DOI:
10.1039/D1TA08495J
(Review Article)
J. Mater. Chem. A, 2022,
10, 2672-2696
Regioregularity-control of conjugated polymers: from synthesis and properties, to photovoltaic device applications
Received
30th September 2021
, Accepted 22nd December 2021
First published on 29th December 2021
Abstract
In the last few decades, extensive academic and industrial efforts have been devoted to developing high-performance conjugated polymers (CPs) for organic electronics. Specifically, the relationships between the molecular structures of CPs and their properties and device characteristics have been a subject of intense studies. In this review, we highlight recent advances in the molecular design of CPs, particularly on tuning their regioregularity (RR). The RR of repeating units (i.e., directional, positional, and sequential regularities) within the CP backbone determines the intrinsic properties of CPs and the performances of the resulting devices. Despite the significant impact of RR on the overall properties of the polymers and the device performances, the importance of RR in the design of CPs has yet to be emphasized. Furthermore, RR control is critical in state-of-the-art CPs with asymmetric molecular structures. This review presents a library of examples that report the impact of RR control in CPs on the properties and performances in various organic electronic devices, including simple homopolymers with asymmetric alkyl side chains and more recently developed copolymers with various donor–acceptor (D–A) combinations. This review summarizes important guidelines and provides insights for the molecular design of RR-controlled CPs and their applications in efficient organic electronics.
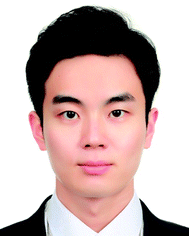 Youngkwon Kim | Youngkwon Kim received a B.S. degree from the Department of Chemical and Biomolecular Engineering from KAIST in 2014 and a Ph.D. degree under Prof. Bumjoon Kim from KAIST in 2020. Afterward he joined Samsung Electronics as a staff engineer. His research focuses on synthesizing functional conjugated polymers, conjugated polymer-based block copolymer self-assembly, and organic electronic applications. |
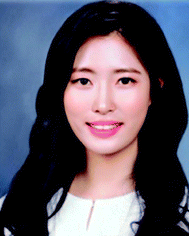 Hyeonjung Park | Hyeonjung Park received a B.S. degree from the Department of Chemical and Biomolecular Engineering from KAIST in 2016. She is currently a Ph.D. candidate under Prof. Bumjoon Kim in KAIST. Her research focuses on the development of high-performance and functional conjugated polymers for organic electronics and mechanical/crystallization behaviors of conjugated polymers. |
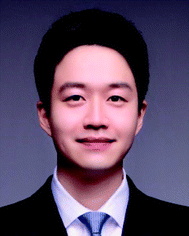 Hyeong Jun Kim | Hyeong Jun Kim is an assistant professor in the Department of Chemical and Biomolecular Engineering at Sogang University. He received a Ph.D degree from the Department of Chemical and Biomolecular Engineering at KAIST under the supervision of prof. Bumjoon Kim. After that, He joined the University of Massachusetts Amherst in the Department of Polymer Science and engineering as a postdoctoral research associate. His postdoc host was prof. Ryan Hayward. His research interests involve physical phenomena of charged and conjugated macromolecules as well as their applications in soft ionic/electronic devices. |
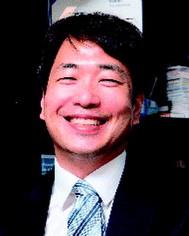 Bumjoon J. Kim | Bumjoon Kim is a KAIST Endowed Chair Professor and Department Head of the Department of Chemical and Biomolecular Engineering at KAIST. He received his Ph.D. degree from UC Santa Barbara under the guidance of Prof. Edward Kramer. Afterward he worked with Prof. Jean Fréchet at UC Berkeley as a postdoc researcher. His current research includes polymer designs for the development of high-performance and mechanically-robust solar cells, polymeric electrolyte and smart responsive block copolymer-based particles. |
1. Introduction
The development of high-performance conjugated polymers (CPs) has been a long-sought goal for numerous organic electronic applications such as polymer solar cells (PSCs), organic light-emitting diodes (OLEDs), and organic field-effect transistors (OFETs).1–7 Through a proper design with versatile chemical structures, CPs can afford excellent optoelectrical and mechanical properties and solution processability for cost-effective production.8–10 These unique features have driven a significant amount of academic and industrial efforts to develop an extensive library of CPs. From these efforts, the figures of merit of organic electronic devices employing CPs have been improved to approach the benchmarks for commercialization typically achieved with electronic devices based on inorganic semiconductors.
With the successful demonstration of CP-based devices, several important molecular design guidelines have been established. These include the backbone structure, alkyl side chain selection, electron-rich donor (D)–electron-poor acceptor (A) alternating copolymer structures, and various electron-withdrawing/donating moieties for efficient CPs.11–14 The impact of the molecular structures of CPs on their optoelectrical properties and corresponding device performances has been extensively investigated.15–18 Among many molecular design parameters, the regioregularity (RR)—geometric regularity of repeating units in CPs—has emerged as an important criterion for achieving high-performance CPs with complex chemical structures.19–21 Although previous literature has revealed the impact of RR on the properties of CPs and the associated device performances,22–26 the importance of RR in the design of CPs has attracted less attention.
In this review, we describe RR control in a variety of CPs and overview its impact on the properties of CPs and their performances in photovoltaic devices, including recent examples of the state-of-the-art D–A copolymers. Traditionally, the definition of RR has been limited to simple homopolymers with asymmetric alkyl side chains such as poly(3-alkylthiophene)s (P3ATs). To expand our understanding of RR to more sophisticated CP structures, three different categories of RRs are considered: (i) directional RR, (ii) positional RR, and (iii) sequential RR. While several reports independently define the RR of different CPs including D–A copolymers, the more systematic classification of RR introduced in this review will improve our understanding of the structure of CPs in terms of their RR. This review presents a library of examples of directional, positional, and sequential RR-controlled CPs and summarizes the impact of RR on the properties of CPs and their performances in organic electronics. Finally, the conclusions drawn from this review and the outlook for further development of RR-controlled CPs are presented.
2. Regioregularity of conjugated polymers
2.1. Definition of regioregularity (RR)
The RR of CPs describes the geometrical symmetry of each monomer unit along the polymer chain. The number of identical regio-isomers in the polymers determines RR, i.e., a RR of 100% indicates perfectly regio-regular (rre) CPs, where all repeating units in the polymers consist of the same regio-isomer. Thus, the presence of other regio-isomers lowers the RR of the CPs. The RR of polymeric materials, particularly of CPs, strongly impacts their intrinsic properties such as thermal, crystalline, rheological, mechanical and optoelectrical properties. The definition of RR of CPs has been well-established for simple conjugated homopolymers with asymmetric alkyl side chains.20,21 However, a more systematic definition of RR is required for state-of-the-art CPs with more complex chemical structures. While several excellent research papers and reviews independently define RR of advanced conjugated materials,19–21 this review presents an extensive library of recent high-performance CPs and classifies them into three categories: directional RR; positional RR; and sequential RR (Fig. 1).
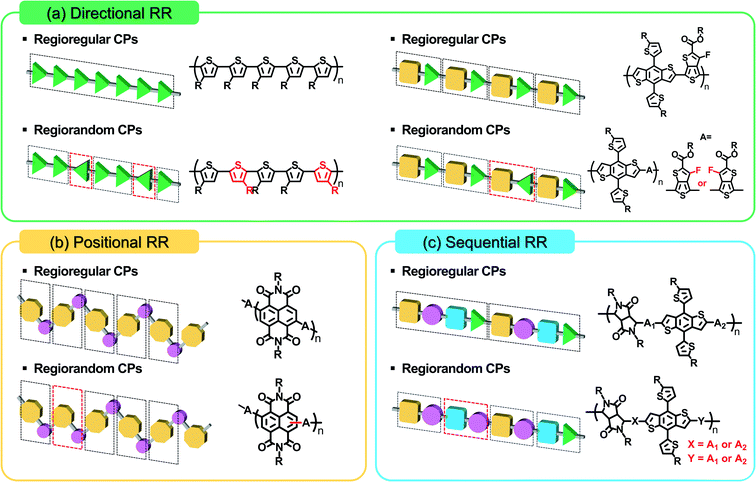 |
| Fig. 1 Structural representation of (a) directional, (b) positional, and (c) sequential RRs of CPs. | |
Directional RR is defined as the fraction of asymmetric repeating units that are arranged in the same direction along a polymer chain. The most representative example of directional RR is P3ATs. During the polymerization of P3ATs, two 3-alkylthiophenes can adopt four possible arrangements: head-to-head (H–H), tail-to-tail (T–T), and two head-to-tail (H–T) bonded thiophene rings. In these systems, the RR of P3ATs is quantified by the amount of H–T bonds in the polymer chain.27–29 Perfectly rre P3AT contains solely H–T bonds whereas the regio-random (rra) one comprises a mixture of isomers, resulting in randomly oriented alkyl chains along the CP backbone (RR = 50%). Similarly, directional RR in other types of CPs can be determined by the directional orientation of the functional groups or side-chains along the conjugated backbone.30–35 One example of D–A type CPs with directional RR is poly[4,8-bis(5-(2-ethylhexyl)thiophene-2-yl)benzo[1,2-b:4,5-b′]dithiophene-2,6-diyl-alt-(4-(2-ethylhexyl)-3-fluorothieno[3,4-b]thiophene-)-2-carboxylate-2-6-diyl] (PTB7-Th), where D is a symmetric benzodithiophene and A is an asymmetric fluoro-substituted thienothiophene (Fig. 1a).36rre PTB7-Th can be obtained when the fluorine moieties are arranged in one direction along the polymer backbone, whereas rra PTB7-Th contains randomly oriented fluorine atoms along the chain.
RR can also be represented by the positional orientation of covalent bonds between monomer units in CP backbones.37–42 An example of positional RR can be found in poly([N,N′-bis(2-octyldodecyl)naphthalene-1,4,5,8-bis(dicarboximide)-2,6-diyl]-alt-5,5′-(2,2′-bithiophene)) (P(NDI2OD-T2) or N2200), which is one of the most widely used n-type CPs.42–44 The naphthalene diimide (NDI) repeating moiety of P(NDI2OD-T2) has four different reactive sites for hydrogen, which can be substituted with bromines in the 2,6- or 2,7-position. Then, the mixture of brominated NDI monomers can undergo either 2,6- or 2,7-oxidation additions between the D and A units during polymerization, resulting in positional regio-isomers (Fig. 1b). Thus, positional RR is defined by the number of the same additions included in the P(NDI2OD-T2) polymer chains. rre P(NDI2OD-T2) has one type of addition (either 2,6 or 2,7) of NDI, whereas rra P(NDI2OD-T2) contains a random mixture of isomerized bonds. Similarly, positional RR can be defined for other CPs if the constituent monomers contain more than one active polymerization site.
Lastly, many D–A alternating copolymers can exhibit sequential RR.45–50 Most of the recent high-performance CPs used in organic electronics are based on D–A molecular structures, where D and A are alternately coupled along the CP backbones. In general, D–A copolymers are prepared via multiple coupling reactions between D and A moieties in an alternating sequence of D–A structures. When the molecular structure of D or A is asymmetric, sequential regularity—H–H or H–T arrangements—can be induced during the coupling polymerization (Fig. 1c). Moreover, different monomer sequences can be introduced in conjugated terpolymers (D–A1–D–A2) or tetrapolymers (D1–A1–D2–A2).45–49 Sequentially rre terpolymers or tetrapolymers comprise repeating (D1–A1) and (D2–A2) sequences (or (D–A1) and (D–A2)) that alternate throughout the polymer chain. In contrast, rra polymers comprise a random sequence of donors (D1 or D2) and acceptors (A1 or A2) in the conjugated terpolymers or tetrapolymers.
Here, we present an overview of directional, positional and sequential RR controlled CPs and their intrinsic properties, starting from simple conjugated homopolymers with asymmetric alkyl chains. Thereafter, the discussion is expanded to more recently developed complex CP structures, particularly focusing on the D–A type copolymers.
2.2 RR control of homopolymers with asymmetric alkyl chains
In the early 1970s, P3ATs were developed by incorporating alkyl side chains along the thiophene backbone to address the low solution processability of polythiophenes.51–53 During that time, P3ATs were often obtained by the reduction of thiophene rings from electro-chemical polymerization with strong dopants such as FeCl3, LiBF4, and NBu4PF6 (A–D).54–56 Although these methods offer a facile synthesis of P3ATs, most electro-chemical methods typically yield regio-irregular (rir) P3ATs as regio-selective coupling of 3-alkylthiophenes does not occur during the electro-chemical polymerization process. We note that rra indicates nearly 50% of RR, while rir indicates the middle range of RR between rre and rra (e.g., RR = 80 and 70%, Fig. 2).
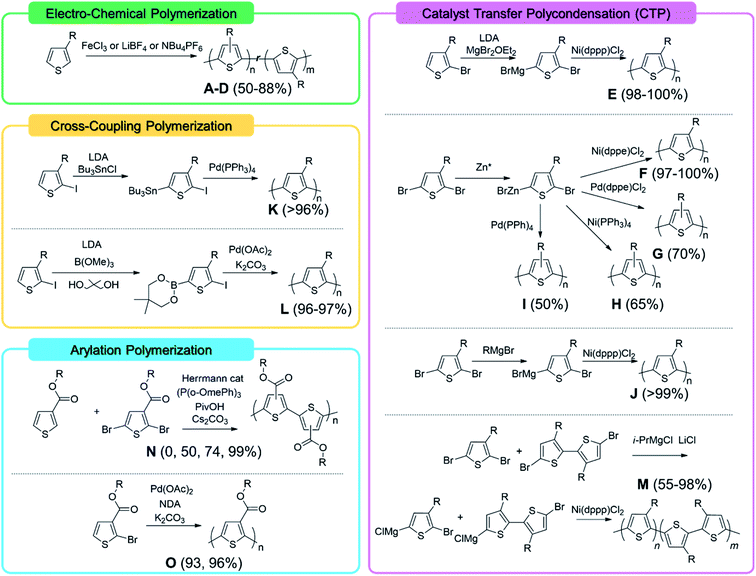 |
| Fig. 2 Synthesis of P3ATs with different RRs via various methods: electro-chemical polymerization,54–56 cross-coupling polymerization,70,71 arylation polymerization,75,76 and catalyst transfer polycondensation.25,60–63,74 | |
A rre P3AT was first synthesized by McCullough in 1992 using lithium diisopropylamide and MgBr2·Et2O with 2-bromo-3-alkylthiophene to produce 2-bromo-5-bromomagnesio-3-alkylthiophene for regio-selective coupling of 3-alkylthiophenes.57–59 Subsequent catalyst transfer polymerization (CTP) of 2-bromo-5-bromomagnesio-3-alkylthiophene by a cross-coupling reaction in the presence of a Ni(dppp)Cl2 catalyst afforded the target with 98–100% of RR (E).60 Around the same time, the Rieke method was developed, where 2,5-dibromo-3-alkylthiophenes react with Rieke Zn and are subsequently polymerized with Ni(dppe)Cl2 to yield high RR (≥97%) P3ATs (F). Different degrees of RR can be achieved by employing different organic ligands and metal catalysts.61,62 For example, RRs of 70%, 65%, and 50% were obtained by CTP using Pd(dppe)Cl2, Ni(PPh3)4, and Pd(PPh3)4 catalysts, respectively (G–I). In 1999, McCullough developed Grignard metathesis (GRIM method) with alkyl-MgCl to provide regio-selectivity for 2,5-dibromo-3-alkylthiophenes without cryogenic temperatures and hazardous metal catalysts (J).63 Importantly, the CTP method can exhibit a living behavior, with precise control of the molecular weight and narrow dispersity, making it an attractive model system for investigating the fundamentals of CPs, including their molecular behaviors and properties.64–67 Moreover, various P3AT-based block copolymers can be prepared by exploiting the living characteristics of CTP reactions, facilitating studies of their unique properties and morphologies.68,69 Another important approach for achieving high RR P3ATs is through cross-coupling polymerization reactions. Barker and co-workers obtained P3ATs with over 96% RR by Stille cross-coupling reactions with 2-iodo-5-Bu3Sn-3-alkylthiophene (K).70 Additionally, Bidan and co-workers adopted Suzuki coupling condensation reactions to yield P3ATs with 96–97% RR (L).71 Recently, ester-functionalized polythiophene with high regioselectivity could also be obtained through nickel-catalyzed Suzuki polycondensation.72 Although air-free techniques are necessary for preparing organometallic monomers under cryogenic conditions, these palladium assisted cross-coupling methods produce well-defined P3ATs with RRs of over 96% (Table 1).
Table 1 Various methods for the synthesis of P3ATs with different RRs
Label |
Method |
RR [%] |
Reactant |
Alkyl side chain |
Catalyst |
Solvent |
Ref. |
A
|
Chemical polymerization |
50 |
3-Alkylthiophene |
Butyl, |
FeCl3 |
CHCl3 |
54
|
hexyl, and |
icosanyl |
B
|
Electro-polymerization |
50 |
3-Alkylthiophene |
Butyl, |
LiBF4 |
MeCN |
54
|
hexyl, and |
icosanyl |
C
|
Electro-polymerization |
70–80 |
3-Alkylthiophene |
Hexyl, |
NBu4PF6 |
PhNO2 |
55
|
octyl, |
decyl, and |
dodecyl |
D
|
Chemical polymerization |
68–88 |
3-Hexylthiophene |
Hexyl |
FeCl3 |
CHCl3 |
56
|
E
|
McCullough |
98–100 |
2-Bromo-3-alkylthiophene |
Butyl, |
LDA and MgBr2OEt2 |
THF |
60
|
hexyl, |
Ni(dppp)Cl2 |
octyl, and |
dodecyl |
F
|
Rieke |
97–100 |
2,5-Dibromo-3-alkylthiophene |
Butyl, |
ZnBr |
THF |
61 and 62 |
hexyl, |
Ni(dppe)Cl2 |
octyl, |
decyl, |
dodecyl, and |
tetradecyl |
G
|
Rieke |
70 |
2,5-Dibromo-3-alkylthiophene |
Hexyl |
ZnBr |
THF |
61
|
Pd(dppe)Cl2 |
H
|
Rieke |
65 |
2,5-Dibromo-3-alkylthiophene |
Hexyl |
ZnBr |
THF |
61
|
Ni(PPh3)4 |
I
|
Rieke |
50 |
2,5-Dibromo-3-alkylthiophene |
Butyl, hexyl, |
ZnBr |
THF |
61
|
and octyl |
Pd(PPh3)4 |
J
|
GRIM |
>99 |
2,5-Dibromo-3-alkylthiophene |
Dodecyl |
RMgX |
THF |
63
|
Ni(dppp)Cl2 |
K
|
Stille coupling |
>96 |
2-Iodo-5-Bu3Sn-3-alkylthiophene |
Hexyl |
Pd(PPh3)4 |
Tol |
70
|
L
|
Suzuki coupling |
96–97 |
2-Iodo-5-boronate-3-alkylthiophene |
Octyl |
Pd(Oac)2, K2CO3 |
THF |
71
|
M
|
GRIM |
55–98 |
2,5-Dibromo-3-alkylthiophene |
Hexyl and |
IsoPrMgCl |
THF |
25 and 74 |
5′-Bromo-3,4′-dialkyl-5-bromo-[2,2′]-bithiophene |
dodecyl |
Ni(dppp)Cl2 |
N
|
Arylation |
0 and 50 |
3-Octyloxycarbonylthiophene |
Octyl |
Herrmann cat. (P(o-OmePh)3) |
Tol |
75
|
74 and 99 |
2,5-Dibromo-3-octyloxycarbonylthiophene |
PivOH, Cs2CO3 |
O
|
Arylation |
93 and 96 |
5-Dibromo-3-octyloxycarbonylthiophene |
Hexyl |
Pd(OAc)2, K2CO3, and neodecanoic acid |
DMA |
76
|
While several polymerization methods have been proposed for producing highly rre P3ATs, precise control of RR of P3ATs remains a great challenge. Fréchet et al. first suggested a simple synthetic method of controlling RR of poly(3-hexylthiophene) (P3HT) by copolymerizing the 3-hexylthiophene monomer and a H–H coupled 3,4′-dihexyl-2,2′-bithiophene dimer.73 Later, Kim and co-workers further developed this method to extend the RR from 55 to 98% by controlling the monomer to H–H coupled dimer feed ratio (M).25,74 Because this approach using the H–H coupled dimer is based on living GRIM polymerization, well-controlled molecular weight depending on the monomer/catalyst ratio and low-dispersity P3HT with precisely tuned RR could be achieved. Alternatively, RR of poly(3-octyloxycarbonylthiophene)s (P3OETs) can be controlled through palladium-catalyzed direct arylated polycondensation (DArP).75,76 The DArP method is based on direct hetero C–H/C–H coupling, which does not require additional functionalization and metalation of the monomers for the polymerization. Gobalasingham et al. synthesized a rre P3OET with a RR higher than 93% using DArP polycondensation (O).76 Later, Menda et al. reported a series of P3OETs with different RRs of 0, 50, 74, and 99%, which has been obtained depending on the combination of 3-octyloxycarbonylthiophene and 2,5-dibromo-3-octyloxycarbonylthiophene monomers (N).75
It is well known that the intrinsic properties of P3ATs are significantly impacted by the RR of their conjugated backbone. The presence of regio-defects (T–T and H–H bonds) in P3ATs induces a large steric hindrance between their alkyl substituents (Fig. 3). Thus, rir linkages in low RR P3ATs induce a steric twisting of the thiophene rings, resulting in short persistence lengths,77 small effective conjugation lengths,78 small crystallite thicknesses,27 and more coil-like chain conformations.79 Consequently, the properties of rre and rra P3ATs become completely different such as thermal properties, crystallinities, self-assembled morphologies, optoelectrical properties, and mechanical properties.
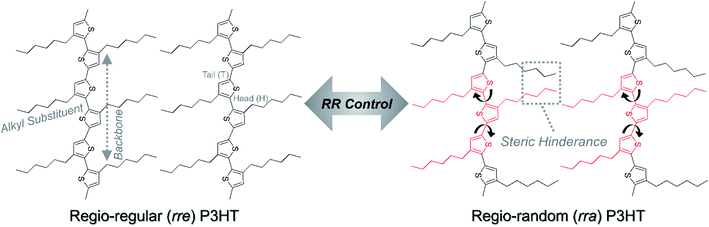 |
| Fig. 3 Molecular structures of rre and rra P3HTs. | |
The RR of P3ATs is well-recognized as a key parameter for determining their crystalline properties (Fig. 4a). For example, the differential scanning calorimetry (DSC) profile of rre P3HTs shows a distinct endothermic peak and high Tm (∼220 °C) from the melting of well-ordered crystallites, whereas no melting peak was observed for rra P3HTs, indicating their amorphous nature.78,80 In particular, Kim et al. produced a series of P3HTs with a wide range of RR from 60 to 98% and compared their crystallization behaviors as a function of RR.25 As the RR of the P3HTs decreased, Tm and ΔHm gradually decreased, attributed to smaller crystalline domains with larger regio-defects. The degree of crystallinity of the P3HTs gradually decreased from 48 (RR = 98%) to 22% (RR = 80%) and to 16% (RR = 75%). When the RR of P3HT was lower than 75%, the crystallization of P3HT was significantly suppressed and no endothermic peaks from melting crystallites were observed by DSC. In addition to the bulk crystallization properties, RR-dependent crystal orientations in thin-films were investigated by Leeuw et al.81 The crystal orientations in thin films, such as in the in-plane or out-of-plane direction, are particularly important for various organic electronic applications, i.e., horizontal charge transportation in OFETs and vertical direction charge transport in PSCs. The rre P3ATs predominantly crystallize into edge-on oriented structures, showing sharp, high-order (h00) lamellar stacking in the out-of-plane direction, along with strong (010) in-plane π–π stacking. In contrast, the rir P3ATs adopt face-on oriented crystal structures, supported by weak (h00) peaks in the in-plane direction and distinct amorphous halo rings.81–83
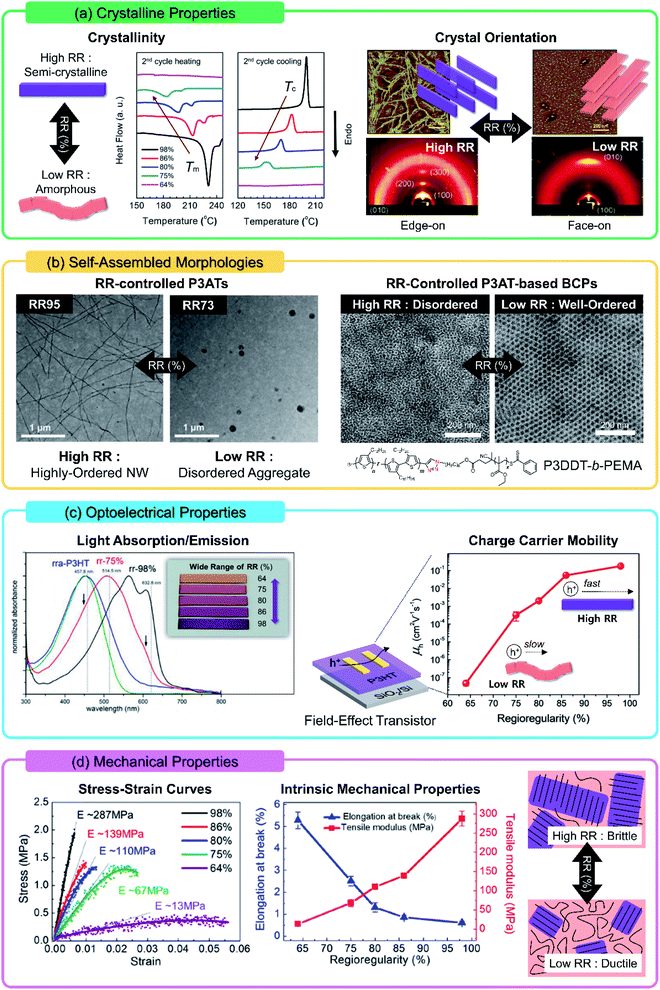 |
| Fig. 4 Influences of RR on the (a) crystallinity, (b) self-assembled morphologies, (c) optoelectrical properties, and (d) mechanical properties of P3ATs as a function of RR. Reproduced with permission.25,81,86,87,92 Copyright 2015, American Chemical Society, copyright 1999, Nature, copyright 2021, Elsevier, copyright 2019, Elsevier, and copyright 2018, American Chemical Society. | |
Such RR dependent structural differences in the P3AT chains can significantly affect their self-assembly behavior. For example, strong π–π interactions in high RR P3HTs drive their unique one-dimensional assembly into nanowires (NWs), which act as highly effective long charge transport pathways in organic electronics (Fig. 4b).84,85 In contrast, the average length of the NWs became much shorter as the RR decreased, and the rra P3HTs adopted irregular structures.86 Thus, systematic RR modulation allows for precise control of the intermolecular interactions between CPs. For example, most P3HT-based block copolymers exhibit only fibril-like morphologies, irrespective of the type and volume fraction of the second block. This is because the strong crystallization in the P3HT block dominates the phase behaviors, resulting in kinetically trapped morphologies. Lowering the RR allows the formation of ordered nanostructures by weakening the initially strong molecular interactions in the P3HT blocks and, thus, enthalpic interaction can govern the phase behaviors of the block copolymers.87–89
These different self-assembled structures formed by rre and rra P3ATs result in significant differences in their optoelectrical properties (Fig. 4c). For example, the correlations between the RR-dependent chain conformations and the UV-vis absorption spectra of P3HTs were relatively well investigated.90–92 High RR P3HTs showed distinct absorption peaks at around 2.09, 2.27, and 2.37 eV (600, 546, and 523 nm), corresponding to the vibronic coupling of π–π* transition. The ratio between the 0–0 peak (at 600 nm) and 0–1 peak (at 546 nm) was highly correlated with strong intra/interchain interactions in well-ordered crystallites (rod-like chain conformations).93 In contrast, the absorption spectrum of the low RR P3HT was blue-shifted with a maximum peak and broad shoulder appearing at 2.72 eV (456 nm) and 3.0 eV (413 nm), respectively. These changes are attributed to a decrease in the effective conjugation length of amorphous P3HTs (coil-like chain conformations), which require more energy for electron excitation. Moreover, based on photoluminescence (PL) analysis, it was reported that the emissions of rre and rra P3HTs differed.94,95 The emission spectrum of rra P3HTs showed a relatively weak and blue-shifted emission compared to that of rre P3HTs due to the weak intra/interchain interactions, similar to the trends observed for the UV-vis absorption spectra. Interestingly, photoexcitation dynamics of the rre P3HTs were completely different from those of the rra isomers, with a long fluorescence decay lifetime (576 ps), where the emission arising from only a single species (the first excited singlet exciton) participates as supported by the wavelength-independent quasi single exponential spectrum.93,96 Thus, the suppressed interchain interactions in rra P3HTs promote faster energy transfer relaxation to generate low energy states.
The relationships between the RRs and electrical properties of P3ATs have been extensively examined by comparing the charge-carrier mobilities (μ) in OFETs (Fig. 4c). In the 1990s, it was first reported that rre P3ATs can afford a much higher value of μ (at least 2 orders of magnitude) compared to rra P3ATs.97–99 As the RR decreases, the regio-defects in the polymer chains twist the polymer backbone, distorting the efficient charge transport pathway, thereby yielding a low μ. For instance, Leeuw et al. reported three orders of the magnitude improved μ from 2 × 10−4 to 1 × 10−1 cm2 V−1 s−1, by increasing the RR value from 81 to 96%, respectively.81 In this regard, many researchers have made efforts to enhance μ by achieving very high RR of P3ATs.100–104 High RR P3HTs form well-ordered intermolecular structures with broader delocalization of the π-electrons, leading to efficient charge transport pathways.
Lastly, the RR also influences the mechanical properties of P3ATs (Fig. 4d). Tuning the mechanical properties of CPs has recently emerged as an important parameter in the design of CPs, particularly for applications in wearable/portable electronics. Kim et al. compared the stretchability (crack-onset strain, COS) and tensile modulus of a series of P3HTs with various RR values (from 64 to 98%).25 In the free-standing tensile test of P3HT thin films on water, a significant increase in the COS from 0.6 to 5.3% and a decrease in the tensile modulus from 287 to 13 MPa were observed for lower RR materials. The enhanced tensile strength and stretchability of low RR P3HTs are attributed to their large amorphous domains, allowing efficient stress relaxations by alignment of the chains in the strain directions. In contrast, excessive crystalline regions in high RR domains can act as an initial crack point, leading to low energy dissipations under strains, resulting in a mechanical failure even under low strain. When incorporated into flexible devices, P3HTs with a RR of 64% exhibited well-maintained current–voltage curves after 800 bending test cycles, whereas the 98% RR P3HTs failed after only 50 bending cycles. Thus, tuning the RR of CPs is an effective means of engineering the mechanical properties of CPs for various organic electronic applications. While there is typically a trade-off relationship between the mechanical and electrical properties of CPs depending on their RR values,105 it is highly desirable to achieve both for efficient electronic devices including the flexible and stretchable devices.15,106–113 In this regard, various strategies for developing robust, high performing conjugated materials have been reported. For example, blending the two polymers with different RRs or combining high RR and low RR blocks in one polymer chain can simultaneously optimize the mechanical and electrical performances (Fig. 5).114–116
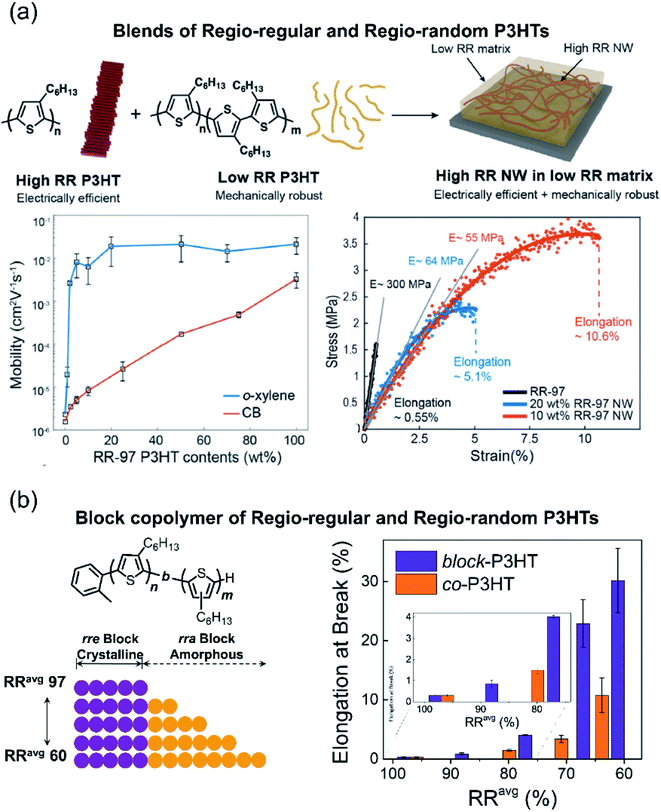 |
| Fig. 5 Flexible and stretchable electronics with the incorporation of rre P3HTs into rra P3HTs using the (a) blend and (b) block copolymer approach, respectively. Reproduced with permission.114,115 Copyright 2017, American Chemical Society, and copyright 2019, American Chemical Society. | |
2.3 RR of D–A copolymers
Most high-performance state-of-the-art CPs feature a D–A molecular configuration, where the D and A moieties are alternatively coupled along the conjugated backbones. D–A alternating polymers can afford advantageous optoelectronic properties through facile tuning of their optical bandgap, energy levels, and charge transport properties via intramolecular charge transfer (ICT) and orbital hybridization between D and A moieties. To exploit these features, numerous D–A CPs have been developed to date with remarkable improvements in their optoelectronic performance. Despite extensive studies on the molecular designs for D–A CPs, the effect of RR on D–A copolymers has been largely unexplored. In particular, the RR is now emerging as a particularly important parameter for high-performance D–A CPs since their chemical structures become more complex with larger molecular asymmetry. We examine the impact of RR on various D–A copolymers by reviewing a library of recently developed D–A type CPs with controlled RR. The CPs are classified into three types depending on the RR (directional, positional, and sequential RRs).
2.3.1 Directional RR of D–A copolymers.
The directional RR of D–A type CPs can be defined by the directional orientation of the functional groups or side chains along the conjugated backbones, as similar to the RR of P3ATs.30–34 An excellent example of controlling the directional RR of D–A copolymers was demonstrated with the PTB7-Th D–A copolymer, which is one of the most successful donor materials used in high-performance PSCs. In general, PTB7-Th is prepared by the poly-condensation reaction of the electron-rich [2,6′-4,8-di(5-ethylhexylthienyl)benzo[1,2-b:3,3-b]dithiophene] (BDTT) moiety with the electron-deficient fluoro-substituted thieno[3,4-b]thiophene (FTT) moiety.36 However, the FTT unit in PTB7-Th contains asymmetric reactive sites at the 4- and 6-positions, resulting in two distinct regio-isomers. In order to produce rre PTB7-Th, the FTT-BDTT-FTT trimer (M2) was first prepared (Fig. 6a). Polymerization of the dibromo-M2 trimer with ditrimethyltin-BDTT by Stille coupling reactions yielded rre PTB7-Th (P2). On the other hand, rra PTB7-Th (P3) was synthesized by polymerizing ditrimethyltin-BDTT and dibromo-FTT through Stille coupling condensation reactions. Thereafter, Bo et al. also demonstrated a similar method of preparing directionally rre and rra poly[(2,6-(4,8-bis(5-(2-ethylhexyl)thiophene-2-yl)-benzo[1,2-b:4,5-b′]dithiophene))-alt-(2,5-(ethyl thiophene carboxylate))] (PThE) D–A copolymers by the polymerization of symmetric D–A–D trimers and an A monomer (Fig. 6b).
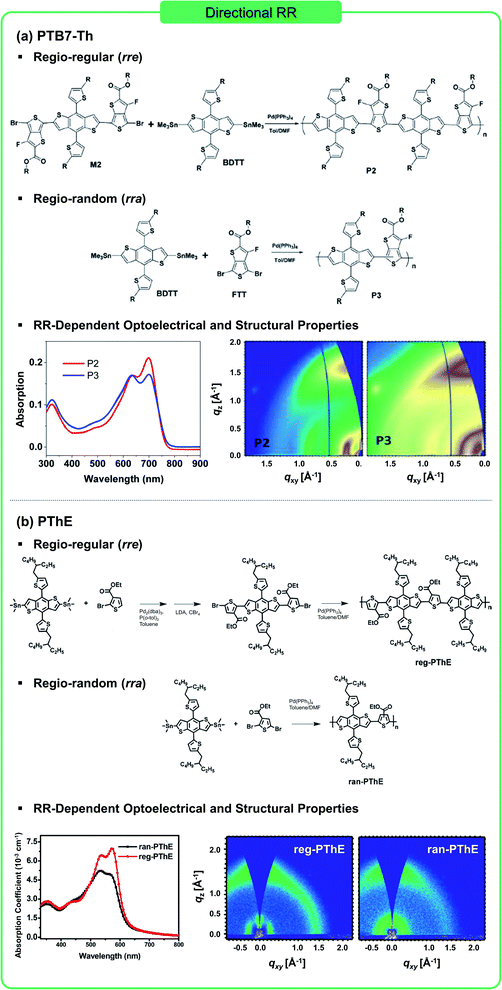 |
| Fig. 6 Influences of RR on the opto-electrical and structural properties of D–A copolymers with directional RR: (a) PTB7-Th; and (b) PThE. Reproduced with permission.30,36 Copyright 2018, American Chemical Society, and copyright 2017, Royal Society of Chemistry. | |
Comparative analysis of directionally rre and rra D–A copolymers demonstrated that the directional RR of the side chains or functional groups exerts an influence on their light absorption, packing order, and charge mobility. These structure–property relationships result from cooperative molecular arrangements of the functional side groups. These arrangements affect the noncovalent interactions with aromatic protons on the conjugated backbone and the planarity of the CP chains. For example, the absorption coefficients of rre PThE and rra PThE in thin films were estimated to be 6.98 × 103 cm−1 and 5.23 × 103 cm−1, respectively.30 Although these two polymers exhibit similar absorption ranges, their relative intensities of the peaks were different. rra PThE showed a stronger absorption peak at 532 nm and a weaker absorption peak at 573 nm, meanwhile rre PThE showed the opposite trend, indicating that rre PThE has stronger inter-chain interactions than rra PThE in thin films. The grazing incidence X-ray scattering (GIXS) pattern shows that the peaks of the lamellar structures in rre PThE disappeared in rra PThE, suggesting that the high RR induced higher crystallinity and stronger intermolecular interactions, consistent with the UV-vis spectra. Differences in the optoelectrical features of directionally rre and rra D–A copolymers have also been reported in other D–A copolymer systems.31–34 Note that directional RR dependent optoelectrical properties in D–A copolymers are less significant than the changes observed in P3ATs. Considering the small fraction of functional groups, related to directional RR in D–A copolymers compared to the whole molecule, the steric effect from RR defects within D–A copolymers is not as significant as in the case of homopolymers with asymmetric alkyl chains. Moreover, the limited Mn and high dispersity of the rre D–A copolymers originating from the step-growth polymerization methods may result in less ordered structures.
2.3.2 Positional RR of D–A copolymers.
A successful example of positional RR in D–A copolymers was demonstrated by Neher and co-workers using the regio-isomers of P(NDI2OD-T2).43 In the acceptor moiety (NDI2OD) of P(NDI2OD-T2), four different hydrogen positions can be substituted as reactive sites for the polymerization, resulting in either 2,6- or 2,7-conformations between the D and A monomer units. The key for obtaining rre P(NDI2OD-T2) is the use of N,N′-bis (2-octyldodecyl)-2,6-dibromonaphthalene-1,4,5,8-bis-(dicarboximide) (NDI2OD-2,6-Br2) as a building block (Fig. 7a). Polymerization at the 2,6-bromide on the NDI backbone leads to the formation of a regio-selective polymer in which the monomers are diagonally oriented along the chain. Meanwhile, positionally rir P(NDI2OD-T2) can be produced by using a mixture of NDI2OD-2,6-Br2 and NDI2OD-2,7-Br2. In the synthesis of rir P(NDI2OD-T2), NDI2OD-Br4 was first prepared by tetrabromination of 1,4,5,8-naphthalenetetracarboxylic dianhydride (NDA) with dibromoisocyanuric acid, which was subsequently reacted with 2-octadecylamine. Then, the tetrabromoimide system was randomly debrominated using Zn catalysts to obtain a mixture of brominated NDI (70% of NDI2OD-2,6Br2 and 30% of NDI2OD-2,7Br2). Finally, the dibromide isomer mixture was reacted with 5,5′-bis(trimethylstannyl)-2,2′-dithiophene using the Pd(PPh3)2Cl2 catalyst to obtain a positional rir copolymer.
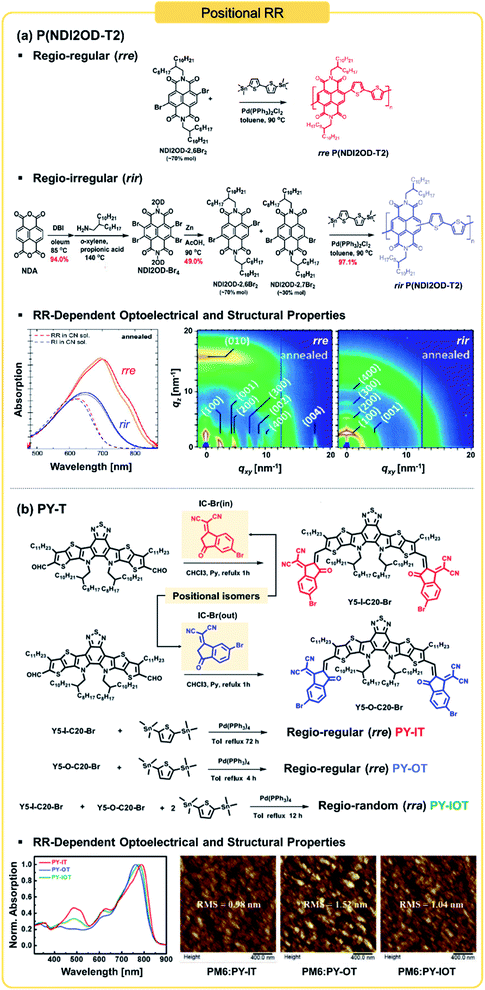 |
| Fig. 7 Influences of RR on the structural and optoelectrical properties of D–A copolymers with positional RR: (a) P(NDI2OD-T2); and (b) PY-T. Reproduced with permission.41,43 Copyright 2021, American Chemical Society, and copyright 2014, American Chemical Society. | |
Another good example of the positional RR in D–A copolymers is polymerized small-molecule-acceptors (PSMAs), a recently developed class of high-performance polymer acceptors. In these systems, non-fullerene small-molecule-acceptor (NFSMA) with multiple fused rings and strong dyes are alternatively copolymerized with donating spacers (i.e., thiophene or selenophene) to yield polymerized NFSMAs (PSMAs).39–41,117,118 The reactive bromides on different positions on the SMA monomer (i.e., 1,1-dicyanomethylene-3-indanone (IC-Br)) result in two different positional isomers of IC-Br units (IC-Br(in) and IC-Br(out)) based on their position relative to other functional groups (i.e., carbonyl and malononitrile groups) (Fig. 7b).39 Thus, the polymerization of these IC-Br SMA monomers results in three different positional-isomeric pairs (i.e., in/in, in/out, and out/out). Therefore, most early-stage PSMAs have rra arrangements along their polymer backbones. RR-controlled PSMAs were recently developed by separating out isomerically pure IC-Br units through recrystallization in different solvents (i.e., CHCl3 for IC-Br(in) and ethanol for IC-Br(out)). For example, Luo and co-workers developed two different RR-controlled PSMAs (PY-IT and PY-OT) using separated IC-Br(in) and IC-Br(out) for each polymer, and compared them with the rra PSMA (PY-IOT) containing a mixture of IC-Br units (Fig. 7b). Also, Jen and co-workers synthesized RR-controlled benzotriazole-based PSMAs (rre PZT-γ) by using separated IC-Br(in) during polymerization, and compared it with the rra PZT counterparts.41
Positional RR significantly impacts the backbone conformation of CPs by inducing tilts between the D and A units. To study these effects, Neher et al. compared the optical and structural properties of P(NDI2OD-T2) with different positional RR.43 The absorption range of positionally rre P(NDI2OD-T2) shifted toward a lower wavelength compared to that of the rir derivatives, as shown in Fig. 7a. Notably, rre P(NDI2OD-T2) produced two different aggregate species in thin-films. The intrachain aggregation was shown in the absorption peak at ∼700 nm and the interchain aggregation was found in the shoulder peak at ∼790 nm. Meanwhile, such vibronic peaks were not found in the spectrum of rir P(NDI2OD-T2), indicating that positional RR affects the intermolecular assembly of CPs. This was also supported by the GIXS plots. Two distinct crystal packing structures were observed corresponding to rre and rir P(NDI2OD-T2). Bar-shaped scattering peaks were observed in the spectrum of the rre polymer film, suggesting a long-range ordering of the crystallites. Later, RR-controlled P(NDI2OD-T2)s were demonstrated by Ludwigs and co-workers by polymerizing 2,6- and 2,7-NDI regio-isomers having various feed ratios.42 Through this system, a gradual hypochromic shift as well as decreased crystal ordering and electron mobility were observed as the positional RR decreased. In contrast to P3ATs where low RR leads to an amorphous film, rir P(NDI2OD-T2) exhibits rather unique two-dimensional ordered microstructures as it is less aggregated in the solution state. This unique feature of positional RR-controlled P(NDI2OD-T2) allows for charge transport along different crystallographic axes. In addition, the different RRs of PSMAs drove clear transitions in their optical and morphological properties. For example, rre PY-IT had a red-shifted absorption region compared to the rra PY-IOT, while the other form of rre PSMA (PY-OT) had blue-shifted absorption compared with the rra one (PY-IOT) (Fig. 7b). Also, surfaces of blend films with a polymer donor (PM6) linearly got rougher in the order of PY-IT, PY-IOT, and PY-OT, suggesting the importance of selectively controlling positional isomers when designing PSMAs.
2.3.3 Sequential RR of D–A copolymers.
Sequential RR in D–A copolymers can be defined by the fraction of identical D–A sequences in the CPs. If the D and A monomers have asymmetric molecular structures, then the H–H or H–T arrangement between D–A will induce the formation of sequential regio-isomers along the polymer backbone. Moreover, different repeating sequences can be obtained in D–A terpolymers (D–A1–D–A2) or tetrapolymers (D1–A1–D2–A2). There are several synthetic routes to control sequential RR. The first representative example is the synthesis of PDTSTTBDT consisting of dithieno[3,2-b:2′,3′-d]silole (DTS), benzo[1,2-b:4,5-b]dithiophene (BDT), and thieno[3,4-b]thiophene (TT) (Fig. 8a). rre PDTSTTBDT was synthesized by polymerizing a symmetric TT–BDT–TT trimer and a DTS monomer via Stille cross-coupling reactions. Sequentially rra PDTSTTBDT was synthesized from a mixture of TT (2 eq.), BDT (1 eq.), and DTS (1 eq.) where BDT and DTS equally compete to react with TT. As a second example, sequential RR in Ph–DPP–Ph–BDT was controlled by using a trimer.46 The rre Ph–DPP–Ph–BDT terpolymer was synthesized from a symmetric trimer (Ph–DPP–Ph) and BDT. In contrast, a rra terpolymer was obtained by polymerizing an asymmetric trimer (Ph–DPP–Th or Ph–DPP–TT) and BDT. In addition, sequentially rre D–A copolymers have been demonstrated by the self-condensation of D1–D2–A trimers, which were selectively functionalized with two different reactive groups (e.g. Br and SnMe3). One example is the copolymer of 5-fluorobenzothiadiazole (FBT), 3-(2-octyldodecyl)-thiophene (3ODT), and thiophene (T), reported by Cao and co-workers (Fig. 8b).48 To synthesize the rre polymer (2TRR), the 3ODT-FBT-T trimer was self-polymerized, ensuring an identical (D–A)n sequence within the polymer backbone where D is the 3ODT–T dimer and A is the FBT monomer. On the other hand, the rra polymer (2TRA) was synthesized from a FBT–3ODT dimer and a T monomer. The random copolymerization of 3ODT and T afforded three donors that contain mono, di, or trithiophene in a single polymer chain. Thus, regio-selective self-condensation of D–A units or incorporating symmetric trimers is key for obtaining sequentially controlled conjugated materials.
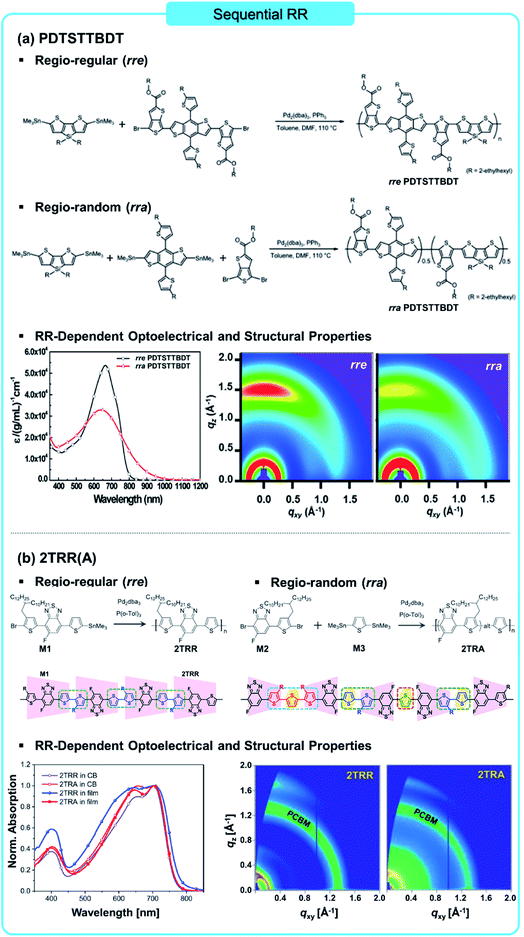 |
| Fig. 8 Influence of RR on the structural and optoelectrical properties of D–A copolymers with sequential RR: (a) PDTSTTBDT; and (b) 2TRR(A). Reproduced with permission.46,48 Copyright 2016, American Chemical Society, and copyright 2017, Wiley. | |
Compared to directional and positional RR, sequential RR typically has more significant impacts on the optoelectrical properties as the conjugations between repeating units are directly varied. In the UV-vis absorption spectra, both the absorption coefficient and wavelength are widely tuned depending on the sequential RR of the CPs.45–49 For example, rre PDTSTTBDT exhibited stronger ICT transitions than the rra counterpart, resulting in a blue shift of its absorption wavelength.46 The frontier orbitals of the rre segments extend across the entire polymer backbone, contributing to a sharp onset and narrow band of electron transition. The absorption spectra of the rra polymers are broadened due to the different sequences in the random conjugated backbone. The broadening of the absorption spectra at lower and higher wavelengths can be assigned to continuous –BDT– fragments and –DTS– fragments, respectively. Thus, the light-absorption profile of random terpolymers with various chemical compositions can be tuned over a broad wavelength range.119–125 Notably, the absorption coefficient of rre PDTSTTBDT is significantly higher than that of rra polymer, indicating the highly efficient light harvesting capabilities. In addition to its optoelectrical properties, rre PDTSTTBDT exhibited higher order peaks in the GIXS pattern compared to that of the rra analog, suggesting that the molecular ordering of the D–A copolymers can be enhanced when the sequential RR is controlled.46
3. Effects of RR on polymer solar cells (PSCs)
Among the many types of organic electronic devices, PSCs have attracted great attention as next-generation energy devices owing to their advantages such as solution processability, light weight, color tunability and mechanical flexibility.126–131 After the first-generation PSCs based on bulk heterojunction (BHJ) blends of p-type CPs as a polymer donor (PD) and n-type fullerene derivative as an electron acceptor were reported, the power conversion efficiency (PCE) of PSCs has been dramatically increased to 17–18%, driven by the development of a wide variety of CPs and NFSMAs.132–137 In particular, the advent of high-performance D–A type CPs with a capability of tunable energy levels and light absorption properties has led to remarkable improvements in the efficiency. By using these D–A copolymers as both PDs and polymer acceptors (PAs), efficient all-polymer solar cells (all-PSCs) have been achieved.2,5,137–150 Engineering of the light absorption and frontier energy levels of both PD and PA affords simultaneous enhancements of the open-circuit voltage (Voc) and short-circuit current density (Jsc), resulting in high-performance all-PSCs. Importantly, the PD and PA chains can be tie molecules and form entangled networks in the BHJ blends, thus resulting in superior mechanical/thermal stabilities and making all-PSCs as promising candidates for wearable and stretchable electronics.151–157 Moreover, recent development of A–D–A (or A–D–A′–D–A) type NFSMAs including Y-series has afforded state-of-the-art PSCs with PCEs of over 18%.158–165 However, NFSMA-based PSCs exhibit relatively poor mechanical and thermal stability. Thus, new D–A type polymer acceptors (PSMA) have been developed by polymerizing NFSMAs and other donating building blocks.39–41,117,166–181 Because different regio-isomers are found in the chemical structures of NFSMA molecules, controlling the RR of the PSMA is an emerging factor for their design and application in high-efficiency all-PSCs. In this section, we will review a library of examples of RR control in the photovoltaic polymers and summarize the impacts of directional, positional, and sequential RRs on the PSC performance.
3.1 RR of homopolymers
In early studies on PSCs, P3ATs were the prototype p-type material for the active layers in PSCs.182–185 In particular, P3HTs are often used as a model CP for BHJ PSCs for exploring general relationships between the primary structure and device function. Among several key variables, the RR of P3HTs has been identified as a primary factor for producing high-efficiency PSCs (Fig. 9). For example, Kim et al. demonstrated the strong influence of the RR on PSC performance, attributed to the enhanced optical absorption and charge transport in the well-ordered high RR P3HTs.188 Consequently, optimal device efficiencies were achieved with the highest RR P3HTs. As shown in Fig. 9a, the current–voltage curves indicated that rre P3HTs produced much higher Jsc and fill factor (FF) than those of rra P3HTs due to its much lower series resistance (RS, 3.6 Ω cm2) compared to rra P3HTs (13 Ω cm2). From the external quantum efficiency (EQE) curve, it was determined that rre P3HTs absorbed light over a wider range than rra P3HTs with higher photon-to-electrical conversion (Fig. 9b). The space-charge limited current (SCLC) measurements in Fig. 9b show that the hole mobility of rre P3HTs was almost 5 times higher than that of rra P3HTs. However, the excessive crystallization of high RR P3HTs can adversely affect the stability of the PSCs. The strong crystallization of the high RR P3HT greatly reduces its solubility and induces excessive phase separation with fullerene acceptors, decreasing the thermal and morphological stability of the PSCs.179 For example, Fréchet and co-workers reported that increasing the RR and crystallinity resulted in more severe phase separation with PCBM under persistent heating.83 As shown in Fig. 9d, P3HTs with 86% RR afforded a high PCE of over 3%, and enabled the fabrication of a thermally-stable PSC due to reduced phase separation with fullerene acceptors. In contrast, P3HTs with 96% RR exhibited the highest initial PCE, but the PCE declined significantly with exposure to thermal stress (at 150 °C). Thus, RR control is an important parameter for optimizing the blend morphology to achieve both high PCE and thermal stability of the P3HT-based PSCs.
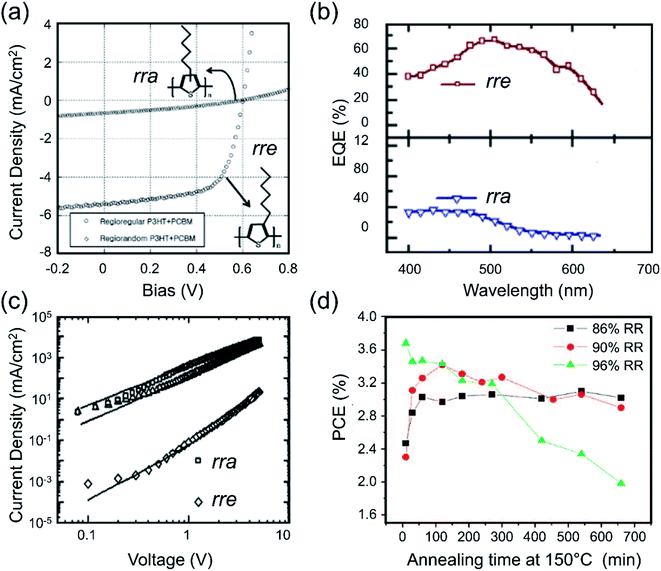 |
| Fig. 9 (a) Current density–voltage curves, (b) EQE curves, and (c) SCLC curves of BHJ-PSCs using blends of rre- and rra P3HT:PCBM. (d) Thermal stability of P3HT:PCBM PSCs with different RRs. Reproduced with permission.83,184,185 Copyright 2008, American Chemical Society, copyright 2009, Elsevier, and copyright 2009, American Chemical Society. | |
3.2 RR of D–A copolymers
3.2.1 Directional RR of D–A copolymers.
Similar to the cases of P3HT-based PSCs, the PCEs and stabilities of the PSCs based on D–A copolymers are largely affected by the directional RR. The rre polymers are more likely to aggregate and to have higher crystallinity than rra counterparts, attributed to the consistent arrangement of side chains or functional groups in the rre polymers. These features afford enhanced charge mobility and less charge recombination in the BHJ, and thus higher PCEs can be obtained from rre polymers (Fig. 10 and Table 2). For example, Lee and co-workers developed two different PD (directionally rre and rra PBDTTT-C-T) and employed them in PSCs with the PC71BM acceptor.35 The rre PBDTTT-C-T based PSCs exhibited a PCE of 7.79%, which was much higher than that of the rra PBDTTT-C-T-based PSCs. Effective molecular ordering between the polymer chains of rre PBDTTT-C-T led to enhanced charge-carrier mobility and broader light absorption. The SCLC hole mobility (μh,SCLC) of rre PBDTTT-C-T was 1.16 × 10−3 cm2 V−1 s−1, which is higher than that of rra PBDTTT-C-T (5.02 × 10−4 cm2 V−1 s−1). In addition, Bo et al. developed two PDs (rre PThE and rra PThE) consisting of benzodithiophene and ethyl 3-thiophenecarboxylate building blocks, which differed in terms of the directional RR, and employed them in the PSCs with ITTC NFSMAs.30 While rra PThE yielded a PCE of 8.38%, rre PThE afforded a significantly increased PCE of 10.14%. Notably, the rre PThE:ITTC blends exhibited a higher μh,SCLC (5.24 × 10−5 cm2 V−1 s−1) than that of the rra PThE:ITTC blends (1.12 × 10−5 cm2 V−1 s−1). The same trends were also observed for other blends with other NFSMAs (i.e., ITIC, and FTIC), where rre PThE afforded improved light absorption and charge-carrier mobilities of PThE-based PSCs. Thus, controlling the directional RR of D–A copolymers is important in optimizing the charge-carrier mobilities and PCEs of the PSCs.
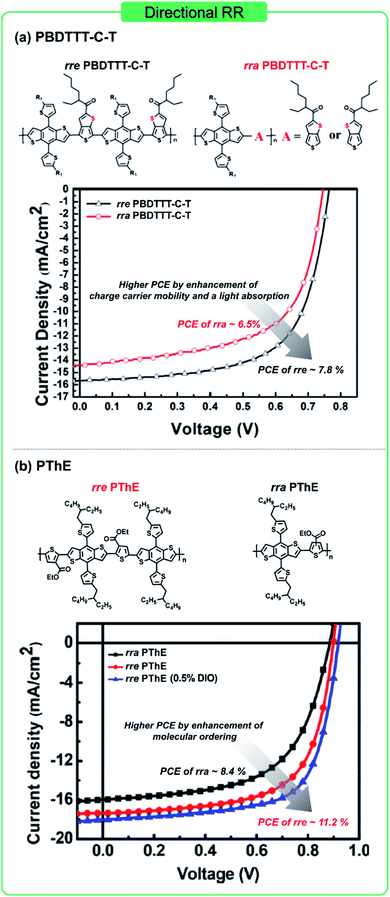 |
| Fig. 10 Influences of RR on PCEs of PSCs based on D–A copolymers with directional RR: (a) PBDTTT-C-T; and (b) PThE. Reproduced with permission.30,35 Copyright 2018, American Chemical Society, and copyright 2015, American Chemical Society. | |
Table 2 PCEs of directional rre- and rra-copolymer based PSCs
RR type |
Active layer |
RR |
PCE [%] |
μ
h,SCLC [cm2 V−1 s−1] |
μ
e,SCLC [cm2 V−1 s−1] |
Ref. |
The mobility was measured with pristine polymer films (not blend).
|
Directional |
rre PBDTTT-C-T:PC71BM |
rre
|
7.79 |
1.16 × 10−3a |
— |
35
|
rra PBDTTT-C-T:PC71BM |
rra
|
6.60 |
5.02 × 10−4a |
— |
rre PThE:ITTC |
rre
|
10.14 |
5.24 × 10−5 |
5.76 × 10−5 |
30
|
rra PThE:ITTC |
rra
|
8.38 |
1.12 × 10−5 |
2.65 × 10−5 |
P2:PC71BM |
rre
|
9.97 |
— |
9.76 × 10−3 |
36
|
P3:PC71BM |
rra
|
7.88 |
— |
7.27 × 10−3 |
PBDT-TSR:PC71BM |
rre
|
10.20 |
3.16 × 10−2a |
— |
31
|
PBDT-TS1:PC71BM |
rra
|
9.74 |
8.78 × 10−3a |
— |
PIPCP:PC61BM |
rre
|
6.13 |
1.4 × 10−4a |
— |
33
|
PIPC-RA:PC61BM |
rra
|
1.67 |
1.3 × 10−5a |
— |
rre-(D1–A–D2–A):PA |
rre
|
5.93 |
3.1 × 10−4a |
— |
32
|
rra-(D1–A–D2–A):PA |
rra
|
4.72 |
3.9 × 10−5a |
— |
PBTzT-4R:PC71BM |
rre
|
9.63 |
4.25 × 10−4 |
— |
34
|
PBTzT-4:PC71BM |
rra
|
9.36 |
2.08 × 10−4 |
— |
PBTzT-6R:PC71BM |
rre
|
9.12 |
2.44 × 10−4 |
— |
PBTzT-6:PC71BM |
rra
|
8.52 |
1.16 × 10−4 |
— |
3.2.2 Positional RR of D–A copolymers.
Similar to directional RR, positional RR control also has a huge impact on the performance of PSCs. Many examples of polymers with positional RR control can be found in all-PSC systems, where many high-performance PAs are composed of either PDI or NDI derivatives with different positional RRs (Fig. 11 and Table 3). In 2018, Zhan et al. reported two regio-isomers of P(SePDI), where alternating selenophenes were attached to either 1,6- or 1,7-positions of PDI derivatives.38 As shown in Fig. 11, the GIXS pattern of rre-P(SePDI) showed distinct scattering peaks along the out-of-plane direction, suggesting favorable face-on oriented molecular stacking within the film. In contrast, rir-P(SePDI) showed no scattering peaks, indicating an amorphous structure. The strong crystallinity of rre-P(SePDI) yielded a higher PCE of 6.2% and μe,SCLC of 5.4 × 10−4 cm2 V−1 s−1 compared with those of rir-P(SePDI) (5.3% and 3.5 × 10−4 cm2 V−1 s−1, respectively). The impacts of positional RR control in NDI-based PA were also investigated. Ludwigs et al. studied a series of P(NDIOD-T2) in which the positional RR ranged from 24 to 100%. rre P(NDIOD-T2) exhibited the highest μe,SCLC of 7 × 10−2 cm2 V−1 s−1, which was 10–100 times higher than that of rir P(NDIOD-T2). As presented above, the positionally rre CPs tend to result in a higher PCE in PSCs compared to the rra CPs. However, we note that strong aggregation of some of the highly rre CPs (i.e., P(NDI2OD-T2)) can also induce severe phase separation in the BHJ that ultimately reduces the overall PCEs.42
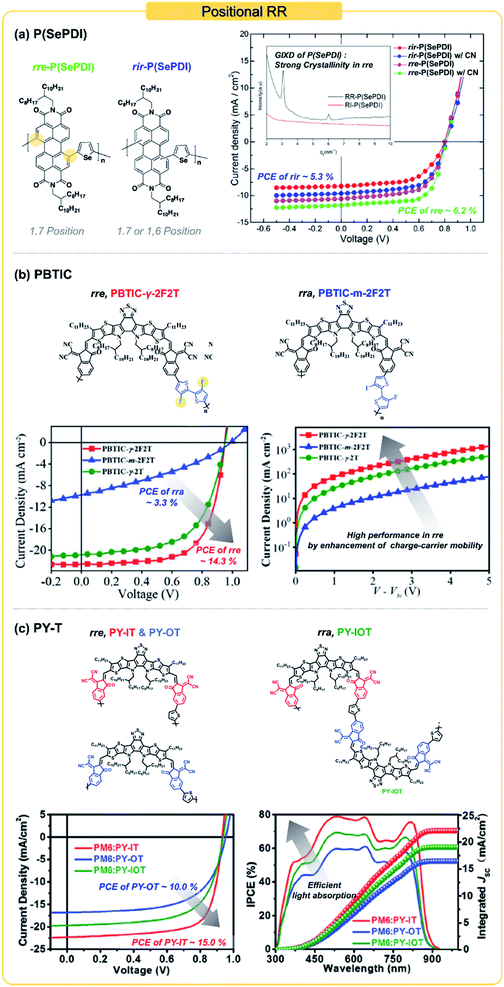 |
| Fig. 11 Examples of PSCs employing D–A copolymers with different positional RRs: (a) P(SePDI); (b) PBTIC; and (c) PY-T. Reproduced with permission.38,39,167 Copyright 2018, American Chemical Society, copyright 2020, Wiley, and copyright 2021, Wiley. | |
Table 3 PCEs of positional rre-, rir-, and rra-copolymer based PSCs
RR type |
Active layer |
RR |
PCE [%] |
μ
h,SCLC [cm2 V−1 s−1] |
μ
e,SCLC [cm2 V−1 s−1] |
Ref. |
The mobility was measured with pristine polymer films (not blend).
|
Positional |
P3HT:rre-PDI-diTh |
rre
|
2.17 |
— |
5 × 10−4a |
37
|
P3HT:rir-PDI-diTh |
rir
|
1.55 |
— |
3 × 10−4a |
PTB7-Th:rre-P(SePDI) |
rre
|
6.2 |
3.59 × 10−4 |
5.43 × 10−4 |
38
|
PTB7-Th:rir-P(SePDI) |
rir
|
5.3 |
7.25 × 10−5 |
3.51 × 10−4 |
PDCBT:RR-P(NDI2OD-T2) |
rre
|
0.75 |
— |
7 × 10−2a |
42
|
PDCBT:RI(70:30) |
rir
|
1.37 |
— |
2 × 10−3a |
PDCBT:RI(47:53) |
rir
|
2.42 |
— |
3 × 10−3a |
PDCBT:RI(24:76) |
rir
|
2.02 |
— |
9 × 10−4a |
PM6:L15 |
rre
|
15.22 |
— |
9.3 × 10−4a |
117
|
PM6:L14 |
rra
|
14.41 |
— |
8.7 × 10−4a |
PBDB-T:PZT-γ |
rre
|
15.8 |
— |
7.53 × 10−4 |
41
|
PBDB-T:PZT |
rra
|
14.5 |
— |
5.75 × 10−4 |
PM6:PY-IT |
rre
|
15.05 |
9.21 × 10−4 |
5.28 × 10−4 |
39
|
PM6:PY-OT |
rre
|
10.04 |
8.79 × 10−4 |
3.81 × 10−4 |
PM6:PY-IOT |
rra
|
12.12 |
9.03 × 10−4 |
4.57 × 10−4 |
PM6:PYF-T-o |
rre
|
15.2 |
8.4 × 10−4 |
7.8 × 10−4 |
40
|
PM6:PYF-T-m |
rre
|
1.4 |
1.2 × 10−4 |
2.7 × 10−4 |
PM6:PYF-T |
rra
|
14.0 |
7.3 × 10−4 |
6.5 × 10−4 |
Very recently, PSMAs have attracted significant attention as they combine the advantages of two distinct systems: (1) the strong light absorption and charge transport capability of NFSMAs,159,160,186 and (2) the superior mechanical robustness and morphological stability of the polymeric active layers of all-PSCs.5,151,154 Nevertheless, the PCE values of all-PSCs based on PSMAs remain lower than those of NFSMA-based PSCs. The lower PCEs are due, in part, to the inhomogeneous molecular structures with low positional RR of PSMAs.40,41,167,169,170,187 To address these issues, He and co-workers recently demonstrated the importance of controlling the positional RR of the PSMAs. They first obtained isomerically pure monomers through recrystallization and employed them to achieve rre PBTIC-γ-2F2T PSMAs by polymerization (Fig. 11b).167 The charge-carrier mobility of the resulting all-PSCs with rre PBTIC-γ-2F2T PSMAs was greatly enhanced and the voltage loss was greatly reduced, achieving a PCE of 14.34%. In contrast, the rra PBTIC-m-2F2T-based all-PSCs showed a PCE of only 3.26%. Moreover, Yang and co-workers reported rre PY-IT and rre PY-OT by polymerizing isomerically pure IC-Br(in) and IC-Br(out), respectively. The rra PY-IOT PSMAs comprising equal amounts of PY-IT and PY-OT were also compared.39 The PY-IT-based PSCs afforded the best performance (PCE = 15.05%, Table 3) as a result of their stronger interchain interaction and optimized blend morphology with PM6 PD. Interestingly, the PCE of the all-PSC device based on rre PY-OT PSMAs (10.04%) was lower than that of rre PY-IT, originating from deficient exciton dissociation, poor charge mobility, and severe charge recombination. These features were mainly driven from the curved molecular conformations of rre PY-OT PSMAs, which precluded the efficient formation of an intermolecular assembly. The rra PY-IOT PSMA-based devices exhibited an intermediate PCE of 12.12% where all photovoltaic parameters were between PY-IT and PY-OT. Thus, for the PSMA system, increasing the positional RR by using isomerically pure monomers is important. Also, controlling the molecular conformation by changing the positional RR of PSMAs is crucial for optimizing the properties of PSMAs and their performance in all-PSCs.40,167,169,170,187
3.2.3 Sequential RR of D–A copolymers.
The control of sequential RR of CPs is crucial for both optoelectrical properties and high-performance PSCs (Fig. 12 and Table 4). As an example, rra and rre PDTSTTBDT D–A copolymers were produced by random-copolymerization with (D1–A1) and (D2–A2) dimers and self-condensation of (D1–A1–D2–A2) tetramers, respectively. The PSCs employing the rre PDTSTTBDT copolymers achieved higher PCEs than the rra PDTSTTBDT-based PSCs.46 In addition, Ong and co-workers developed sequential RR-controlled D–A copolymers based on rre P2′ and rra P2′ (Fig. 12a). The rre P2′:PC71BM devices achieved a high PCE of 7.57% and μh,SCLC of 9.01 × 10−4 cm2 V−1 s−1 compared to those of rra P2′:PC71BM (4.96% and 4.80 × 10−4 cm2 V−1 s−1, respectively).45 The improved molecular packing of rre polymers effectively enhances their charge-carrier mobility and light absorption properties. In the cases of PDTSTTBDT and difluorobenzene-NDI polymers with different arrangements of the sequence of repeating units (i.e., P1′ and P2′ in Table 4), the rre polymers exhibited higher PCEs and SCLC mobilities than the rra polymers. However, sequentially rre polymers are not always beneficial for high performance PSCs. For example, Y. Cao et al. developed sequential RR-controlled polymers, 2TRR (rre) and 2TRA (rra).48 The PCE and μh,SCLC of the rra 2TRA-based PSCs were higher (8.56% and 8.6 × 10−3 cm2 V−1 s−1, respectively) than those of the rre 2TRR-based PSCs (5.05% and 5.1 × 10−4 cm2 V−1 s−1, respectively) (Fig. 12b). More distributed alkyl chains within rra 2TRR afford localized aggregates and reduce the π–π stacking distance, increasing PCEs. In addition, Saeki et al. reported two sequential regio-isomers of rre PPy and rra PPy.49 When blended with PC71BM, rra PPy-based PSCs showed better PCE (3.75%) and μh,SCLC (9.1 × 10−6 cm2 V−1 s−1) than rre PPy-based PSCs (2.56% and 6.4 × 10−6 cm2 V−1 s−1, respectively). Therefore, the suitable control of sequential RR of CPs can further optimize the optoelectrical properties of the CPs as well as the PCE in PSCs. The structures of D–A copolymers described in this section and Tables 2–4 are shown in Fig. 13.
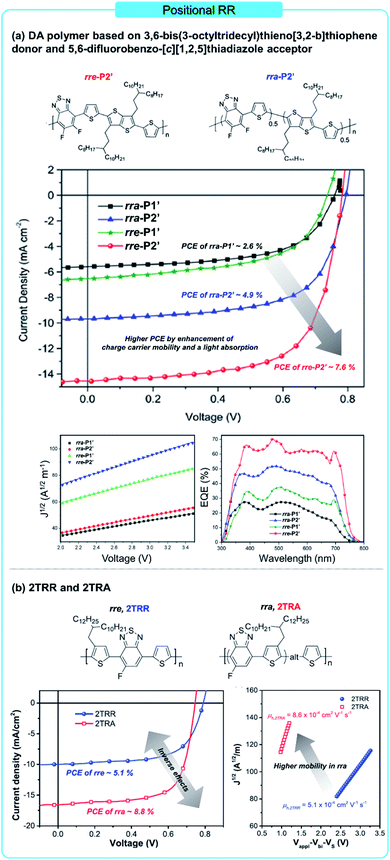 |
| Fig. 12 Influences of RR on PCEs of PSCs based on D–A copolymers having different sequential RRs: (a) DA polymer based on 3,6-bis(3-octyltridecyl)thieno[3,2-b]thiophene donor and 5,6-difluorobenzo-[c][1,2,5]thiadiazole acceptor; and (b) 2TRR and 2TRA. Reproduced with permission.45,48 Copyright 2016, American Chemical Society, and copyright 2017, Wiley. | |
Table 4 PCEs of sequential rre- and rra-copolymer based PSCs
RR type |
Active layer |
RR |
PCE [%] |
μ
h,SCLC [cm2 V−1 s−1] |
μ
e,SCLC [cm2 V−1 s−1] |
Ref. |
The mobility was measured with pristine polymer films (not blend).
|
Sequential |
rre-P1′:PC71BM |
rre
|
3.59 |
5.80 × 10−4 |
— |
45
|
rra-P1′:PC71BM |
rra
|
2.60 |
3.49 × 10−4 |
— |
rre-P2′:PC71BM |
rre
|
7.57 |
9.01 × 10−4 |
— |
rra-P2′:PC71BM |
rra
|
4.96 |
4.80 × 10−4 |
— |
rre PDTSTTBDT:PC71BM |
rre
|
6.14 |
7.0 × 10−5 |
— |
46
|
rra PDTSTTBDT:PC71BM |
rra
|
1.11 |
1.7 × 10−6 |
— |
PTB7-Th:P2′′ |
rre
|
5.20 |
2.1 × 10−4 |
1.6 × 10−4 |
47
|
PTB7-Th:P1′′ |
rra
|
1.30 |
1.3 × 10−6 |
7.3 × 10−5 |
2TRR:PC71BM |
rre
|
5.05 |
5.1 × 10−4a |
— |
48
|
2TRA:PC71BM |
rra
|
8.56 |
8.6 × 10−3a |
— |
rre PPy:PCBM |
rre
|
2.56 |
6.4 × 10−6 |
2.1 × 10−4 |
49
|
rra PPy:PCBM |
rra
|
3.75 |
9.1 × 10−6 |
8.5 × 10−4 |
P1′′′:PCBM |
rre
|
0.45 |
— |
— |
50
|
P2′′′:PCBM |
rra
|
2.30 |
— |
— |
P3′′′:PCBM |
rra
|
2.40 |
— |
— |
 |
| Fig. 13 Molecular structures of regioisomers in PSCs categorized by (a) directional RR, (b) positional RR, and (c) sequential RR which are described in Tables 2–4, respectively. | |
4. Conclusion and outlook
In this review, an overview of CPs with controlled RR, covering from simple homopolymers to state-of-the-art D–A copolymers, is presented. To provide a general guideline for RR control of a wide variety of CPs, we define three different types of RRs, i.e., directional, positional and sequential RRs. We also highlight the importance of these three types of RRs from synthesis and properties of materials to performance of PSC devices.
The definition of RR for simple homopolymers with asymmetric alkyl chains such as P3ATs has been well-established. Also, various synthetic methods have been developed, which achieve the precise control of their structure and purity. As a result, the effect of the RR of CPs on the polymer properties and device performances has been extensively explored. In general, higher RR leads to stronger molecular interactions and higher crystallinity; thus, the optical and electrical properties can be enhanced to be suitable for the devices. However, the strong crystallization of high RR CPs can often induce low solution processability, mechanical fragility, and low thermal stability in PSC blends. Therefore, precise RR control is important in the design of CPs for various organic electronic applications.
While the importance of the RR of homopolymers is relatively well-demonstrated, the RR of recently developed high-performance CPs such as D–A copolymers and PSMA has largely been unexplored due to their chemical complexity and lack of synthetic methods to control RR. However, since the state-of-the-art device performances are achieved by the D–A copolymers and PSMA, controlling the RR of these CPs is emerging as a crucial parameter for high-performance materials. From many examples of these CPs, we categorize the RR-varied polymers into three types depending on the directional, positional, and sequential regularities of the repeating units. Then, we describe synthetic methods to control each type of RR, and its impact on the optoelectrical properties of the polymers and their device performances. In directional RR, regular alignments of the functional groups and/or side chains in the same direction induces stronger molecular packing and enhanced optoelectrical properties, improving device performances compared to those afforded by the random isomers. Different positional RR induces changes in the molecular conformation due to the different tilted angles between the repeating units of the CPs. Therefore, control of positional RR can give rise to unique crystal structures. Positionally rre CPs generally exhibit excellent optoelectrical properties and enhanced performances in PSCs. Compared with directional and positional RR, differences in sequential RR induce larger impacts on the optoelectrical properties of the CPs. Sequentially rre CPs typically are more crystalline. Nevertheless, some examples show that sequentially rir CPs can result in a higher PCE of the PSCs, probably due to the inferior morphology of the rre CP-based blends driven by excessive crystallization. Therefore, delicate tuning of the sequential RR is required for achieving high-performance PSCs.
The importance of controlling the RR of D–A copolymers has been recently highlighted in the organic electronics community. Many unrevealed correlations between the RR of D–A copolymers and their properties/device performances should be elucidated. For example, tuning the mechanical properties of CPs without compromising their electrical and optical properties is of great importance for their applications in wearable and portable electronics. While RR is one of the key parameters for controlling the mechanical properties of the polymers, the impact of the RR control is largely unexplored for the high-performance D–A copolymers. Most examples of RR-controlled polymers have been found in the PSC and organic transistor applications and the studies should be extended to other electronic devices considering the huge influence of RR on various intrinsic properties of CPs.
Conflicts of interest
There are no conflicts to declare.
Acknowledgements
This work was supported by the National Research Foundation of Korea (2017M3D1A1039553 and 2020R1A4A1018516). This research is additionally performed as a cooperation project of “Basic project”, supported by the Korea Research Institute of Chemical Technology (KRICT). And, this work is also supported by (Grant No. 1425144083) the Ministry of SMEs and Startups of the Korean Government.
References
- C. Bruner and R. Dauskardt, Macromolecules, 2014, 47, 1117–1121 CrossRef CAS
.
- N. B. Kolhe, D. K. Tran, H. Lee, D. Kuzuhara, N. Yoshimoto, T. Koganezawa and S. A. Jenekhe, ACS Energy Lett., 2019, 4, 1162–1170 CrossRef CAS
.
- J. Chen, X. Zhuang, W. Huang, M. Su, L.-w. Feng, S. M. Swick, G. Wang, Y. Chen, J. Yu, X. Guo, T. J. Marks and A. Facchetti, Chem. Mater., 2020, 32, 5317–5326 CrossRef CAS
.
- S. Samal and B. C. Thompson, ACS Macro Lett., 2018, 7, 1161–1167 CrossRef CAS
.
- C. Lee, S. Lee, G.-U. Kim, W. Lee and B. J. Kim, Chem. Rev., 2019, 119, 8028–8086 CrossRef CAS PubMed
.
- Y. Zhang, D. Hanifi, E. Lim, S. Chourou, S. Alvarez, A. Pun, A. Hexemer, B. Ma and Y. Liu, Adv. Mater., 2014, 26, 1223–1228 CrossRef CAS PubMed
.
- M. Kim, S. U. Ryu, S. A. Park, K. Choi, T. Kim, D. Chung and T. Park, Adv. Funct. Mater., 2020, 30, 1904545 CrossRef CAS
.
- J. Z. Low, B. Capozzi, J. Cui, S. Wei, L. Venkataraman and L. M. Campos, Chem. Sci., 2017, 8, 3254–3259 RSC
.
- M. Kim, H. I. Kim, S. U. Ryu, S. Y. Son, S. A. Park, N. Khan, W. S. Shin, C. E. Song and T. Park, Chem. Mater., 2019, 31, 5047–5055 CrossRef CAS
.
- S.-I. Na, Y.-H. Seo, Y.-C. Nah, S.-S. Kim, H. Heo, J.-E. Kim, N. Rolston, R. H. Dauskardt, M. Gao, Y. Lee and D. Vak, Adv. Funct. Mater., 2019, 29, 1805825 CrossRef
.
- N. B. Kolhe, H. Lee, D. Kuzuhara, N. Yoshimoto, T. Koganezawa and S. A. Jenekhe, Chem. Mater., 2018, 30, 6540–6548 CrossRef CAS
.
- Y.-J. Hwang, H. Li, B. A. E. Courtright, S. Subramaniyan and S. A. Jenekhe, Adv. Mater., 2016, 28, 124–131 CrossRef CAS PubMed
.
- Y. Wang, T. Hasegawa, H. Matsumoto and T. Michinobu, J. Am. Chem. Soc., 2019, 141, 3566–3575 CrossRef CAS PubMed
.
- E. Wang, W. Mammo and M. R. Andersson, Adv. Mater., 2014, 26, 1801–1826 CrossRef CAS PubMed
.
- A. X. Chen, A. T. Kleinschmidt, K. Choudhary and D. J. Lipomi, Chem. Mater., 2020, 32, 7582–7601 CrossRef CAS
.
- E. L. Melenbrink, K. M. Hilby, K. Choudhary, S. Samal, N. Kazerouni, J. L. McConn, D. J. Lipomi and B. C. Thompson, ACS Appl. Mater. Interfaces, 2019, 1, 1107–1117 CAS
.
- S. W. Kim, Y. Wang, H. You, W. Lee, T. Michinobu and B. J. Kim, ACS Appl. Mater. Interfaces, 2019, 11, 35896–35903 CrossRef CAS PubMed
.
- R. Ma, T. Liu, Z. Luo, K. Gao, K. Chen, G. Zhang, W. Gao, Y. Xiao, T.-K. Lau, Q. Fan, Y. Chen, L.-K. Ma, H. Sun, G. Cai, T. Yang, X. Lu, E. Wang, C. Yang, A. K. Y. Jen and H. Yan, ACS Energy Lett., 2020, 5, 2711–2720 CrossRef CAS
.
- L. Ying, F. Huang and G. C. Bazan, Nat. Commun., 2017, 8, 14047 CrossRef CAS PubMed
.
- I. Osaka and R. D. McCullough, Acc. Chem. Res., 2008, 41, 1202–1214 CrossRef CAS PubMed
.
- B. Amna, H. M. Siddiqi, A. Hassan and T. Ozturk, RSC Adv., 2020, 10, 4322–4396 RSC
.
- B. Kuei and E. D. Gomez, Soft Matter, 2017, 13, 49–67 RSC
.
- A. R. Aiyar, J.-I. Hong and E. Reichmanis, Chem. Mater., 2012, 24, 2845–2853 CrossRef CAS
.
- C. R. Snyder and E. D. Gomez, J. Polym. Sci., Part B: Polym. Phys., 2016, 54, 1202–1206 CrossRef CAS PubMed
.
- J.-S. Kim, J.-H. Kim, W. Lee, H. Yu, H. J. Kim, I. Song, M. Shin, J. H. Oh, U. Jeong and T.-S. Kim, Macromolecules, 2015, 48, 4339–4346 CrossRef CAS
.
- M. Baghgar, J. Labastide, F. Bokel, I. Dujovne, A. McKenna, A. M. Barnes, E. Pentzer, T. Emrick, R. Hayward and M. D. Barnes, J. Phys. Chem. Lett., 2012, 3, 1674–1679 CrossRef CAS PubMed
.
- P. Kohn, S. Huettner, H. Komber, V. Senkovskyy, R. Tkachov, A. Kiriy, R. H. Friend, U. Steiner, W. T. S. Huck, J.-U. Sommer and M. Sommer, J. Am. Chem. Soc., 2012, 134, 4790–4805 CrossRef CAS PubMed
.
- S. Pankaj, E. Hempel and M. Beiner, Macromolecules, 2009, 42, 716–724 CrossRef CAS
.
- S. Qu, C. Ming, Q. Yao, W. Lu, K. Zeng, W. Shi, X. Shi, C. Uher and L. Chen, Polymers, 2018, 10, 815 CrossRef PubMed
.
- Y. Liu, H. Lu, M. Li, Z. Zhang, S. Feng, X. Xu, Y. Wu and Z. Bo, Macromolecules, 2018, 51, 8646–8651 CrossRef CAS
.
- H. Yao, W. Zhao, Z. Zheng, Y. Cui, J. Zhang, Z. Wei and J. Hou, J. Mater. Chem. A, 2016, 4, 1708–1713 RSC
.
- S. W. Kim, H. Kim, J.-W. Lee, C. Lee, B. Lim, J. Lee, Y. Lee and B. J. Kim, Macromolecules, 2019, 52, 738–746 CrossRef CAS
.
- M. Wang, H. Wang, T. Yokoyama, X. Liu, Y. Huang, Y. Zhang, T.-Q. Nguyen, S. Aramaki and G. C. Bazan, J. Am. Chem. Soc., 2014, 136, 12576–12579 CrossRef CAS PubMed
.
- D. Zhu, Q. Wang, Y. Wang, X. Bao, M. Qiu, B. Shahid, Y. Li and R. Yang, Chem. Mater., 2018, 30, 4639–4645 CrossRef CAS
.
- H. Kim, H. Lee, D. Seo, Y. Jeong, K. Cho, J. Lee and Y. Lee, Chem. Mater., 2015, 27, 3102–3107 CrossRef CAS
.
- H. Zhong, L. Ye, J.-Y. Chen, S. B. Jo, C.-C. Chueh, J. H. Carpenter, H. Ade and A. K. Y. Jen, J. Mater. Chem. A, 2017, 5, 10517–10525 RSC
.
- Y. Zhou, Q. Yan, Y.-Q. Zheng, J.-Y. Wang, D. Zhao and J. Pei, J. Mater. Chem. A, 2013, 1, 6609–6613 RSC
.
- Y. Liang, S. Lan, P. Deng, D. Zhou, Z. Guo, H. Chen and H. Zhan, ACS Appl. Mater. Interfaces, 2018, 10, 32397–32403 CrossRef CAS PubMed
.
- Z. Luo, T. Liu, R. Ma, Y. Xiao, L. Zhan, G. Zhang, H. Sun, F. Ni, G. Chai, J. Wang, C. Zhong, Y. Zou, X. Guo, X. Lu, H. Chen, H. Yan and C. Yang, Adv. Mater., 2020, 32, 2005942 CrossRef CAS PubMed
.
- H. Yu, M. Pan, R. Sun, I. Agunawela, J. Zhang, Y. Li, Z. Qi, H. Han, X. Zou, W. Zhou, S. Chen, J. Y. L. Lai, S. Luo, Z. Luo, D. Zhao, X. Lu, H. Ade, F. Huang, J. Min and H. Yan, Angew. Chem., Int. Ed., 2021, 60, 10137–10146 CrossRef CAS PubMed
.
- H. Fu, Y. Li, J. Yu, Z. Wu, Q. Fan, F. Lin, H. Y. Woo, F. Gao, Z. Zhu and A. K. Y. Jen, J. Am. Chem. Soc., 2021, 143, 2665–2670 CrossRef CAS PubMed
.
- Y. M. Gross, D. Trefz, R. Tkachov, V. Untilova, M. Brinkmann, G. L. Schulz and S. Ludwigs, Macromolecules, 2017, 50, 5353–5366 CrossRef CAS
.
- R. Steyrleuthner, R. Di Pietro, B. A. Collins, F. Polzer, S. Himmelberger, M. Schubert, Z. Chen, S. Zhang, A. Salleo, H. Ade, A. Facchetti and D. Neher, J. Am. Chem. Soc., 2014, 136, 4245–4256 CrossRef CAS PubMed
.
- S. Wang, D. Fazzi, Y. Puttisong, M. J. Jafari, Z. Chen, T. Ederth, J. W. Andreasen, W. M. Chen, A. Facchetti and S. Fabiano, Chem. Mater., 2019, 31, 3395–3406 CrossRef CAS PubMed
.
- P. Deng, B. Wu, Y. Lei, H. Cao and B. S. Ong, Macromolecules, 2016, 49, 2541–2548 CrossRef CAS
.
- H. Heo, H. Kim, D. Lee, S. Jang, L. Ban, B. Lim, J. Lee and Y. Lee, Macromolecules, 2016, 49, 3328–3335 CrossRef CAS
.
- P. Deng, C. H. Y. Ho, Y. Lu, H.-W. Li, S.-W. Tsang, S. K. So and B. S. Ong, Commun. Chem., 2017, 53, 3249–3252 RSC
.
- C. Zhou, Z. Chen, G. Zhang, C. McDowell, P. Luo, X. Jia, M. J. Ford, M. Wang, G. C. Bazan, F. Huang and Y. Cao, Adv. Energy Mater., 2018, 8, 1701668 CrossRef
.
- K. Aoshima, M. Nomura and A. Saeki, ACS Omega, 2019, 4, 15645–15652 CrossRef CAS PubMed
.
- K. Aoshima, M. Ide and A. Saeki, RSC Adv., 2018, 8, 30201–30206 RSC
.
- M. Kobayashi, J. Chen, T. C. Chung, F. Moraes, A. J. Heeger and F. Wudl, Synth. Met., 1984, 9, 77–86 CrossRef CAS
.
- R. D. McCullough and S. P. Williams, J. Am. Chem. Soc., 1993, 115, 11608–11609 CrossRef CAS
.
- R. L. Elsenbaumer, K. Y. Jen and R. Oboodi, Synth. Met., 1986, 15, 169–174 CrossRef CAS
.
- R. S. Maior, K. Hinkelmann, H. Eckert and F. Wudl, Macromolecules, 1990, 23, 1268–1279 CrossRef CAS
.
- M. Leclerc, F. M. Diaz and G. Wegner, Makromol. Chem., 1989, 190, 3105–3116 CrossRef CAS
.
- S. Amou, O. Haba, K. Shirato, T. Hayakawa, M. Ueda, K. Takeuchi and M. Asai, J. Polym. Sci., Part A: Polym. Chem., 1999, 37, 1943–1948 CrossRef CAS
.
- R. D. Mccullough, S. P. Williams, S. Tristramnagle, M. Jayaraman, P. C. Ewbank and L. Miller, Synth. Met., 1995, 69, 279–282 CrossRef CAS
.
- R. D. Mccullough, R. D. Lowe, M. Jayaraman and D. L. Anderson, J. Org. Chem., 1993, 58, 904–912 CrossRef CAS
.
- R. D. Mccullough, S. Tristramnagle, S. P. Williams, R. D. Lowe and M. Jayaraman, J. Am. Chem. Soc., 1993, 115, 4910–4911 CrossRef CAS
.
- R. D. Mccullough, R. D. Lowe, M. Jayaraman and D. L. Anderson, J. Org. Chem., 1993, 58, 904–912 CrossRef CAS
.
- T. A. Chen, X. M. Wu and R. D. Rieke, J. Am. Chem. Soc., 1995, 117, 233–244 CrossRef CAS
.
- T. A. Chen and R. D. Rieke, J. Am. Chem. Soc., 1992, 114, 10087–10088 CrossRef CAS
.
- R. S. Loewe, S. M. Khersonsky and R. D. McCullough, Adv. Mater., 1999, 11, 250–253 CrossRef CAS
.
- E. E. Sheina, J. S. Liu, M. C. Iovu, D. W. Laird and R. D. McCullough, Macromolecules, 2004, 37, 3526–3528 CrossRef CAS
.
- R. Miyakoshi, A. Yokoyama and T. Yokozawa, J. Am. Chem. Soc., 2005, 127, 17542–17547 CrossRef CAS PubMed
.
- M. Jeffries-El, G. Sauve and R. D. McCullough, Adv. Mater., 2004, 16, 1017–1019 CrossRef CAS
.
- M. Jeffries-El, G. Sauve and R. D. McCullough, Macromolecules, 2005, 38, 10346–10352 CrossRef CAS
.
- H. C. Moon, D. Bae and J. K. Kim, Macromolecules, 2012, 45, 5201–5207 CrossRef CAS
.
- V. Ho, B. W. Boudouris, B. L. McCulloch, C. G. Shuttle, M. Burkhardt, M. L. Chabinyc and R. A. Segalman, J. Am. Chem. Soc., 2011, 133, 9270–9273 CrossRef CAS PubMed
.
- G. Barker, J. Mater. Chem., 1998, 8, 25–29 RSC
.
- S. Guillerez and G. Bidan, Synth. Met., 1998, 93, 123–126 CrossRef CAS
.
- Y. Qiu, J. C. Worch, A. Fortney, C. Gayathri, R. R. Gil and K. J. T. Noonan, Macromolecules, 2016, 49, 4757 CrossRef CAS
.
- B. C. Thompson, B. J. Kim, D. F. Kavulak, K. Sivula, C. Mauldin and J. M. J. Fréchet, Macromolecules, 2007, 40, 7425–7428 CrossRef CAS
.
- J.-S. Kim, J.-E. Choi, H. Park, Y. Kim, H. J. Kim, J. Han, J. M. Shin and B. J. Kim, Polym. Chem., 2019, 10, 3030–3039 RSC
.
- T. Menda, T. Mori and T. Yasuda, Polym. J., 2021, 53, 403–408 CrossRef CAS
.
- N. S. Gobalasingham, S. Noh and B. C. Thompson, Polym. Chem., 2016, 7, 1623 RSC
.
- B. McCulloch, V. Ho, M. Hoarfrost, C. Stanley, C. Do, W. T. Heller and R. A. Segalman, Macromolecules, 2013, 46, 1899–1907 CrossRef CAS
.
- P. Willot, J. Steverlynck, D. Moerman, P. Leclere, R. Lazzaroni and G. Koeckelberghs, Polym. Chem., 2013, 4, 2662–2671 RSC
.
- T. Adachi, J. Brazard, R. J. Ono, B. Hanson, M. C. Traub, Z. Q. Wu, Z. C. Li, J. C. Bolinger, V. Ganesan, C. W. Bielawski, D. A. V. Bout and P. F. Barbara, J. Phys. Chem. Lett., 2011, 2, 1400–1404 CrossRef CAS
.
- S. Pal and A. K. Nandi, Macromolecules, 2003, 36, 8426–8432 CrossRef CAS
.
- H. Sirringhaus, P. J. Brown, R. H. Friend, M. M. Nielsen, K. Bechgaard, B. M. W. Langeveld-Voss, A. J. H. Spiering, R. A. J. Janssen, E. W. Meijer, P. Herwig and D. M. de Leeuw, Nature, 1999, 401, 685–688 CrossRef CAS
.
- K. Zhou, J. G. Liu, R. Zhang, Q. Q. Zhao, X. X. Cao, X. H. Yu, R. B. Xing and Y. C. Han, Polymer, 2016, 86, 105–112 CrossRef CAS
.
- C. H. Woo, B. C. Thompson, B. J. Kim, M. F. Toney and J. M. J. Frechet, J. Am. Chem. Soc., 2008, 130, 16324–16329 CrossRef CAS PubMed
.
- L. Qiu, W. H. Lee, X. Wang, J. S. Kim, J. A. Lim, D. Kwak, S. Lee and K. Cho, Adv. Mater., 2009, 21, 1349–1353 CrossRef CAS
.
- K. A. Mazzio, A. H. Rice, M. M. Durban and C. K. Luscombe, J. Phys. Chem. C, 2015, 119, 14911–14918 CrossRef CAS
.
- H. J. Kim, J.-S. Kim, Y. Kim, Y. S. Jung, B. J. Kim and Y. Kim, Polymer, 2019, 178, 121569 CrossRef CAS
.
- J.-S. Kim, J. Han, Y. Kim, H. Park, J. P. Coote, G. E. Stein and B. J. Kim, Macromolecules, 2018, 51, 4077–4084 CrossRef CAS
.
- J.-S. Kim, Y. Kim, H.-J. Kim, H. J. Kim, H. Yang, Y. S. Jung, G. E. Stein and B. J. Kim, Macromolecules, 2017, 50, 1902–1908 CrossRef CAS
.
- Y. Kim, H. J. Kim, J.-S. Kim, H. Yun, H. Park, J. Han and B. J. Kim, Chem. Mater., 2018, 30, 7912–7921 CrossRef CAS
.
- C. A. Sandstedt, R. D. Rieke and C. J. Eckhardt, Chem. Mater., 1995, 7, 1057–1059 CrossRef CAS
.
- P. J. Brown, D. S. Thomas, A. Kohler, J. S. Wilson, J. S. Kim, C. M. Ramsdale, H. Sirringhaus and R. H. Friend, Phys. Rev. B: Condens. Matter Mater. Phys., 2003, 67, 064203 CrossRef
.
- L. Brambilla, J. S. Kim, B. J. Kim, V. Hernandez, J. T. L. Navarrete and G. Zerbi, J. Mol. Struct., 2020, 1221, 128882 CrossRef CAS
.
- J. Clark, J.-F. Chang, F. C. Spano, R. H. Friend and C. Silva, Appl. Phys. Lett., 2009, 94, 163306 CrossRef
.
- C. Carach, I. Riisness and M. J. Gordon, Appl. Phys. Lett., 2012, 101, 083302 CrossRef
.
- O. J. Korovyanko, R. Osterbacka, X. M. Jiang, Z. V. Vardeny and R. A. J. Janssen, Phys. Rev. B: Condens. Matter Mater. Phys., 2001, 64, 235122 CrossRef
.
- R. Mauer, M. Kastler and F. Laquai, Adv. Funct. Mater., 2010, 20, 2085–2092 CrossRef CAS
.
- F. C. Grozema, P. T. van Duijnen, Y. A. Berlin, M. A. Ratner and L. D. A. Siebbeles, J. Phys. Chem. B, 2002, 106, 7791–7795 CrossRef CAS
.
- C. Poelking and D. Andrienko, Macromolecules, 2013, 46, 8941–8956 CrossRef CAS
.
- W. Takashima, S. S. Pandey, T. Endo, M. Rikukawa and K. Kaneto, Curr. Appl. Phys., 2001, 1, 90–97 CrossRef
.
- D. H. Kim, J. T. Han, Y. D. Park, Y. Jang, J. H. Cho, M. Hwang and K. Cho, Adv. Mater., 2006, 18, 719–723 CrossRef CAS
.
- S. K. Patra, R. Ahmed, G. R. Whittell, D. J. Lunn, E. L. Dunphy, M. A. Winnik and I. Manners, J. Am. Chem. Soc., 2011, 133, 8842–8845 CrossRef CAS PubMed
.
- C. Yang, J. K. Lee, A. J. Heeger and F. Wudl, J. Mater. Chem., 2009, 19, 5416–5423 RSC
.
- E. J. W. Crossland, K. Tremel, F. Fischer, K. Rahimi, G. Reiter, U. Steiner and S. Ludwigs, Adv. Mater., 2012, 24, 839–844 CrossRef CAS PubMed
.
- P.-T. Wu, H. Xin, F. S. Kim, G. Ren and S. A. Jenekhe, Macromolecules, 2009, 42, 8817–8826 CrossRef CAS
.
- A. D. Printz and D. J. Lipomi, Appl. Phys. Rev., 2016, 3, 021302 Search PubMed
.
- G.-J. N. Wang, A. Gasperini and Z. Bao, Adv. Electron. Mater., 2018, 4, 1700429 CrossRef
.
- H.-C. Wu, S. J. Benight, A. Chortos, W.-Y. Lee, J. Mei, J. W. F. To, C. Lu, M. He, J. B. H. Tok, W.-C. Chen and Z. Bao, Chem. Mater., 2014, 26, 4544–4551 CrossRef CAS
.
- R. Peng, B. Pang, D. Hu, M. Chen, G. Zhang, X. Wang, H. Lu, K. Cho and L. Qiu, J. Mater. Chem. C, 2015, 3, 3599–3606 RSC
.
- Z. C. Smith, Z. M. Wright, A. M. Arnold, G. Sauvé, R. D. McCullough and S. A. Sydlik, Adv. Electron. Mater., 2017, 3, 1600316 CrossRef
.
- J. I. Scott, X. Xue, M. Wang, R. J. Kline, B. C. Hoffman, D. Dougherty, C. Zhou, G. Bazan and B. T. O'Connor, ACS Appl. Mater. Interfaces, 2016, 8, 14037–14045 CrossRef CAS PubMed
.
- D. Choi, H. Kim, N. Persson, P.-H. Chu, M. Chang, J.-H. Kang, S. Graham and E. Reichmanis, Chem. Mater., 2016, 28, 1196–1204 CrossRef CAS
.
- J. Xu, S. Wang, G. Wang, C. Zhu, S. Luo, L. Jin, X. Gu, S. Chen, V. R. Feig, J. W. F. To, S. Rondeau-Gagné, J. Park, B. Schroeder, C. Lu, J. Y. Oh, Y. Wang, Y.-H. Kim, H. Yan, R. Sinclair, D. Zhou, G. Xue, B. Murmann, C. Linder, W. Cai, J. B.-H. Tok, J. W. Chung and Z. Bao, Science, 2017, 355, 59–64 CrossRef CAS PubMed
.
- D. J. Lipomi, B. C. K. Tee, M. Vosgueritchian and Z. Bao, Adv. Mater., 2011, 23, 1771–1775 CrossRef CAS PubMed
.
- H. Park, B. S. Ma, J. S. Kim, Y. Kim, H. J. Kim, D. Kim, H. Yun, J. Han, F. S. Kim, T. S. Kim and B. J. Kim, Macromolecules, 2019, 52, 7721–7730 CrossRef CAS
.
- H. J. Kim, M. Y. Lee, J. S. Kim, J. H. Kim, H. Yu, H. Yun, K. Liao, T. S. Kim, J. H. Oh and B. J. Kim, ACS Appl. Mater. Interfaces, 2017, 9, 14120–14128 CrossRef CAS PubMed
.
- P. H. Chu, G. Wang, B. Y. Fu, D. Choi, J. O. Park, M. Srinivasarao and E. Reichmanis, Adv. Electron. Mater., 2016, 2, 1500384 CrossRef
.
- H. Sun, B. Liu, Y. Ma, J.-W. Lee, J. Yang, J. Wang, Y. Li, B. Li, K. Feng, Y. Shi, B. Zhang, D. Han, H. Meng, L. Niu, B. J. Kim, Q. Zheng and X. Guo, Adv. Mater., 2021, 33, 2102635 CrossRef CAS PubMed
.
- S. Seo, C. Sun, J.-W. Lee, S. Lee, D. Lee, C. Wang, T. N.-L. Phan, G.-U. Kim, S. Cho, Y.-H. Kim and B. J. Kim, Adv. Funct. Mater., 2021, 2108508 CrossRef
.
- K.-H. Kim, S. Park, H. Yu, H. Kang, I. Song, J. H. Oh and B. J. Kim, Chem. Mater., 2014, 26, 6963–6970 CrossRef CAS
.
- T. E. Kang, K.-H. Kim and B. J. Kim, J. Mater. Chem. A, 2014, 2, 15252–15267 RSC
.
- T. E. Kang, H.-H. Cho, H. j. Kim, W. Lee, H. Kang and B. J. Kim, Macromolecules, 2013, 46, 6806–6813 CrossRef CAS
.
- Z. Genene, J. Wang, X. Xu, R. Yang, W. Mammo and E. Wang, RSC Adv., 2017, 7, 17959–17967 RSC
.
- D. Chen, S. Liu, J. Liu, J. Han, L. Chen and Y. Chen, ACS Appl. Mater. Interfaces, 2021, 3, 1923–1931 CAS
.
- D. Dang, D. Yu and E. Wang, Adv. Mater., 2019, 31, 1807019 CrossRef PubMed
.
- J. Wu, G. Li, J. Fang, X. Guo, L. Zhu, B. Guo, Y. Wang, G. Zhang, L. Arunagiri, F. Liu, H. Yan, M. Zhang and Y. Li, Nat. Commun., 2020, 11, 4612 CrossRef CAS PubMed
.
- O. Inganäs, Adv. Mater., 2018, 30, 1800388 CrossRef PubMed
.
- P. P. Khlyabich, M. Sezen-Edmonds, J. B. Howard, B. C. Thompson and Y.-L. Loo, ACS Energy Lett., 2017, 2, 2149–2156 CrossRef CAS
.
- B. C. Thompson and J. M. J. Fréchet, Angew. Chem., Int. Ed., 2008, 47, 58–77 CrossRef CAS PubMed
.
- B. Kippelen and J.-L. Brédas, Energy Environ. Sci., 2009, 2, 251–261 RSC
.
- K. A. Mazzio and C. K. Luscombe, Chem. Soc. Rev., 2015, 44, 78–90 RSC
.
- C. J. Brabec, S. Gowrisanker, J. J. M. Halls, D. Laird, S. Jia and S. P. Williams, Adv. Mater., 2010, 22, 3839–3856 CrossRef CAS PubMed
.
- Z. Luo, R. Ma, T. Liu, J. Yu, Y. Xiao, R. Sun, G. Xie, J. Yuan, Y. Chen, K. Chen, G. Chai, H. Sun, J. Min, J. Zhang, Y. Zou, C. Yang, X. Lu, F. Gao and H. Yan, Joule, 2020, 4, 1236–1247 CrossRef CAS
.
- L. Zhan, S. Li, T.-K. Lau, Y. Cui, X. Lu, M. Shi, C.-Z. Li, H. Li, J. Hou and H. Chen, Energy Environ. Sci., 2020, 13, 635–645 RSC
.
- Y. Lin, J. Wang, Z.-G. Zhang, H. Bai, Y. Li, D. Zhu and X. Zhan, Adv. Mater., 2015, 27, 1170–1174 CrossRef CAS PubMed
.
- S. Zhang, Y. Qin, J. Zhu and J. Hou, Adv. Mater., 2018, 30, 1800868 CrossRef PubMed
.
- C. Sun, F. Pan, H. Bin, J. Zhang, L. Xue, B. Qiu, Z. Wei, Z.-G. Zhang and Y. Li, Nat. Commun., 2018, 9, 743 CrossRef PubMed
.
- V. Vohra, K. Kawashima, T. Kakara, T. Koganezawa, I. Osaka, K. Takimiya and H. Murata, Nat. Photonics, 2015, 9, 403–408 CrossRef CAS
.
- G. Wang, F. S. Melkonyan, A. Facchetti and T. J. Marks, Angew. Chem., Int. Ed., 2019, 58, 4129–4142 CrossRef CAS PubMed
.
- A. Facchetti, Mater. Today, 2013, 16, 123–132 CrossRef CAS
.
- Z. Genene, W. Mammo, E. Wang and M. R. Andersson, Adv. Mater., 2019, 31, 1807275 CrossRef PubMed
.
- Z. Li, L. Ying, P. Zhu, W. Zhong, N. Li, F. Liu, F. Huang and Y. Cao, Energy Environ. Sci., 2019, 12, 157–163 RSC
.
- R. Zhao, N. Wang, Y. Yu and J. Liu, Chem. Mater., 2020, 32, 1308–1314 CrossRef CAS
.
- Y.-J. Hwang, B. A. E. Courtright, A. S. Ferreira, S. H. Tolbert and S. A. Jenekhe, Adv. Mater., 2015, 27, 4578–4584 CrossRef CAS PubMed
.
- H. Yao, F. Bai, H. Hu, L. Arunagiri, J. Zhang, Y. Chen, H. Yu, S. Chen, T. Liu, J. Y. L. Lai, Y. Zou, H. Ade and H. Yan, ACS Energy Lett., 2019, 4, 417–422 CrossRef CAS
.
- H. Sun, B. Liu, C. W. Koh, Y. Zhang, J. Chen, Y. Wang, P. Chen, B. Tu, M. Su, H. Wang, Y. Tang, Y. Shi, H. Y. Woo and X. Guo, Adv. Funct. Mater., 2019, 29, 1903970 CrossRef CAS
.
- S. Chen, Y. An, G. K. Dutta, Y. Kim, Z.-G. Zhang, Y. Li and C. Yang, Adv. Funct. Mater., 2017, 27, 1603564 CrossRef
.
- J.-W. Lee, C. Sun, B. S. Ma, H. J. Kim, C. Wang, J. M. Ryu, C. Lim, T.-S. Kim, Y.-H. Kim, S.-K. Kwon and B. J. Kim, Adv. Energy Mater., 2021, 11, 2003367 CrossRef CAS
.
- R. Zhao, J. Liu and L. Wang, Acc. Chem. Res., 2020, 53, 1557–1567 CrossRef CAS PubMed
.
- D. K. Tran, A. Robitaille, I. J. Hai, X. Ding, D. Kuzuhara, T. Koganezawa, Y.-C. Chiu, M. Leclerc and S. A. Jenekhe, J. Mater. Chem. A, 2020, 8, 21070–21083 RSC
.
- H. Benten, T. Nishida, D. Mori, H. Xu, H. Ohkita and S. Ito, Energy Environ. Sci., 2016, 9, 135–140 RSC
.
- H. Kang, W. Lee, J. Oh, T. Kim, C. Lee and B. J. Kim, Acc. Chem. Res., 2016, 49, 2424–2434 CrossRef CAS PubMed
.
- T. Kim, J.-H. Kim, T. E. Kang, C. Lee, H. Kang, M. Shin, C. Wang, B. Ma, U. Jeong, T.-S. Kim and B. J. Kim, Nat. Commun., 2015, 6, 8547 CrossRef CAS PubMed
.
- P. B. J. St. Onge, M. U. Ocheje, M. Selivanova and S. Rondeau-Gagné, Chem. Rec., 2019, 19, 1008–1027 CrossRef PubMed
.
- N. Balar, J. J. Rech, R. Henry, L. Ye, H. Ade, W. You and B. T. O'Connor, Chem. Mater., 2019, 31, 5124–5132 CrossRef CAS
.
- J. Choi, W. Kim, S. Kim, T.-S. Kim and B. J. Kim, Chem. Mater., 2019, 31, 9057–9069 CrossRef CAS
.
- Q. Fan, W. Su, S. Chen, W. Kim, X. Chen, B. Lee, T. Liu, U. A. Méndez-Romero, R. Ma, T. Yang, W. Zhuang, Y. Li, Y. Li, T.-S. Kim, L. Hou, C. Yang, H. Yan, D. Yu and E. Wang, Joule, 2020, 4, 658–672 CrossRef CAS
.
- F. P. V. Koch, J. Rivnay, S. Foster, C. Müller, J. M. Downing, E. Buchaca-Domingo, P. Westacott, L. Yu, M. Yuan, M. Baklar, Z. Fei, C. Luscombe, M. A. McLachlan, M. Heeney, G. Rumbles, C. Silva, A. Salleo, J. Nelson, P. Smith and N. Stingelin, Prog. Polym. Sci., 2013, 38, 1978–1989 CrossRef CAS
.
- Y. Wang, J. Lee, X. Hou, C. Labanti, J. Yan, E. Mazzolini, A. Parhar, J. Nelson, J.-S. Kim and Z. Li, Adv. Energy Mater., 2021, 11, 2003002 CrossRef CAS
.
- S. Li, C.-Z. Li, M. Shi and H. Chen, ACS Energy Lett., 2020, 5, 1554–1567 CrossRef CAS
.
- C. Yan, S. Barlow, Z. Wang, H. Yan, A. K. Y. Jen, S. R. Marder and X. Zhan, Nat. Rev. Mater., 2018, 3, 18003 CrossRef CAS
.
- Y. Cui, H. Yao, J. Zhang, K. Xian, T. Zhang, L. Hong, Y. Wang, Y. Xu, K. Ma, C. An, C. He, Z. Wei, F. Gao and J. Hou, Adv. Mater., 2020, 32, 1908205 CrossRef CAS PubMed
.
- J. Yuan, Y. Zhang, L. Zhou, G. Zhang, H.-L. Yip, T.-K. Lau, X. Lu, C. Zhu, H. Peng, P. A. Johnson, M. Leclerc, Y. Cao, J. Ulanski, Y. Li and Y. Zou, Joule, 2019, 3, 1140–1151 CrossRef CAS
.
- Q. Liu, Y. Jiang, K. Jin, J. Qin, J. Xu, W. Li, J. Xiong, J. Liu, Z. Xiao, K. Sun, S. Yang, X. Zhang and L. Ding, Sci. Bull., 2020, 65, 272–275 CrossRef CAS
.
- Y. Lin, M. I. Nugraha, Y. Firdaus, A. D. Scaccabarozzi, F. Aniés, A.-H. Emwas, E. Yengel, X. Zheng, J. Liu, W. Wahyudi, E. Yarali, H. Faber, O. M. Bakr, L. Tsetseris, M. Heeney and T. D. Anthopoulos, ACS Energy Lett., 2020, 5, 3663–3671 CrossRef CAS
.
- P. Bi, S. Zhang, Z. Chen, Y. Xu, Y. Cui, T. Zhang, J. Ren, J. Qin, L. Hong, X. Hao and J. Hou, Joule, 2021, 5, 2408–2419 CrossRef CAS
.
- Z.-G. Zhang and Y. Li, Angew. Chem., Int. Ed., 2021, 60, 4422–4433 CrossRef CAS PubMed
.
- H. Wang, H. Chen, W. Xie, H. Lai, T. Zhao, Y. Zhu, L. Chen, C. Ke, N. Zheng and F. He, Adv. Funct. Mater., 2021, 31, 2100877 CrossRef CAS
.
- T. Wang, R. Sun, W. Wang, H. Li, Y. Wu and J. Min, Chem. Mater., 2021, 33, 761–773 CrossRef CAS
.
- H. Yu, S. Luo, R. Sun, I. Angunawela, Z. Qi, Z. Peng, W. Zhou, H. Han, R. Wei, M. Pan, A. M. H. Cheung, D. Zhao, J. Zhang, H. Ade, J. Min and H. Yan, Adv. Funct. Mater., 2021, 31, 2100791 CrossRef CAS
.
- H. Yang, H. Fan, Z. Wang, H. Yan, Y. Dong, C. Cui, H. Ade and Y. Li, Macromolecules, 2020, 53, 9026–9033 CrossRef CAS
.
- Z.-G. Zhang, Y. Yang, J. Yao, L. Xue, S. Chen, X. Li, W. Morrison, C. Yang and Y. Li, Angew. Chem., Int. Ed., 2017, 56, 13503–13507 CrossRef CAS PubMed
.
- F. Peng, K. An, W. Zhong, Z. Li, L. Ying, N. Li, Z. Huang, C. Zhu, B. Fan, F. Huang and Y. Cao, ACS Energy Lett., 2020, 5, 3702–3707 CrossRef CAS
.
- B. Liu, H. Sun, J.-W. Lee, J. Yang, J. Wang, Y. Li, B. Li, M. Xu, Q. Liao, W. Zhang, D. Han, L. Niu, H. Meng, B. J. Kim and X. Guo, Energy Environ. Sci., 2021, 14, 4499–4507 RSC
.
- Q. Fan, Q. An, Y. Lin, Y. Xia, Q. Li, M. Zhang, W. Su, W. Peng, C. Zhang, F. Liu, L. Hou, W. Zhu, D. Yu, M. Xiao, E. Moons, F. Zhang, T. D. Anthopoulos, O. Inganäs and E. Wang, Energy Environ. Sci., 2020, 13, 5017–5027 RSC
.
- A. Tang, J. Li, B. Zhang, J. Peng and E. Zhou, ACS Macro Lett., 2020, 9, 706–712 CrossRef CAS
.
- Q. Fan, R. Ma, T. Liu, W. Su, W. Peng, M. Zhang, Z. Wang, X. Wen, Z. Cong, Z. Luo, L. Hou, F. Liu, W. Zhu, D. Yu, H. Yan and E. Wang, Sol. RRL, 2020, 4, 2000142 CrossRef CAS
.
- J. Du, K. Hu, L. Meng, I. Angunawela, J. Zhang, S. Qin, A. Liebman-Pelaez, C. Zhu, Z. Zhang, H. Ade and Y. Li, Angew. Chem., Int. Ed., 2020, 59, 15181–15185 CrossRef CAS PubMed
.
- T. Jia, J. Zhang, W. Zhong, Y. Liang, K. Zhang, S. Dong, L. Ying, F. Liu, X. Wang, F. Huang and Y. Cao, Nano Energy, 2020, 72, 104718 CrossRef CAS
.
- S. Huang, F. Wu, Z. Liu, Y. Cui, L. Chen and Y. Chen, J. Energy Chem., 2021, 53, 63–68 CrossRef
.
- H. Yao, L.-K. Ma, H. Yu, J. Yu, P. C. Y. Chow, W. Xue, X. Zou, Y. Chen, J. Liang, L. Arunagiri, F. Gao, H. Sun, G. Zhang, W. Ma and H. Yan, Adv. Energy Mater., 2020, 10, 2001408 CrossRef CAS
.
- Y. Li, Z. Jia, Q. Zhang, Z. Wu, H. Qin, J. Yang, S. Wen, H. Y. Woo, W. Ma, R. Yang and J. Yuan, ACS Appl. Mater. Interfaces, 2020, 12, 33028–33038 CrossRef CAS PubMed
.
- N. Chandrasekaran, E. Gann, N. Jain, A. Kumar, S. Gopinathan, A. Sadhanala, R. H. Friend, A. Kumar, C. R. McNeill and D. Kabra, ACS Appl. Mater. Interfaces, 2016, 8, 20243–20250 CrossRef CAS PubMed
.
- C. N. Hoth, S. A. Choulis, P. Schilinsky and C. J. Brabec, J. Mater. Chem., 2009, 19, 5398–5404 RSC
.
- M. Campoy-Quiles, Y. Kanai, A. El-Basaty, H. Sakai and H. Murata, Org. Electron., 2009, 10, 1120–1132 CrossRef CAS
.
- M.-S. Kim, B.-G. Kim and J. Kim, ACS Appl. Mater. Interfaces, 2009, 1, 1264–1269 CrossRef CAS PubMed
.
- P. Cheng, G. Li, X. Zhan and Y. Yang, Nat. Photonics, 2018, 12, 131–142 CrossRef CAS
.
- R. Sun, W. Wang, H. Yu, Z. Chen, X. Xia, H. Shen, J. Guo, M. Shi, Y. Zheng, Y. Wu, W. Yang, T. Wang, Q. Wu, Y. Yang, X. Lu, J. Xia, C. J. Brabec, H. Yan, Y. Li and J. Min, Joule, 2021, 5, 1548–1565 CrossRef CAS
.
- Y. Kim, S. Cook, S. M. Tuladhar, S. A. Choulis, J. Nelson, J. R. Durrant, D. D. C. Bradley, M. Giles, I. McCulloch, C.-S. Ha and M. Ree, Nat. Mater., 2006, 5(3), 197–203 CrossRef CAS
.
Footnote |
† Y. Kim and H. Park contributed equally to this work. |
|
This journal is © The Royal Society of Chemistry 2022 |