A novel hypocrellin-based assembly for sonodynamic therapy against glioblastoma†
Received
30th August 2021
, Accepted 10th November 2021
First published on 16th November 2021
Abstract
The non-invasive treatment of glioblastoma (GBM) is of great significance and can greatly reduce the complications of craniotomy. Sonodynamic therapy (SDT) is an emerging tumor therapeutic strategy that overcomes some fatal flaws of photodynamic therapy (PDT). Different from PDT, SDT has deep tissue penetration and can be applied in the non-invasive treatment of deep-seated tumors. However, effective sonosensitizers that can be used for SDT of GBM are still very rare. Herein, we have prepared a suitable assembly based on a hypocrellin derivative (CTHB) with good biocompatibility. Excitedly, the hypocrellin-based assembly (CTHB NPs) can effectively produce reactive oxygen species under ultrasound stimulation. The inherent fluorescence and photoacoustic imaging characteristics of the CTHB NPs are conducive to the precise positioning of the tumors. It has been proved both in subcutaneous and in intracranial tumor models that CTHB NPs can be used as an effective sonosensitizer to inhibit tumor growth under ultrasound irradiation. This hypocrellin-based assembly has a good clinical prospect in the non-invasive treatment of GBM.
1. Introduction
Glioblastoma (GBM) is one of the most invasive tumors in the skull. Craniotomy surgery is very risky to patients, and has a high recurrence rate and mortality rate.1–4 The combination of surgery, radiotherapy and chemotherapy has made some progress in the treatment of GBM. However, there are still huge challenges in the non-invasive treatment of GBM. Although photodynamic therapy (PDT) has made encouraging progress in tumor treatment as a non-invasive treatment mode, lasers still cannot break through the limitation of tissue penetration depth.5–7 Recently, sonodynamic therapy (SDT) has been rapidly developed as a new technology for noninvasive treatment of cancers, in which SDT activates a sonosensitizer to produce reactive oxygen species under low-intensity ultrasound (US) irradiation, inducing cell apoptosis or necrosis.8–14 Ultrasound with the appropriate frequency can accurately activate the sonosensitizer enriched in tumor sites without damage to normal tissues. SDT has a high tissue penetration and can be applied in noninvasive treatment of deep-seated tumors, which is difficult to achieve in PDT.15–20 Therefore, compared with PDT, SDT is a promising noninvasive treatment for deep intracranial tumors.
Porphyrin and its derivatives, as the most widely used sonosensitizers in SDT, have a large π-electron conjugated system.21–23 However, these porphyrin molecules usually have low chemical and biological stability, and their long-term retention in the body may cause side effects such as skin phototoxicity.24–27 As a result, there is a high demand to develop new sonosensitizers for efficient SDT. Alternatively, hypocrellin, as a natural photosensitizer extracted from hypocrella bambusae, has been widely applied in highly efficient photodynamic therapy.28–31 Hypocrellin and its derivatives have the essential benefits of high singlet oxygen yield, good biocompatibility, short residence time and fast metabolism in vivo.32–36 Although hypocrellin has been receiving considerable attention for photodynamic therapy in recent years, hypocrellin used for SDT is still very rare. For example, Wang et al. used hypocrellin B (HB) for SDT of hepatocellular carcinoma cells because intracellular reactive oxygen species (ROS) were significantly increased after ultrasound, inducing apoptosis of cells.37 Jia et al. proved that HB could be used for SDT of breast cancer cells, and the cell apoptosis caused by ultrasound was partly involved in the caspase pathway.38 Liu et al. reported that HB-triggered SDT could synergistically increase DOX-induced SGC-7901cell/ADR cell apoptosis.39 Zheng et al. synthesized a 1,2-diaminopropane-modified hypocrellin derivative (APHB),32 which was further self-assembled with polyethylene glycol-poly(lactic-co-glycolic acid) (PEG–PLGA) to prepare APHB NPs. APHB NPs could be used for near-infrared fluorescence (FL) imaging of tumors and SDT of deep-seated tumors. However, so far there are still no hypocrellin and its derivatives used in SDT of GBM. In addition, hypocrellin derivatives with longer absorption wavelength can also reduce skin phototoxicity caused by sunlight. Therefore, the development of sonotheranostic agents based on the hypocrellin derivatives for SDT in deep intracranial tumors has good application prospects.
The photophysical properties of the HB skeleton change with the modification of different substituents. Compared with HB, the absorption and fluorescence spectra of 2-position modified hypocrellin derivatives or 4-/5-positions modified hypocrellin derivatives have a large red shift.33,40,41 In the case of the cysteamine modified hypocrellin derivative (referred to as AETHB), cysteamine was modified to the 4-/5-positions of the HB skeleton to form a cyclic derivative (Fig. 1a).33 In this work, both cysteamine modification in the 4-/5-positions and tryptophan modification in the 2-position of the HB skeleton were designed to obtain a novel cysteamine and tryptophan-modified hypocrellin derivative (referred to as CTHB). Compared with AETHB, the absorption and fluorescence spectra of CTHB were distinctly red-shifted. The maximum absorption wavelength of CTHB was red-shifted from 534 nm to 640 nm and the maximum emission wavelength was red-shifted from 710 nm to 800 nm. As we know, fluorescent diagnostic materials with longer emission can significantly reduce the autofluorescence of biological tissues and enhance the contrast between tumor and normal tissues. In addition, both cysteamine and tryptophan have good biocompatibility. To improve water solubility of CTHB, the biodegradable polyethylene glycol material (PEG–PLGA) was assembled with CTHB to prepare CTHB nanoparticles (referred to as CTHB NPs) (Scheme 1). The prepared CTHB NPs had a wide absorption spectrum in the range of 450–800 nm and an emission band peaked at 800 nm, which was convenient to realize FL and photoacoustic (PA) imaging because in the near-infrared region CTHB NPs further reduced the interference of biological autofluorescence. Under ultrasound irradiation, CTHB NPs could effectively generate 1O2 for SDT at the cellular and living levels. More importantly, CTHB NPs with suitable particle size and lipophilicity could effectively be enriched in intracranial tumors. The growth of intracranial tumors in mice was significantly inhibited with SDT. Therefore, CTHB NPs could be used in FL/PA dual-modality imaging-guided SDT and had good biocompatibility. This nano-sonotheranostic agent based on the hypocrellin derivative provides a new method for the accurate diagnosis and noninvasive treatment of GBM.
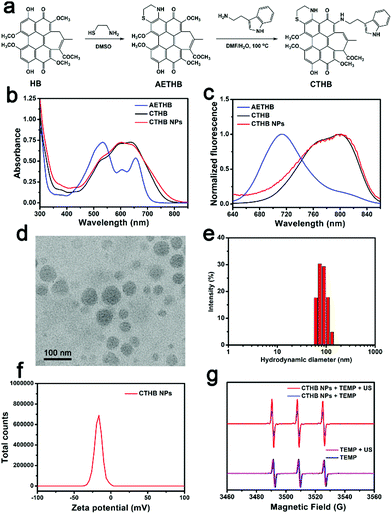 |
| Fig. 1 (a) The synthetic route of CTHB and CTHB NPs. (b) Absorption spectra of AETHB, CTHB and CTHB NPs. (c) Normalized FL spectra of AETHB, CTHB and CTHB NPs. (d) TEM image of CTHB NPs. (e) DLS analysis of CTHB NPs. (f) Zeta potential of CTHB NPs. (g) ESR signal of CTHB NPs and TEMP before and after ultrasound irradiation. | |
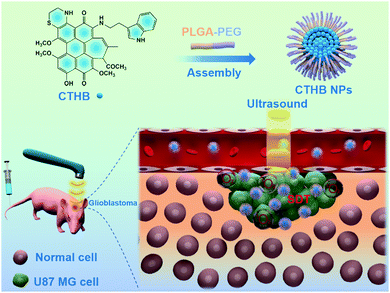 |
| Scheme 1 Schematic illustration of the preparation of CTHB NPs and their application in sonodynamic therapy for glioblastoma. | |
2. Results and discussion
2.1. Synthesis and characterization of CTHB and CTHB NPs
A cysteamine and tryptamine-modified hypocrellin derivative (CTHB) was synthesized (Fig. 1a) and characterized by 1H NMR, 13C NMR, and HR-MS. However, the lipophilicity of CTHB limits its further application in biological systems. To enhance the water solubility and biocompatibility of CTHB, amphiphilic biodegradable polyethylene glycol PEG–PLGA was used to encapsulate CTHB to prepare water-soluble CTHB NPs by a single emulsion method (Fig. 1a).42–44 The loading efficiency of CTHB in CTHB NPs was calculated to be about 9.1% by a standard curve of O.D. value at 640 nm (Y = 0.0253X–0.0059, R2 = 0.9999) (Fig. S1a and b, ESI†). CTHB NPs exhibited a broad absorption spectrum at 450–800 nm (Fig. 1b) and a maximum fluorescence peak at 800 nm (Fig. 1c), indicating that it could be used as a fluorescence and photoacoustic diagnostic reagent in the near infrared region. The transmission electron micrograph (TEM, Fig. 1d) showed that the prepared CTHB NPs had a particle size of approximately 50 nm. Furthermore, the average hydrated particle size of CTHB NPs suspended in water was about 85 nm according to the dynamic light scattering (DLS) analysis (Fig. 1e). The zeta potential of CTHB NPs was −17.1 mV (Fig. 1f). The suitable particle size and negative zeta potential of CTHB NPs are conducive to their effective enrichment in tumors through the enhanced permeability and retention (EPR) effect.45,46
Using 2,2,6,6-tetramethylpiperidine (TEMP) as the 1O2 capture agent, the characteristic of CTHB NPs to produce 1O2 under ultrasound irradiation was studied. As shown in Fig. 1g, after 5 min of ultrasound (frequency: 1 MHz, intensity: 0.8 W cm−2, duty cycle: 20%), an obvious characteristic signal of 1O2 was observed in the CTHB NPs + TEMP solution, while no 1O2 was generated in the TEMP only solution. This shows that CTHB NPs can effectively produce 1O2 after ultrasound irradiation, indicating that it can be used as an effective SDT agent.
2.2. Cellular uptake and sonodynamic therapy
In order to study the endocytosis ability of U87MG cells to CTHB NPs, U87MG cells were incubated with CTHB NPs (50 μg mL−1) for 6 h for fluorescence imaging. Cell fluorescence images showed that CTHB NPs could be effectively endocytosed by U87MG cells (Fig. 2a). In order to confirm the ability of CTHB NPs to generate ROS in cells under ultrasound irradiation, a 2′,7′-dichlorodihydrofluorescein (DCFH-DA) staining experiment was implemented (Fig. 2b). In the DCFH-DA only group or CTHB NPs only group, no green fluorescence was observed. However, in the CTHB NPs + DCFH-DA group, obvious green fluorescence was observed after ultrasound irradiation. It is concluded that CTHB NPs generate a large amount of ROS in the cells after ultrasound irradiation and they can be applied to intracellular SDT.
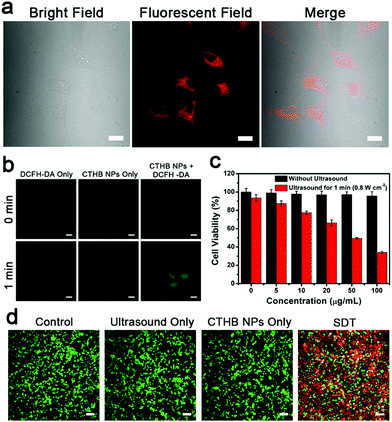 |
| Fig. 2 (a) Confocal images of CTHB NPs in U87MG cells. Scale bar: 20 μm. (b) ROS-generated images in U87MG cells with different treatments. Scale bar: 20 μm. (c) Cytotoxicity of CTHB NPs and cell survival rates with SDT. (d) Confocal images of the calcein AM/PI-costained U87MG cells with different treatments. Scale bar: 100 μm. | |
The standard 3-(4,5-dimethylthiazol-2-yl)-2, 5-diphenyl tetrazolium bromide (MTT) assays were used to evaluate the cytotoxicity of CTHB NPs on U87MG cells and the efficacy of SDT (Fig. 2c). U87MG cells were incubated with CTHB NPs (5–100 μg mL−1) for 24 h and the cell survival rates exceeded 95%, indicating that the CTHB NPs had low cytotoxicity and good biocompatibility. On the other hand, the intracellular SDT experiment was implemented. U87MG cells were incubated with CTHB NPs (5–100 μg mL−1) for 6 h with ultrasound for 1 min (frequency: 1 MHz, intensity: 0.8 W cm−2, duty cycle: 20%). It was found that cell survival rates gradually decreased with the increase of CTHB NPs. When the concentration of CTHB NPs was 100 μg mL−1, approximately 70% of the tumor cells were killed under ultrasound irradiation, indicating that CTHB NPs had a good SDT effect at the cellular level.
Next, the co-staining experiment of calcein AM (green) and propidium iodide (PI, red) further verified the efficacy of SDT of CTHB NPs. As shown in Fig. 2d, for the the cells treated with ultrasound only or incubated with CTHB NPs only, uniform green fluorescence was observed, indicating that these cells were still alive. After SDT, a large area of red fluorescence was observed, indicating that most cells were killed. These results further indicate that CTHB NPs have a good SDT efficiency.
2.3. PA imaging of subcutaneous tumors
Encouraged by the intracellular experimental results, PA imaging was used to study the enrichment ability of CTHB NPs in tumor sites. First, PA imaging of CTHB NPsin vitro was studied. As shown in Fig. S2 (ESI†), the PA intensity gradually increased with the amount of CTHB NPs, indicating that CTHB NPs could be used as a PA imaging diagnostic reagent. Next, PA images of CTHB NPs in the tumor sites at different time points (0, 2, 5, 8, and 24 h) were recorded after intravenous injection with CTHB NPs (100 μL, 1.0 mg mL−1). As shown in Fig. 3a, the obvious PA signal was detected in the tumor area, and the PA intensity gradually increased with time. The PA signal in the tumor area reached a maximum value at 5 h, and then gradually decreased. After injection of CTHB NPs for 24 h, the tumor and main organs of the mouse were harvested for ex vivo FL imaging (Fig. 3b and c). It was found that CTHB NPs are highly enriched in tumor sites and were mainly metabolized out of the body through the liver and kidneys. Therefore, CTHB NPs can be passively enriched in tumor sites through the EPR effect, and the best time for ultrasound treatment is 5 h after intravenous injection. The above experimental results show that CTHB NPs can be used for PA imaging-guided SDT.
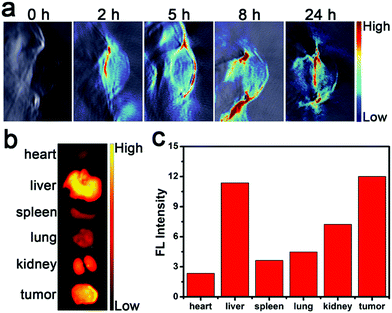 |
| Fig. 3 (a) In vivo PA imaging after i.v. injection of CTHB NPs after 0, 2, 5, 8 and 24 h. (b) FL images and (c) average FL intensities of the tumor and major organs 24 h after i.v. injection with CTHB NPs. | |
2.4. FL imaging of an intracranial tumor
In order to further evaluate the enrichment ability of CTHB NPs in the intracranial tumors, U87MG cells were implanted into the right brain of mice using a brain stereotaxic instrument to establish a mouse orthotopic glioblastoma model. Five hours after intravenous injection with CTHB NPs (100 μL, 1.0 mg mL−1), the intracranial tumor and main organs of the mouse were harvested for ex vivo FL imaging. It was found that the fluorescence intensity in the intracranial tumor was 2.6-fold higher than that in normal brain tissues. CTHB NPs could be effectively enriched in intracranial tumors because the CTHB NPs had suitable particle sizes and lipophilicity (Fig. 4a and b).47,48 As a result, CTHB NPs can be applied to the SDT of intracranial tumors.
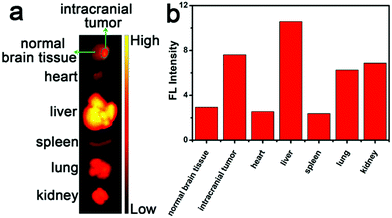 |
| Fig. 4 (a) FL images and (b) average FL intensities of intracranial tumor and major organs 5 h after i.v. injection with CTHB NPs. | |
Generally, nanoparticle sizes determine their biodistribution and pharmacokinetic characteristics in the body, such as circulatory half-life, macrophage uptake, clearance of the reticuloendothelial system, and tumor blood vessel permeability.49 For the drug delivery to brain tumors, the ideal size of nanoparticles is 20–70 nm.50,51 In addition, PEG-coated nanoparticles can avoid adhesion to the extracellular matrix and have a stronger penetration ability in brain tissue, and the enhanced lipophilicity will promote the blood–brain barrier (BBB) penetration of the nanoparticles.52–54 Because hypocrellin derivatives have strong lipophilicity inherently, surface-modified PEG can not only enhance their dispersion stability in water, but also avoid being adhered by proteins in the physiological environment. Therefore, CTHB NPs with suitable particle sizes (about 50 nm) and lipophilicity may be effectively enriched in the intracranial tumors.
2.5. Sonodynamic therapy of subcutaneous tumors
The SDT efficiency of CTHB NPs on subcutaneous U87MG tumors was studied. The tumor-bearing mice were randomly divided into 4 groups (5 mice in each group): (1) Control group; (2) PBS + ultrasound group; (3) CTHB NPs only group (100 μL, 1.0 mg mL−1); (4) CTHB NPs + ultrasound group (SDT group). After five hours for intravenous injection with CTHB NPs (100 μL, 1.0 mg mL−1), ultrasound irradiation (frequency: 1 MHz, intensity: 0.8 W cm−2, duty cycle: 20%, 5 min) of the tumors was conducted. As shown in Fig. 5a, tumors of mice treated with ultrasound only or injected with CTHB NPs only grew rapidly, and the growth rates were consistent with the control group, indicating that ultrasound only or CTHB NPs only could not effectively inhibit tumor growth. For the control group, the PBS + ultrasound group and CTHB NPs only group, the tumor volume of the mice increased to about 9-fold larger compared to the initial volume, while the tumor volume of the SDT group only has a 3-fold increase. Therefore, the tumor growth rate in the SDT group was slower than those in the three control groups, indicating that CTHB NPs had a superior therapeutic efficiency under ultrasound irradiation. During the treatment, the body weights of the mice in each group did not decrease significantly (Fig. 5b), demonstrating that CTHB NPs had no obvious acute toxicity to mice. On the 14th day after treatment, the tumors of each group were taken for weighing (Fig. 5c and d). In the control group, PBS + ultrasound group and CTHB NPs only group, the tumor weights were about 0.8 g, while the tumor weights in the SDT group were about 0.35 g. It was found that the tumors treated with SDT were significantly smaller than those in the three control groups. After different treatments, the tumors of mice were harvested and hematoxylin and eosin (H&E), terminal deoxynucleotidyl transferase-mediated dUTP nick-end labeling (TUNEL) stained tumor sections, and Ki67 immunohistochemical analysis were used to further evaluate the efficiency of SDT (Fig. 5e). It was found from the H&E staining images of tumor sections that the tumor cells of the mice in the ultrasound only or CTHB NPs only groups were not damaged. The tumor cells of mice were seriously damaged with the sonodynamic therapy. TUNEL staining analysis further proved that the tumor cells in the PBS + Ultrasound group and CTHB NPs only groups did not have obvious apoptosis, while the tumor cells in the SDT group were partially apoptotic. Ki67 immunohistochemical analysis proved that the proliferation ability of tumor cells in the SDT group was partially inhibited. The above experimental results indicate that the SDT of CTHB NPs can effectively inhibit tumor growth.
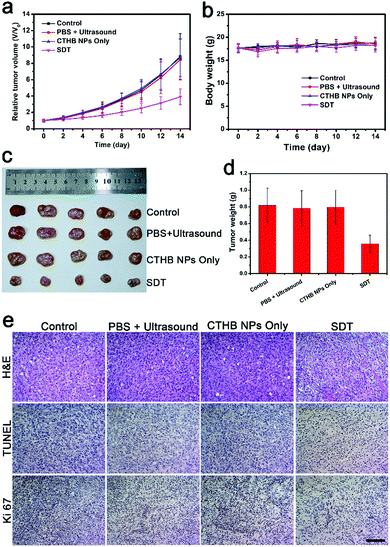 |
| Fig. 5 (a) Tumor growth curves in different groups. (b) Body weights of the mice after various treatments. (c) The photographs of tumors taken from mice on the 14th day in different groups. (d) Weight statistics of tumors taken from mice on the 14th day. (e) H&E, TUNEL, and Ki 67 staining images of tumor sections with different treatments. Scale bar: 100 μm. | |
2.6. Sonodynamic therapy of intracranial tumors
Because ultrasound has a deep tissue penetration ability,55–57 the SDT efficiency of CTHB NPs for intracranial tumors was further evaluated. The orthotopic glioblastoma-bearing mice were randomly divided into 3 groups (4 mice in each group): (1) PBS + ultrasound group; (2) CTHB NPs only group (100 μL, 1.0 mg mL−1); (3) CTHB NPs + ultrasound group (SDT group). After five hours for intravenous injection with CTHB NPs (100 μL, 1.0 mg mL−1), ultrasound irradiation (frequency: 1 MHz, intensity: 0.8 W cm−2, duty cycle: 20%, 5 min) in the brains of mice was conducted. As shown in Fig. 6a, the survival time of the mice in the SDT group was 8 days longer than those in the other two control groups, indicating that the growth of intracranial tumors in the SDT group was significantly inhibited. The H&E staining images of the brains on the 10th day after different treatments also showed that the tumor size in the SDT group was significantly smaller than those in the PBS + Ultrasound group and CTHB NPs only group (Fig. 6c). After different treatments, the body weights of mice in each group did not show obvious changes, indicating that the ultrasound in SDT was safe for mice and the CTHB NPs had good biocompatibility (Fig. 6b). In addition, the long-term toxicity of CTHB NPs towards mice was further investigated. Compared with the control groups, the H&E staining images on the 1st day, the 7th day and the 30th day with intravenous injection of CTHB NPs (10 mg kg−1) were obtained, and no obvious damage and inflammation were observed (Fig. S3, ESI†), further indicating that CTHB NPs had excellent biocompatibility. The results show that CTHB NPs in the SDT can effectively inhibit the growth of deep intracranial tumors.
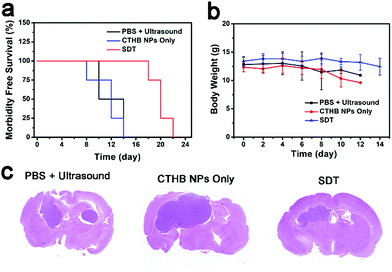 |
| Fig. 6 (a) Survival rates of mice in different groups. (b) Body weights of the mice with various treatments. (c) H&E staining images of slices of mice brain organs on the 10th day. | |
3. Conclusions
In summary, a cysteamine and tryptophan-modified hypocrellin derivative CTHB was synthesized, and CTHB NPs were prepared by encapsulating CTHB with biocompatible PEG–PLGA. The prepared CTHB NPs have good water solubility, broad absorption spectrum and emission in the near-infrared region. In addition, CTHB NPs could effectively generate singlet oxygen under ultrasound irradiation. Cell experiments showed that CTHB NPs could be effectively endocytosed by U87MG cells and had good biocompatibility and sonodynamic treatment effect. Animal experiments showed that CTHB NPs could be used for PA imaging-guided SDT to effectively inhibit tumor growth. More importantly, CTHB NPs could be effectively enriched in the intracranial tumors that could effectively inhibit the growth of intracranial tumors of mice in SDT. This sonotheranostic agent shows good application prospects in the diagnosis and treatment of intracranial tumors.
Author contributions
C. Zhang and J. Wu designed and characterized materials, analyzed the data and wrote the manuscript; W. Liu, W. Zhang and C. S. Lee provided useful suggestions to this work; X. Zheng assisted with data analysis; P. Wang organized and supervised the whole project.
Conflicts of interest
There are no conflicts to declare.
Acknowledgements
This research was supported by the National Natural Science Foundation of China (Grant No. 21873110, 61720106014 and 62005294), the Beijing Municipal Science & Technology Commission (No. Z191100004819004), and the Instrument Developing Project of the Chinese Academy of Sciences (No. YJKYYQ20170015).
References
- M. R. Gilbert, J. J. Dignam, T. S. Armstrong, J. S. Wefel, D. T. Blumenthal, M. A. Vogelbaum, H. Colman, A. Chakravarti, S. Pugh, M. Won, R. Jeraj, P. D. Brown, K. A. Jaeckle, D. Schiff, V. W. Stieber, D. G. Brachman, M. Werner-Wasik, I. W. Tremont-Lukats, E. P. Sulman, K. D. Aldape, W. J. Curran and M. P. Mehta, N. Engl. J. Med., 2014, 370, 699–708 CrossRef CAS.
- C. Alifieris and D. T. Trafalis, Pharmacol. Ther., 2015, 152, 63–82 CrossRef CAS.
- S. Roy, D. Lahiri, T. Maji and J. Biswas, South Asian J. Cancer, 2015, 4, 163–173 CrossRef PubMed.
- H. I. Robins, S. Chang, N. Butowski and M. Mehta, Curr. Oncol. Rep., 2007, 9, 66–70 CrossRef CAS PubMed.
- L. Cheng, C. Wang, L. Feng, K. Yang and Z. Liu, Chem. Rev., 2014, 114, 10869–10939 CrossRef CAS PubMed.
- D. E. Dolmans, D. Fukumura and R. K. Jain, Photodynamic Therapy for Cancer, Nat. Raev. Cancer, 2003, 3, 380 CrossRef CAS.
- B. M. Luby, C. D. Walsh and G. Zheng, Angew. Chem., Int. Ed., 2019, 58, 2558–2569 CrossRef CAS PubMed.
- L. Serpe, F. Foglietta and R. Canaparo, Nanotechnol. Rev., 2012, 1, 173–182 CAS.
- X. Lin, J. Song, X. Chen and H. Yang, Angew. Chem., Int. Ed., 2020, 59, 2–24 CrossRef.
- X. Pan, L. Bai, H. Wang, Q. Wu, H. Wang, S. Liu, B. Xu, X. Shi and H. Liu, Adv. Mater., 2018, 30, 1800180 CrossRef PubMed.
- P. Zhu, Y. Chen and J. Shi, ACS Nano, 2018, 12, 3780–3795 CrossRef CAS PubMed.
- J. Chen, H. Luo, Y. Liu, W. Zhang, H. Li, T. Luo, K. Zhang, Y. Zhao and J. Liu, ACS Nano, 2017, 11, 12849–12862 CrossRef CAS PubMed.
- F. Gong, L. Cheng, N. Yang, Y. Gong, Y. Ni, S. Bai, X. Wang, M. Chen, Q. Chen and Z. Liu, Nat. Commun., 2020, 11, 3712 CrossRef CAS PubMed.
- X. Wang, X. Zhong, L. Bai, J. Xu, F. Gong, Z. Dong, Z. Yang, Z. Zeng, Z. Liu and L. Cheng, J. Am. Chem. Soc., 2020, 142, 6527–6537 CrossRef CAS.
- A. Ma, H. Chen, Y. Cui, Z. Luo, R. Liang, Z. Wu, Z. Chen, T. Yin, J. Ni, M. Zheng and L. Cai, Small, 2019, 15, e1804028 CrossRef PubMed.
- F. Gong, L. Cheng, N. Yang, O. Betzer, L. Feng, Q. Zhou, Y. Li, R. Chen, R. Popovtzer and Z. Liu, Adv. Mater., 2019, 31, 1900730 CrossRef PubMed.
- X. Wang, X. Zhong, Z. Liu and L. Cheng, Nano Today, 2020, 35, 100946 CrossRef CAS.
- G. Li, S. Wang, D. Deng, Z. Xiao, Z. Dong, Z. Wang, Q. Lei, S. Gao, G. Huang, E. Zhang, G. Zeng, Z. Wen, S. Wu and Z. Liu, ACS Nano, 2020, 14, 1586–1599 CrossRef CAS PubMed.
- L. Sun, Y. Xu, X. Zhang, Y. Gao, J. Chen, A. Zhou, Q. Lu, Z. Wang, K. Shao, H. Wu and X. Ning, Adv. Mater., 2020, 32, 2005295 CrossRef CAS PubMed.
- S. Son, J. H. Kim, X. Wang, C. Zhang, S. A. Yoon, J. Shin, A. Sharma, M. H. Lee, L. Cheng, J. Wu and J. S. Kim, Chem. Soc. Rev., 2020, 49, 3244–3261 RSC.
- J. Huang, F. Liu, X. Han, L. Zhang, Z. Hu, Q. Jiang, Z. Wang, H. Ran, D. Wang and P. Li, Theranostics, 2018, 8, 6178–6194 CrossRef CAS PubMed.
- Y. Zhou, X. Liang and Z. Dai, Nanoscale, 2016, 8, 12394–12405 RSC.
- H. Zhang, J. Chen, X. Zhu, Y. Ren, F. Cao, L. Zhu, L. Hou, H. Zhang and Z. Zhang, J. Mater. Chem. B, 2018, 6, 6108–6121 RSC.
- H. Gong, Z. Dong, Y. Liu, S. Yin, L. Cheng, W. Xi, J. Xiang, K. Liu, Y. Li and Z. Liu, Adv. Funct. Mater., 2014, 24, 6492–6502 CrossRef CAS.
- K. Hayashi, M. Nakamura, H. Miki, S. Ozaki, M. Abe, T. Matsumoto, T. Kori and K. Ishimura, Adv. Funct. Mater., 2014, 24, 503–513 CrossRef CAS.
- M. A. Rajora, J. W. H. Lou and G. Zheng, Chem. Soc. Rev., 2017, 46, 6433–6469 RSC.
- V. Almeida-Marrero, E. van de Winckel, E. Anaya-Plaza, T. Torres and A. de la Escosura, Chem. Soc. Rev., 2018, 47, 7369–7400 RSC.
- B. T. Paul, M. S. Babu, T. R. Santhoshkumar, D. Karunagaran, G. S. Selvam, K. Brown, T. Woo, S. Sharma, S. Naicker and R. Murugesan, J. Photochem. Photobiol., B, 2009, 94, 38–44 CrossRef CAS PubMed.
- H. Deng, T. Li, J. Xie, N. Huang, Y. Gu and J. Zhao, Dyes Pigm., 2013, 99, 930–939 CrossRef CAS.
- H. Deng, X. Liu, J. Xie, R. Yin, N. Huang, Y. Gu and J. Zhao, J. Med. Chem., 2012, 55, 1910–1919 CrossRef CAS.
- Q. Xiao, J. Wu, X. Pang, Y. Jiang, P. Wang, A. W. Leung, L. Gao, S. Jiang and C. Xu, Curr. Med. Chem., 2018, 25, 839–860 CrossRef CAS.
- X. Zheng, W. Liu, J. Ge, Q. Jia, F. Nan, Y. Ding, J. Wu, W. Zhang, C. S. Lee and P. Wang, ACS Appl. Mater. Interfaces, 2019, 11, 18178–18185 CrossRef CAS.
- C. Zhang, J. Wu, W. Liu, X. Zheng and P. Wang, ACS Appl. Mater. Interfaces, 2019, 11, 44989–44998 CrossRef CAS PubMed.
- C. Zhang, J. Wu, W. Liu, X. Zheng, W. Zhang, C. S. Lee and P. Wang, ACS Appl. Bio Mater., 2020, 3, 3817–3826 CrossRef CAS.
- Y. Ding, W. Liu, J. Wu, X. Zheng, J. Ge, H. Ren, W. Zhang, C. S. Lee and P. Wang, Chem. – Asian J., 2020, 15, 3462–3468 CrossRef CAS.
- H. Wang, Q. Jia, W. Liu, F. Nan, X. Zheng, Y. Ding, H. Ren, J. Wu and J. Ge, ChemMedChem, 2020, 15, 177–181 CrossRef CAS PubMed.
- X. Wang, A. W. Leung, Y. Jiang, H. Yu, X. Li and C. Xu, Ultrasonics, 2012, 52, 543–546 CrossRef CAS.
- Y. Jia, X. Wang, Q. Liu, A. W. Leung, P. Wang and C. Xu, Ultrasonics, 2017, 73, 154–161 CrossRef CAS.
- Y. Liu, H. Bai, K. Guo and P. Wang, J. Chemother., 2020, 32, 385–393 CrossRef CAS.
- S. Xu, S. Chen, M. Zhang, T. Shen, X. Zhang and Z. Wang, Photochem. Photobiol., 2003, 78, 411–415 CrossRef CAS.
- X. Liu, J. Xie, L. Zhang, H. Chen, Y. Gu and J. Zhao, Chin. Sci. Bull., 2009, 54, 2045–2050 CAS.
- K. Li, D. Ding, D. Huo, K. Y. Pu, N. N. P. Thao, Y. Hu, Z. Li and B. Liu, Adv. Funct. Mater., 2012, 22, 3107–3115 CrossRef CAS.
- P. Upadhyay, S. Sarker, A. Ghosh, P. Gupta, S. Das, M. Ahir, S. Bhattacharya, S. Chattopadhyay, S. Ghosh and A. Adhikary, Biomater. Sci., 2019, 7, 4325–4344 RSC.
- L. B. Cao, S. Zeng and W. Zhao, Nanoscale Res. Lett., 2016, 11, 305 CrossRef PubMed.
- Y. Dai, C. Xu, X. Sun and X. Chen, Chem. Soc. Rev., 2017, 46, 3830–3852 RSC.
- L. Zhao, W. Yuan, J. Li, L. Yang, Y. Su, J. Peng, R. Chen, H. P. Tham, H. Chen, W. Q. Lim, H. Xiang, P. Xing, F. Li and Y. Zhao, Adv. Funct. Mater., 2018, 28, 1806162 CrossRef.
- E. Nance, C. Zhang, T. Y. Shih, Q. Xu, B. S. Schuster and J. Hanes, ACS Nano, 2014, 8, 10655–10664 CrossRef CAS PubMed.
- J. Mo, L. He, B. Ma and T. Chen, ACS Appl. Mater. Interfaces, 2016, 8, 6811–6825 CrossRef CAS PubMed.
- J. M. Morachis, E. A. Mahmoud and A. Almutairi, Pharmacol. Rev., 2012, 64, 505–519 CrossRef CAS PubMed.
- M. Aldea, I. A. Florian, G. Kacso, L. Craciun, S. Boca, O. Soritau and I. S. Florian, Pharm. Res., 2016, 33, 2059–2077 CrossRef CAS PubMed.
- J. Mo, L. He, B. Ma and T. Chen, ACS Appl. Mater. Interfaces, 2016, 8, 6811–6825 CrossRef CAS.
- E. A. Nance, G. F. Woodworth, K. A. Sailor, T.-Y. Shih, Q. Xu, G. Swaminathan, D. Xiang, C. Eberhart and J. Hanes, Sci. Transl. Med., 2012, 149, 119 Search PubMed.
- E. Nance, C. Zhang, T.-Y. Shih, Q. Xu, B. S. Schuster and J. Hanes, ACS Nano, 2014, 8, 10655–10664 CrossRef CAS.
- E. Song, A. Gaudin, A. R. King, Y.-E. Seo, H.-W. Suh, Y. Deng, J. Cui, G. T. Tietjen, A. Huttner and W. M. Saltzman, Nat. Commun., 2017, 8, 15322 CrossRef CAS.
- Y. Liu, G. Wan, H. Guo, Y. Liu, P. Zhou, H. Wang, D. Wang, S. Zhang, Y. Wang and N. Zhang, Nano Res., 2017, 10, 834–855 CrossRef CAS.
- J. Shi, Z. Chen, B. Wang, L. Wang, T. Lu and Z. Zhang, ACS Appl. Mater. Interfaces, 2015, 7, 28554–28565 CrossRef CAS.
- Y. W. Chen, T. Y. Liu, P. H. Chang, P. H. Hsu, H. L. Liu, H. C. Lina and S. Y. Chen, Nanoscale, 2016, 8, 12648–12657 RSC.
Footnote |
† Electronic supplementary information (ESI) available. See DOI: 10.1039/d1tb01886h |
|
This journal is © The Royal Society of Chemistry 2022 |
Click here to see how this site uses Cookies. View our privacy policy here.