DOI:
10.1039/D2TB00717G
(Paper)
J. Mater. Chem. B, 2022,
10, 7600-7606
Metal-phenolic networks as tuneable spore coat mimetics†
Received
1st April 2022
, Accepted 27th May 2022
First published on 7th June 2022
Abstract
Bacillus subtilis are important probiotic microbes currently formulated for delivery as spores, but their ability to germinate in the gut remains debatable. To optimize their application, cells should be delivered in their vegetative state, but the sensitivity of B. subtilis prevents this. Through the application of self-assembled metal-phenolic network (MPN) cellular coatings, B. subtilis are protected from lyophilization stresses. These MPNs are an important class of self-assembled materials comprised of polyphenols and metal ions, and the efficacy of MPN protection was found to be dependent on the MPN components used for assembly. Both the size of the polyphenol and stability of the metal-phenol coordination were important factors that influenced their cellular protection; the smallest polyphenol, gallic acid, and the most stable chelated ion, FeIII, were found to provide the highest level of protection. Further, delivery to the gut involves exposure to acidic conditions in the form of stomach acid and intestinal fluid. MPN coatings rapidly disassemble upon mild acid treatment but were found to protect B. subtilis from the negative impacts of the acid. Overall, optimized MPNs were found to protect vegetative B. subtilis cells from lyophilization stress and enable a more complete understanding of the role of each component in MPNs.
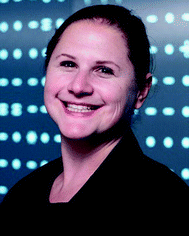
Ariel L. Furst
| Ariel L. Furst is the Raymond (1921) & Helen St. Laurent Career Development Professor of Chemical Engineering at MIT. Her lab works at the intersection of biological and chemical engineering to develop low-cost technologies to improve human and environmental health in low-resource settings. She received her PhD from CalTech working with Prof. Jacqueline K. Barton and was then an A. O. Beckman Postdoctoral Fellow with Prof. Matthew Francis at UC, Berkeley. Prof. Furst was a speaker at the ACS Sensors Young Investigator Symposium in 2020 and an MIT Future Founders Prize Finalist in 2021. In addition to her research, she is passionate about increasing participation of underrepresented groups in engineering. |
Introduction
Metal-phenolic networks (MPNs) are a promising class of materials for a variety of human health applications, ranging from contrast agents and drug delivery vehicles to sources of both prokaryotic and eukaryotic cell protection.1–3 Broadly, these materials are comprised of metal ions and polyphenols that self-assemble through coordination-based interactions. The diversity of potential applications is tied to the ease of formation of MPNs, allowing for convenient and simple addition to a variety of substrates.4–7
Specifically, there has recently been growing interest in the protection of beneficial microbes as both biotherapeutics and agricultural additives.8,9 However, in many cases, non-model microbes remain challenging to produce at scale due to processing stresses. Traditional lyophilization methods can lead to less than 0.1% cell survival for delicate microbes, many of which are promising candidates for biotherapeutics.10,11 We recently reported the protection of Bacterioides thetaiotaomicron, an important potential living biotherapeutic, from lyophilization and subsequent aerobic exposure using iron-containing MPNs.12 Others have reported the protection of live Escherichia coli from UV exposure following MPN coating.13 These results are encouraging for MPN suitability as a general protective coating for microbes. The achievement of relatively high post-processing viability will allow the development and stable manufacturing of exciting new microorganisms.14
MPNs can be generated from many different polyphenols and metal ions, but the majority of work with these systems has centered on the application of tannic acid (TA) and iron as MPN components.2 Yet, MPNs derived from alternative polyphenols and metal ions have significantly variable physicochemical properties that could be beneficial for different applications and may offer different protective properties based on the microbial strain employed. Thus, when designing these systems for specific strains and production stressors, the physical characteristics of the MPNs are important to consider. The most common mechanical metric reported is the Young's modulus, generally acquired through atomic force microscopy (AFM). The Young's modulus has been found to heavily depend on the molecular weight of the polyphenols and the pH of the buffer used; alkaline buffers and polyphenols with lower molecular weights lead to higher Young's modulus.15 MPN network size has additionally been shown to be dependent on the ionic strength of the assembly solution: increasing ionic strength results in thicker and rougher iron-TA films, measured by scanning electron microscopy (SEM).16 This controlled variation in the physical properties of MPNs based on variation of the metal ion and assembly solution is anticipated to be critical for their efficacy in microbial protection.
Our initial studies centered on the gram-negative, facultative anaerobe B. thetaiotaomicron that is challenging to manufacture.17,18 We reported the evaluation of three polyphenols (tannic acid (TA), epigallocatechin gallate (EGCG), and gallic acid (GA)) combined with FeIII to generate MPNs on living microbes to protect them from harsh processing stressors, including oxygen exposure and lyophilization.12 Our results demonstrated that the components used to form MPNs impacted the resultant protection afforded by the coatings. Bacillus subtilis, in contrast, are gram-positive and spore forming, enabling their production and delivery through sporulation.19
The spores of these microbes are highly tolerant to environmental stressors, but spores are dormant. Thus, there has been significant debate over the efficacy of spore-based probiotics including B. subtilis because germination must occur in the gastrointestinal (GI) tract for most host benefits. Though Bacillus spores do yield a host immune response, efficacy as a probiotic is linked to their germination and conversion to active, vegetative cells.20 Studies have shown Bacillus strains to be capable of germination in the human gut, but the sample size was small and the data is recent.21 Thus, we sought to employ MPN coatings as “synthetic spore coats” on non-sporulated cells to enable their rapid recovery in the GI tract. The importance of a healthy gut microbiome and the negative health effects of an unbalanced microbiome are well known, necessitating improved probiotics for more effective gut implantation.14
Surprisingly, we observed that for B. subtilis preservation, the canonical MPN comprised of TA and FeIII was not the optimal coating. Thus, we evaluated the impact of varying the polyphenol and the metal ion on the cellular protection effects of MPNs and developed an optimized coating recipe to protect B. subtilis from the freeze-drying process. We demonstrate that MPNs are readily self-assembled on silica bead substrates using different metal ions (FeIII, MnII, ZnII, and AlIII) and phenols (TA, EGCG and GA) for spectroscopic characterization. The coating process was found to be biocompatible and showed negligible adverse effects on microbial viability. Furthermore, FeIII-TA- and FeIII-GA-coated B. subtilis in cryoprotectant-free buffer showed faster recovery following lyophilization. Remarkably, after a simple L-ascorbic acid treatment (which mimics the pH of the GI tract), MPN-coated B. subtilis reached exponential phase after incubation for 16 h, whereas no growth was observed for the uncoated control. Overall, our results demonstrate that MPN coatings protect different microbes from lyophilization stresses, but the components of these coatings must be optimized based on the strain of interest. Specifically, we have shown the protection of non-sporulated B. subtilis during processing with MPN components different from the conventional composition as well as the ready subsequent removal of the coating in a GI environment.
Results and discussion
Spectroscopic characterization of MPN assemblies
The radii of metal ions, size of phenols, and chelation strength have all been reported to contribute to the difference in stiffness of MPNs.15 Thus, by simply mixing a series of metal ions and polyphenols, we could gain access to a library of MPN coatings with tunable mechanical properties.16,22 Biocompatible and biorelevant metal ions (FeIII, MnII, ZnII, and AlIII) and phenols (TA, EGCG, and GA) were selected for this evaluation (Fig. 1). In addition to the structure of the phenols and the valency of the metal ions, the pH of the coordination solution is also important for MPN formation, as alkaline conditions are necessary for effective chelation between the metal ion and hydroxyl groups.3 To evaluate MPN formation using various metals and polyphenols, solutions containing each were mixed. An equal volume of 3-morpholinopropane-1-sulfonic acid (MOPS) buffer (20 mM, pH 7.5) was added to the pre-complexed MPN solution, with a final MOPS concentration of 10 mM when in solution with the MPNs. Interestingly, upon addition of the buffer, a weak ligand-to-metal charge transfer (LMCT) band between 400 nm to 700 nm was observed in the UV-Vis spectrum, indicating the formation of the MPN complex (Fig. 1). These complexes are known to form under a variety of conditions and on diverse substrates due simply to the presence of a nucleation site and the correct pH.16,23–25
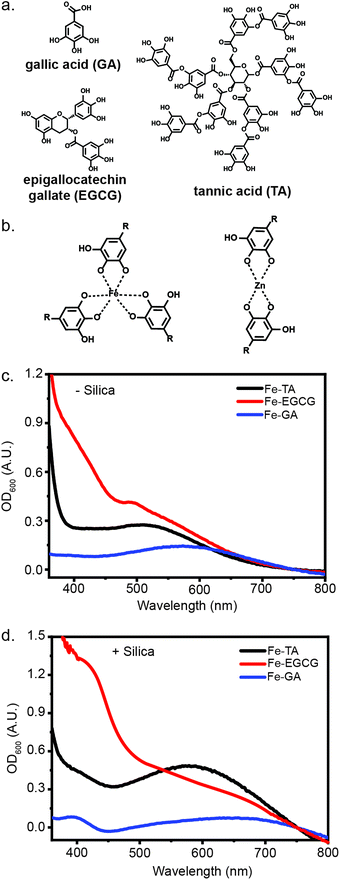 |
| Fig. 1 Abiotic characterization of metal-phenolic networks (MPNs). (a) Polyphenol components used in the MPN assemblies. (b) Example chelation of metal ions (FeIII and ZnII) to polyphenols. (c) UV-Vis absorbance of FeIII-TA, FeIII-GA, and FeIII-EGCG complexes in MOPS buffer (10 mM, pH 7.5). (d) FeIII-TA, FeIII-GA, and FeIII-EGCG complexes formed on the surface of silica particles (1 mg mL−1). | |
To evaluate the impact of micron-scale substrates for MPN formation, we evaluated the encapsulation of negatively-charged, micron-scale silica particles. Excitingly, upon addition of silica to the MPN solution, followed by pH adjustment, stronger absorbance bands were observed, indicating the deposition of MPNs on silica particles (Fig. 1). Some metal-phenol pairs (FeIII-TA, FeIII-EGCG, FeIII-GA, AlIII-EGCG and ZnII-EGCG) yielded strong LMCT bands, while others (ZnII-TA, ZnII-GA, MnII-GA) featured weaker bands, highlighting the differences in d–d transition strength from the metal and the polyphenol (Fig. S1, ESI†).26 Based on previous research into the physical differences between coatings derived from different metal ions and polyphenols combined with the spectroscopic characterization, these assemblies differ significantly in their ligand–metal coordination.26,27 We therefore hypothesized that these differences could impact cellular protection capabilities.
Microbial coating and subsequent viability assessment
To apply MPNs as protective agents, these coatings must readily form on microbial substrates with the same ease as the silica particles. Following our established protocol, FeIII-TA assemblies were readily deposited on the surface of B. subtilis. Surface coating was confirmed through observation of the metals on the surface of the bacteria by transmission electron microscopy (TEM) (Fig. 2(b)). As can be seen in the image of the uncoated cells, the edges of the uncoated and unstained cells appear smooth and ill-defined. In contrast, the well-defined surface of the MPN-coated cells is visible due to the presence of the metal ions. Following confirmation of coating formation on the cells, we evaluated the impact of the MPNs on cell growth and viability. Bacterial growth was monitored by optical density measurements at 600 nm (OD600), and a delay of 15 hours was observed for the MPN-coated cells to reach exponential phase as compared to the uncoated cells (MPN-coated: 20 hours vs. uncoated controls: 5 hours) (Fig. S2, ESI†). However, cell viability, which was assessed by live/dead staining coupled with fluorescence microscopy, showed minimal bacterial death for MPN-coated B. subtilis (85% viable), as compared to the uncoated control (92% viable) (Fig. 2(a)). Thus, we believe the lag in reaching exponential phase for the coated cells is due to the rigidity of the coating inhibiting cell division, rather than the MPNs impacting viability.
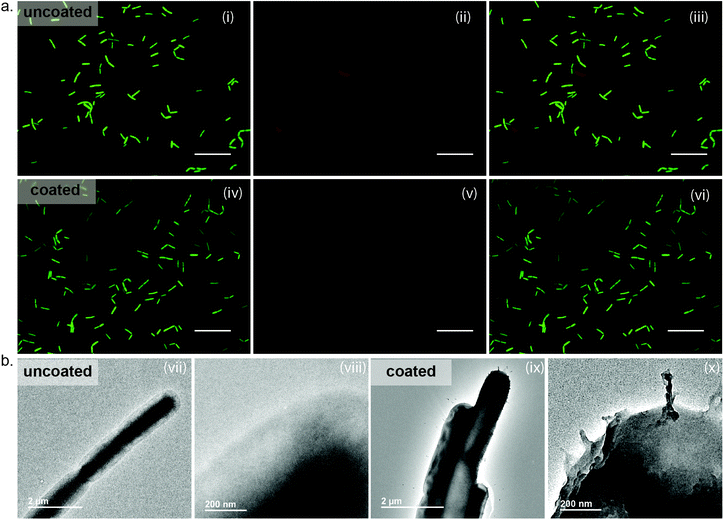 |
| Fig. 2 Fluorescence and TEM images of encapsulated B. subtilis. (a) Cells were treated with a live/dead staining kit. Shown are fluorescence images of (i)–(iii) uncoated and (iv)–(vi) FeIII-TA-coated B. subtilis in the FITC channel (SYTO-9), and TxRed channel (propidium iodide) and the merged images. Scale bars indicate 20 μm. (b) TEM images of (vii), (viii) uncoated and (ix), (x) FeIII-TA-coated B. subtilis. | |
Importantly, we further observed that a stable MPN assembly is critical for protection. Without the addition of MOPS buffer at a slightly alkaline pH, which serves to ensure chelation of the polyphenols to the metal ions and to rigidify the MPN capsule, a significant loss in the viability of MPN-coated B. subtilis (54% viable) was shown (Fig. S3, ESI†). This significant decrease in viability in the absence of MOPS buffer addition is attributed to the protonated polyphenols and unchelated metal ions in solution. Indeed, tannic acid has demonstrated inhibitory effects on the growth of some microbes.28 However, a stable MPN assembly under neutral pH yielded cells with equivalent viability to uncoated cells. Though simply coating cells is a first step in the development of non-sporulated B. subtilis for probiotics, lyophilization is necessary for formulation and delivery of these microbes to the gut. Thus, we next investigated the impact of lyophilization on B. subtilis viability.
Role of the phenol in MPN microbial protection
Previously, we discovered that FeIII-TA MPNs protected living microbes (E. coli and B. thetaiotaomicron) from stresses caused by the freezing and lyophilization processes, significantly increasing their viability post-reconstitution. We hypothesized that this protection could be extended to B. subtilis. We previously observed the most significant protection with TA-containing MPNs. We therefore began our evaluations of the impact of MPN coating on post-lyophilization viability of B. subtilis with FeIII-TA MPNs. FeIII-TA-coated B. subtilis were flash-frozen and lyophilized in one of four different buffers: phosphate citrate (PC) or phosphate buffered saline (PB), either in the absence of or presence of 0.1 M trehalose (cryoprotectant-PCT or PBT, respectively). OD600 was monitored post-reconstitution in the nutrient broth medium at 30 °C (Fig. 3(a)). Surprisingly, the protection of cells with this coating was found to be highly buffer-dependent. OD600 values after 48 hours of post-reconstitution growth was fastest when lyophilized in PCT, with high variability in growth from lyophilization in other buffered conditions (Fig. 3(a)). This result indicates that the protective ability of the MPNs is somewhat strain dependent. Thus, we sought to investigate whether altering the components of the MPN impacted post-lyophilization viability. We expanded the polyphenols investigated to include EGCG and GA. No significant difference was observed in the post-reconstitution growth of FeIII-EGCG-coated B. subtilis as compared to the uncoated controls. Yet interestingly, FeIII-GA-coated B. subtilis demonstrated higher OD600 values post-reconstitution as compared to the uncoated controls in both buffers (PBT and PCT).
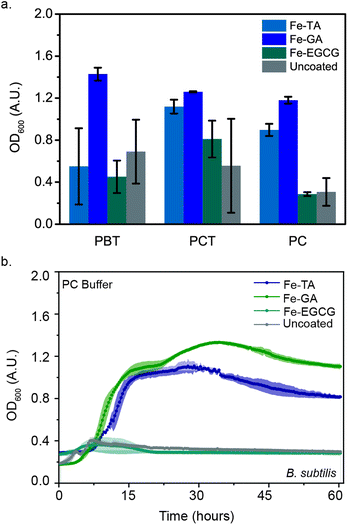 |
| Fig. 3 FeIII-phenol encapsulation protects microbes during lyophilization. (a) OD600 of lyophilized B. subtilis following 48 h of growth in the presence and absence of FeIII-phenols and cryoprotectants (0.1 M) (PC, phosphate citrate buffer; PB, phosphate-buffered saline; T, trehalose). (b) Growth curves of FeIII-phenols-coated and uncoated B. subtilis following lyophilization without cryoprotectants. Error bars and shaded region represent SD for n = 3 biological replicates. | |
Though trehalose is canonically used to maintain biomolecule stability during lyophilization, the addition of this molecule is not ideal for the eventual formulation of the cells for oral delivery.29–31 Thus, we additionally investigated the impact of MPN coating on protection of B. subtilis following lyophilization in the absence of trehalose. Remarkably, FeIII-GA- and FeIII-TA-coated B. subtilis in cryoprotectant-free buffer (PC) demonstrated 4-fold and 3-fold increases in OD600, respectively. Under the same conditions, FeIII-GA- and FeIII-TA-coated B. subtilis reach exponential growth within 8 hours, whereas no growth was observed for either FeIII-EGCG-coated microbes or the uncoated controls (Fig. 3(b)). These results demonstrate the ability of iron-containing MPNs to provide protection for B. subtilis post-lyophilization even in absence of cryoprotectant. Furthermore, this protection was found to be phenol-dependent, where the efficacy decreased in the order of GA > TA > EGCG. It was previously reported that the size of the phenol ligand affects the Young's modulus (EY) of MPNs, with smaller ligands leading to higher moduli.15 Thus, we anticipate that the higher protection afforded by FeIII-GA complexes could be attributed to the modulus of this MPN. Meanwhile, our results further demonstrate how critical the lyophilization buffers are in stabilizing microbes during the freeze-drying process. Because of the significant impact that varying the polyphenol was found to have on post-lyophilization stability, we further sought to investigate the impact of varying the metal ion in the MPNs.
Role of the metal in MPN-based microbe protection
Though 18 metal ions have been shown in the literature to form MPNs with polyphenols, only biocompatible metal ions were investigated in this study, as the eventual goal of coating B. subtilis is to enable their oral delivery.26 Specifically, we focused on four ions: FeIII, MnII, ZnII, and AlIII. Because GA demonstrated the highest level of microbial protection of the three polyphenols investigated, the four metal ions were tested as components of MPNs formed with GA. FeIII-GA, MnII-GA, ZnII-GA, and AlIII-GA-coated B. subtilis were flash-frozen and lyophilized in either PBT or PCT. OD600 was monitored after reconstitution in nutrient broth medium (Fig. 4(a)). No significant difference in OD600 values after 48 h was observed between FeIII-GA, MnII-GA, and AlIII-GA-coated B. subtilis as compared to the uncoated control. Moreover, no growth was observed for ZnII-GA coated microbes. We attribute this to the protective abilities of the trehalose in the buffer because, upon addition of cryoprotectant-free PC buffer, no growth was observed after 48 h for MnII-GA-coated B. subtilis or the uncoated controls. However, growth was observed for cells coated in MPNs formed with the three other metal ions. Interestingly, the delay in reaching exponential growth (teg) decreased in the order of FeIII < AlIII < ZnII, revealing that metal ions also impact the efficacy of MPN cellular protection (Fig. S5, ESI†). This trend is consistent with previous work showing that trivalent metal ions increased the network size of MPN assemblies as compared to those using divalent metal ions with same phenol. Further, the increased recovery rate with FeIII-GA as compared to AlIII-GA-coated B. subtilis is consistent with the strength of ligand chelation and the size of the metal ionic radius.32,33 Taken together, these results confirm that both the polyphenol and the metal ion used to form MPNs impact the ability of the MPNs to protect microbes following lyophilization. Further, as compared to our previously-reported results with B. thetaiotaomicron, the coating efficacy seems to be strain-dependent, with optimal MPNs varying based on the microbe being evaluated.
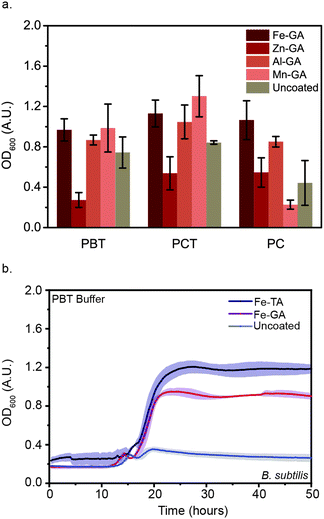 |
| Fig. 4 Metal-GA encapsulation protect microbes during lyophilization. (a) OD600 of lyophilized B. subtilis following 48 h of growth in the presence and absence of metal-GA and cryoprotectants (0.1 M) (PC, phosphate citrate buffer; PB, phosphate-buffered saline). (b) Growth curves of FeIII-TA, FeIII-GA-coated and uncoated lyophilized B. subtilis following dilute L-ascorbic acid (AA) treatment (5 min). Error bars and shaded region represent SD for n = 3 biological replicates. | |
Mild acid treatment leads to faster recovery
Under acidic conditions, hydroxyl groups on the polyphenols in the MPN complexes are protonated, disrupting chelation and leading to disassembly.34 For oral delivery applications, this pH-dependent degradation is important, as it provides an inherent on-demand disassembly for the cells upon their exposure to stomach acid and intestinal fluid. Though uncoated B. subtilis demonstrated faster recovery than MPN-coated cells post-reconstitution in nutrient broth with PBT or PCT (Fig. S4, S5 and S7, ESI†), this appears to be due to the inherent lag in growth consistently observed with MPN-coated B. subtilis (Fig S2, ESI†). This hypothesis is supported by the rapid growth of lyophilized MPN-coated B. subtilis when cells are reconstituted in 10 mM L-ascorbic acid solution for 5 min prior to transferal to nutrient broth. The pH of the L-ascorbic acid solution used in this study was measured to be pH = 2.5, which is within the range of the pH of stomach acid (pH = 1.5–3.5). Removal of the coating prior to growth studies decreased the delay time to exponential growth (teg) of FeIII-GA and FeIII-TA-coated cells dramatically. Further, microbial survival following acid treatment was only observed with MPN-coated cells; no growth after 48 hours was observed with the uncoated controls (Fig. 4). These results further demonstrate the utility of MPN protective coatings for oral delivery of microbes, as these cells will necessarily be exposed to acidic conditions in the stomach and gut.
Conclusions
MPNs are an important class of self-assembled materials comprised of polyphenols and metal ions that had previously been shown to protect microbes of interest as next-generation biotherapeutics during lyophilization and processing. However, the universality of these coatings for microbial protection and delivery had not been established. Here, we investigated the protection of B. subtilis, an important probiotic strain, from processing stressors during lyophilization. Surprisingly, the efficacy of protection was found to be dependent on the metal and polyphenol used for assembly. Both the size of the polyphenol and stability of the metal-phenol coordination were important factors that influenced their cellular protection; the smallest polyphenol, GA, and the most stable chelated ion, FeIII, were found to provide the highest level of protection. Further, for probiotics and living biotherapeutics, delivery to the gut involves exposure to acidic conditions in the form of stomach acid and intestinal fluid. MPN coatings rapidly disassembled upon mild acid treatment but protected the B. subtilis from the negative impacts of the acid. Overall, B. subtilis is an important probiotic strain currently formulated for delivery as spores, but their ability to germinate in the gut remains debatable. Thus, the ability to deliver viable B. subtilis cells directly is anticipated to improve the efficacy of these strains and serves as an additional study to better understand role of each component in MPNs.
Conflicts of interest
We have filed a provisional patent based on this work.
Acknowledgements
We acknowledge the MIT-Deshpande Center for funding of this work. G. F. acknowledges the MIT Chemical Engineering Communication Lab for partially funding of this work. P. W.'s and B. B.'s work was funded through the Undergraduate Research Opportunities Program (UROP) at MIT.
Notes and references
- W. Youn, J. Y. Kim, J. Park, N. Kim, H. Choi, H. Cho and I. S. Choi, Single-cell nanoencapsulation: From passive to active shells, Adv. Mater., 2020, 32(35), 1907001, DOI:10.1002/adma.201907001.
- Z. Guo, W. Xie, J. Lu, X. Guo, J. Xu, W. Xu, Y. Chi, N. Takuya, H. Wu and L. Zhao, Tannic acid-based metal phenolic networks for bio-applications: A review, J. Mater. Chem. B, 2021, 9(20), 4098–4110, 10.1039/D1TB00383F.
- Z. Zhang, L. Xie, Y. Ju and Y. Dai, Recent advances in metal-phenolic networks for cancer theranostics, Small, 2021, 17(43), 1–33, DOI:10.1002/smll.202100314.
- H. Geng, Q.-Z. Zhong, J. Li, Z. Lin, J. Cui, F. Caruso and J. Hao, Metal ion-directed functional metal–phenolic materials, Chem. Rev., 2022 DOI:10.1021/acs.chemrev.1c01042.
- D. Wu, J. Zhou, M. N. Creyer, W. Yim, Z. Chen, P. B. Messersmith and J. V. Jokerst, Phenolic-enabled nanotechnology: Versatile particle engineering for biomedicine., Chem. Soc. Rev., 2021, 50(7), 4432–4483, 10.1039/D0CS00908C.
- G. Fan, J. Cottet, M. R. Rodriguez-Otero, P. Wasuwanich and A. L. Furst, Metal–phenolic networks as versatile coating materials for biomedical applications., ACS Appl. Bio Mater., 2022 DOI:10.1021/acsabm.2c00136.
- Q. Dai, H. Geng, Q. Yu, J. Hao and J. Cui, Polyphenol-based particles for theranostics., Theranostics, 2019, 9(11), 3170–3190, DOI:10.7150/thno.31847.
- H. J. Wu and E. Wu, The role of gut microbiota in immune homeostasis and autoimmunity, Gut Microbes, 2012, 3(1), 4–14, DOI:10.4161/gmic.19320.
- N. Vassilev, M. Vassileva, V. Martos, L. F. del Moral, J. Kowalska, B. Tylkowski and E. Malusá, Formulation of microbial inoculants by encapsulation in natural polysaccharides: Focus on beneficial properties of carrier additives and derivatives., Front. Plant Sci., 2020, 11, DOI:10.3389/fpls.2020.00270.
- Y. Miyamoto-Shinohara, T. Imaizumi, J. Sukenobe, Y. Murakami, S. Kawamura and Y. Komatsu, Survival rate of microbes after freeze-drying and long-term storage., Cryobiology, 2000, 41(3), 251–255, DOI:10.1006/cryo.2000.2282.
- E. B. Minelli and A. Benini, Relationship between number of bacteria and their probiotic effects, Microb. Ecol. Health Dis., 2008, 20(4), 180–183, DOI:10.1080/08910600802408095.
- G. Fan, P. Wasuwanich, M. R. Rodriguez-Otero and A. L. Furst, Protection of anaerobic microbes from processing stressors using metal–phenolic networks., J. Am. Chem. Soc., 2022, 144(6), 2438–2443, DOI:10.1021/jacs.1c09018.
- W. Li, W. Bing, S. Huang, J. Ren and X. Qu, Mussel byssus-like reversible metal-chelated supramolecular complex used for dynamic cellular surface engineering and imaging., Adv. Funct. Mater., 2015, 25(24), 3775–3784, DOI:10.1002/adfm.201500039.
- A. Terpou, A. Papadaki, I. K. Lappa, V. Kachrimanidou, L. A. Bosnea and N. Kopsahelis, Probiotics in food systems: Significance and emerging strategies towards improved viability and delivery of enhanced beneficial value., Nutrients, 2019, 11, 1591, DOI:10.3390/nu11071591.
- G. Yun, J. J. Richardson, M. Biviano and F. Caruso, Tuning the mechanical behavior of metal-phenolic networks through building block composition., ACS Appl. Mater. Interfaces, 2019, 11(6), 6404–6410, DOI:10.1021/acsami.8b19988.
- H. Ejima, J. J. Richardson, K. Liang, J. P. Best, M. P. van Koeverden, G. K. Such, J. Cui and F. Caruso, One-step assembly of coordination complexes for versatile film and particle engineering., Science, 2013, 341(6142), 154–157 CrossRef CAS PubMed.
- H. M. Wexler, Bacteroides: The good, the bad, and the nitty–gritty., Clin. Microbiol. Rev., 2007, 20(4), 593–621, DOI:10.1128/CMR.00008-07.
- H. J. Flint, K. P. Scott, S. H. Duncan, P. Louis and E. Forano, Microbial degradation of complex carbohydrates in the gut., Gut Microbes, 2012, 3(4), 289–306, DOI:10.4161/gmic.19897.
- Z. Xiaopei, A.-D. Amal, H. Myer, S. Peter and L. Jiahe, Applications of bacillus subtilis spores in biotechnology and advanced materials, Appl. Environ. Microbiol., 2022, 86(17), e01096-20, DOI:10.1128/AEM.01096-20.
- L. Rhayat, M. Maresca, C. Nicoletti, J. Perrier, K. S. Brinch, S. Christian, E. Devillard and E. Eckhardt, Effect of bacillus subtilis strains on intestinal barrier function and inflammatory response., Front. Immunol., 2019, 10, DOI:10.3389/fimmu.2019.00564.
- J. Colom, D. Freitas, A. Simon, A. Brodkorb, M. Buckley, J. Deaton and A. M. Winger, Presence and germination of the probiotic bacillus subtilis de111® in the human small intestinal tract: A randomized, crossover, double-blind, and placebo-controlled study., Front. Microbiol., 2021, 12, DOI:10.3389/fmicb.2021.715863.
- O. Mazaheri, M. S. Alivand, A. Zavabeti, S. Spoljaric, S. Pan, D. Chen, F. Caruso, H. C. Suter and K. A. Mumford, Assembly of metal–phenolic networks on water-soluble substrates in nonaqueous media, Adv. Funct. Mater., 2022, 2111942, DOI:10.1002/adfm.202111942.
- J. Meng, X. Liu, C. Niu, Q. Pang, J. Li, F. Liu, Z. Liu and L. Mai, Advances in metal–organic framework coatings: Versatile synthesis and broad applications., Chem. Soc. Rev., 2020, 49(10), 3142–3186, 10.1039/c9cs00806c.
- H. Geng, L. Zhuang, M. Li, H. Liu, F. Caruso, J. Hao and J. Cui, Interfacial assembly of metal–phenolic networks for hair dyeing., ACS Appl. Mater. Interfaces, 2020, 12(26), 29826–29834, DOI:10.1021/acsami.0c06928.
- Q. Z. Zhong, S. Pan, M. A. Rahim, G. Yun, J. Li, Y. Ju, Z. Lin, Y. Han, Y. Ma and J. J. Richardson,
et al., Spray assembly of metal-phenolic networks: Formation, growth, and applications., ACS Appl. Mater. Interfaces, 2018, 10(39), 33721–33729, DOI:10.1021/acsami.8b13589.
- J. Guo, Y. Ping, H. Ejima, K. Alt, M. Meissner, J. J. Richardson, Y. Yan, K. Peter, D. von Elverfeldt and C. E. Hagemeyer,
et al., Engineering multifunctional capsules through the assembly of metal-phenolic networks, Angew. Chem., Int. Ed., 2014, 53(22), 5546–5551 CrossRef CAS PubMed.
- J. Guo, J. J. Richardson, Q. A. Besford, A. J. Christofferson, Y. Dai, C. W. Ong, B. L. Tardy, K. Liang, G. H. Choi and J. Cui,
et al., Influence of ionic strength on the deposition of metal-phenolic networks., Langmuir, 2017, 33(40), 10616–10622, DOI:10.1021/acs.langmuir.7b02692.
- N. Sahiner, S. Sagbas, M. Sahiner, C. Silan, N. Aktas and M. Turk, Biocompatible and biodegradable poly(tannic acid) hydrogel with antimicrobial and antioxidant properties., Int. J. Biol. Macromol., 2016, 82, 150–159, DOI:10.1016/j.ijbiomac.2015.10.057.
- S. B. Leslie, E. Israeli, B. Lighthart, J. H. Crowe and L. M. Crowe, Trehalose and sucrose protect both membranes and proteins in intact bacteria during drying., Appl. Environ. Microbiol., 1995, 61(10), 3592–3597, DOI:10.1128/aem.61.10.3592-3597.1995.
- L. M. Crowe, C. Womersley, J. H. Crowe, D. Reid, L. Appel and A. Rudolph, Prevention of fusion and leakage in freeze-dried liposomes by carbohydrates, Biochim. Biophys. Acta, Biomembr., 1986, 861(C), 131–140, DOI:10.1016/0005-2736(86)90411-6.
- C. A. Morgan, N. Herman, P. A. White and G. Vesey, Preservation of micro–organisms by drying; a review, J. Microbiol. Methods, 2006, 183–193, DOI:10.1016/j.mimet.2006.02.017.
- E. Degtyar, M. J. Harrington, Y. Politi and P. Fratzl, The mechanical role of metal ions in biogenic protein-based materials, Angew. Chem., Int. Ed., 2014, 53(45), 12026–12044, DOI:10.1002/anie.201404272.
- R. C. Hider and X. Kong, Chemistry and Biology of Siderophores., Nat. Prod. Rep., 2010, 27(5), 637–657, 10.1039/B906679A.
- J. M. Shin, G. H. Choi, S. H. Song, H. Ko, E. S. Lee, J. A. Lee, P. J. Yoo and J. H. Park, Metal-phenolic network-coated hyaluronic acid nanoparticles for ph-responsive drug delivery., Pharmaceutics, 2019, 11(12), 1–11, DOI:10.3390/pharmaceutics11120636.
Footnotes |
† Electronic supplementary information (ESI) available: Additional experimental protocol details and supplemental figures. See DOI: https://doi.org/10.1039/d2tb00717g |
‡ These authors contributed equally. |
|
This journal is © The Royal Society of Chemistry 2022 |
Click here to see how this site uses Cookies. View our privacy policy here.