Montesarchio, G-quadruplex-based aptamers targeting human thrombin: Discovery, chemical modifications and antithrombotic effects, Pharmacol. Ther., 2021, 217, 107649 CrossRef CAS.
- L. Li, Y. Liang, Y. Zhao and Z. Chen, Target binding and DNA hybridization-induced gold nanoparticle aggregation for colorimetric detection of thrombin, Sens. Actuators, B, 2018, 262, 733 CrossRef CAS.
- C. K. Chen, C. C. Huang and H. T. Chang, Label-free colorimetric detection of picomolar thrombin in blood plasma using a gold nanoparticle-based assay, Biosens. Bioelectron., 2010, 25, 1922 CrossRef CAS PubMed.
- M. Shen, Y. Wang and X. Kan, Dual-recognition colorimetric sensing of thrombin based on surface-imprinted aptamer-Fe3O4, J. Mater. Chem. B, 2021, 9, 4249 RSC.
- T. Cheng, X. Li, P. Huang, H. Wang, M. Wang and W. Yang, Colorimetric and electrochemical (dual) thrombin assay based on the use of a platinum nanoparticle modified metal-organic framework (type Fe-MIL-88) acting as a peroxidase mimic, Microchim. Acta, 2019, 186, 6 CrossRef.
- L. Wang, W. Yang, T. F. Li, D. Li, Z. M. Cui, Y. Wang, S. L. Ji, Q. X. Song, C. Shu and L. Ding, Colorimetric determination of thrombin by exploiting a triple enzyme-mimetic activity and dual-aptamer strategy, Microchim. Acta, 2017, 184, 3145 CrossRef CAS.
- C. Zheng, A. X. Zheng, B. Liu, X. L. Zhang, Y. He, J. Li, H. H. Yang and G. N. Chen, One-pot synthesized DNA-templated Ag/Pt bimetallic nanoclusters as peroxidase mimics for colorimetric detection of thrombin, Chem. Commun., 2014, 50, 13103 RSC.
- J. Li, W. Li, W. Qiang, X. Wang, H. Li and D. Xu, A non-aggregation colorimetric assay for thrombin based on catalytic properties of silver nanoparticles, Anal. Chim. Acta, 2014, 807, 120 CrossRef CAS.
- L. Zhang and L. Li, Colorimetric thrombin assay using aptamer-functionalized gold nanoparticles acting as a peroxidase mimetic, Microchim. Acta, 2016, 183, 485 CrossRef CAS.
|
This journal is © The Royal Society of Chemistry 2023 |