DOI:
10.1039/D2SC04605A
(Edge Article)
Chem. Sci., 2022,
13, 11878-11882
Heteroarylation of unactivated C–H bonds suitable for late-stage functionalization†
Received
17th August 2022
, Accepted 25th September 2022
First published on 26th September 2022
Abstract
The late-stage introduction of diverse heterocycles onto complex small molecules enables efficient access to new medicinally relevant compounds. An attractive approach to such a transformation would utilize the ubiquitous aliphatic C–H bonds of a complex substrate. Herein, we report a system that enables direct C–H heteroarylation using a stable, commercially available O-alkenylhydroxamate with heterocyclic sulfone partners. The C–H heteroarylation proceeds efficiently with a range of aliphatic substrates and common heterocycles, and is a rare example of heteroarylation of strong C–H bonds. Importantly, the present approach is amenable to late-stage functionalization as the substrate is the limiting reagent in all cases.
Introduction
The site-selective, intermolecular functionalization of aliphatic C–H bonds affords unique approaches to the modification of small molecules.1 There are several general platforms which unlock a range of valuable unactivated C–H transformations, including high-valent metal-oxo,2 rhodium carbenoid,3 or polyoxometalate catalysis.4 Alternatively, we have demonstrated the utility of N-functionalized amides to facilitate a range of site-selective C–H functionalizations via hydrogen atom transfer (HAT) involving amidyl radical intermediates.5 Collectively, these approaches encompass an impressive array of C–H transformations.
Despite these achievements, there are important C–H transformations which remain inaccessible. A clear example is the direct, site-selective C(sp3)–H heteroarylation of unactivated, aliphatic C–H bonds. Such a process would enable the concise introduction of synthetically and medicinally relevant heterocycles onto small molecule substrates, while obviating the preparation of functionalized aliphatic coupling partners used in metal-catalyzed C(sp3)–C(sp2) cross-couplings, which are typically used to access such products.6 While there are many useful heteroarylations of weaker C–H bonds,7 reactions involving strong aliphatic C–H bonds have been a challenge (Fig. 1). MacMillan and co-workers reported the first examples of such a transformation via nickel-polyoxometalate dual catalysis and aryl halide coupling partners.8 While it is impressively broad in scope, excess C–H substrate was required in all cases, which is suboptimal in late-stage contexts where the starting material is precious. Several other approaches to aliphatic C–H heteroarylation have been reported, including cross-dehydrogenative couplings,9 Minisci-type additions,10 and homolytic aromatic substitutions.11 These transformations also require the use of substrate in large excess and feature poorly selective HAT reagents which are undesirable in applications involving complex substrates with multiple reactive sites.12 Additionally, C–H heteroarylations involving Minisci-type additions can suffer from poor regio- and chemoselectivity in the radical addition, leading to mixtures of products and, in certain cases, overalkylation.13
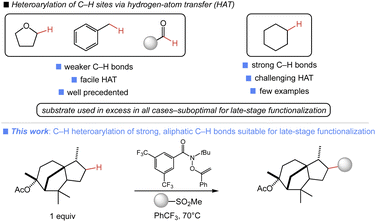 |
| Fig. 1 Heteroarylation of C–H bonds for late-stage functionalization. | |
We have recently demonstrated the ability of a simple, commercially available O-alkenylhydroxamate reagent to enable the broad diversification of C–H bonds using substrate as limiting reagent.5a In this prior work, group transfer reagents capable of generating a sulfonyl radical to facilitate chain transfer were well suited to C–H diversification. We hypothesized that the use of readily accessed heteroaromatic sulfones in this manifold could unlock a direct heteroarylation of aliphatic C–H bonds. Herein, we report the successful development of such a process, enabling the direct C(sp3)–H heteroarylation of diverse aliphatic substrates. This approach uses the substrate as limiting reagent in all cases and is thus ideally suited for the late-stage functionalization (LSF) of complex molecules.
Results and discussion
We began our studies with the C–H benzothiazolylation of a range of simple substrates using O–alkenylhydroxamate 1 and 2-(methylsulfonyl)benzothiazole as coupling partner in PhCF3 at 70 °C (Fig. 2). The reactions of common cyclic and bridged hydrocarbons provided the C–H heteroarylation products 2–6 in good yield (34–75%). Notably, the functionalization of norbornane 4 proceeded with excellent exo stereoselectivity (>20
:
1 dr), and that of adamantane provided 5 as a single regioisomer resulting from functionalization at the less hindered tertiary C–H site. The reaction of 2-adamantanone to afford 6 occurred selectively at the more electron-rich tertiary C–H site distal from the ketone functionality; previous heteroarylation of this substrate using decatungstate as catalyst proceeded with only modest regioselectivity.8 Common saturated heterocycles also reacted efficiently, delivering products 7–9 with excellent regioselectivity and good yield (47–91%). Notably, the functionalization of N-Boc morpholine proceeded with excellent regioselectivity to deliver 9 as a single regioisomer, in contrast to the mixture of regioisomers produced by decatungstate-catalyzed functionalization of this substrate.8 Heteroarylation of [2.2.1] oxabicycloheptane produced product 10 in moderate yield (39%), but with the high levels of diastereoselectivity (>20
:
1 dr) typical of this system. Linear substrates also participate in the reaction, as demonstrated by the functionalization of the pinacol acetal of 2-pentanone, delivering 11 albeit with modest regioselectivity.
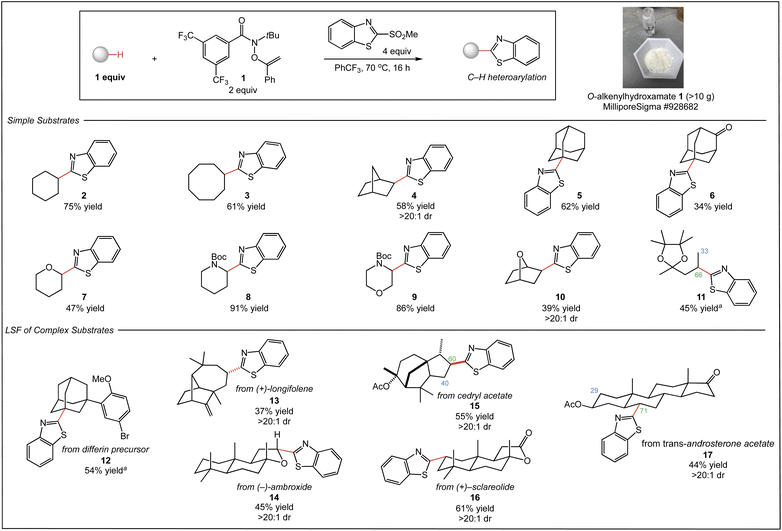 |
| Fig. 2 C–H heteroarylation of diverse substrates using 2-(methylsulfonyl)benzothiazole as coupling partner. All yields are of isolated product unless otherwise indicated. Regio- and diastereoselectivities were determined by 1H NMR spectroscopy of crude reaction mixture. Percent functionalization values are provided in examples containing regioisomeric mixtures. aReaction yield determined by NMR using an internal standard. | |
A distinct advantage of our approach is the success of the heteroarylation using substrate as limiting reagent, which is crucial to applications in LSF. As shown in Fig. 2, we next explored the site-selective C–H heteroarylation of several complex molecules. Heteroarylation of a differin precursor afforded product 12 in 54% yield. As with adamantane and 2-adamantanone, the reaction was highly selective for the less sterically encumbered tertiary C–H site. LSF of the terpenoid natural product (+)–longifolene proceeded exclusively at the least hindered methylene site to deliver 13 with high diastereoselectivity, demonstrating successful C–H heteroarylation in the presence of alkenes. The benzothiazolylation of (−)–ambroxide produced 14 in 45% isolated yield as a single diastereomer, highlighting the excellent stereoselectivity of the reaction system; alternative LSFs of this substrate by us5a,5d and others14 delivered a mixture of diastereomers. Stereoselective C–H heteroarylation of the sesquiterpene cedryl acetate delivered single diastereomers of 15 in good combined yield (55%), favoring functionalization of the most accessible methylene site (1.5
:
1 rr). Terpenoid (+)–sclareolide—a benchmark substrate for intermolecular C–H functionalization—underwent reaction with substrate as limiting reagent to afford 16 in 61% isolated yield as a single diastereomer. Prior C–H heteroarylation of this substrate using nickel/polyoxometalate dual-catalysis required (+)–sclareolide in superstoichiometric amounts (5 equiv.).8 Steroid trans-androsterone acetate was also derivatized with high stereoselectivity, favoring the C6 site on the B-ring (44% yield, 2.4
:
1 rr).
We next surveyed diverse heteroaryl coupling partners in this transformation (Fig. 3).15 Benzoxazole and benzimidazole sulfones coupled with similar efficiency to benzothiazole, delivering C–H heteroarylation products of (+)–sclareolide (18 and 19) in good yield. Interestingly, this represents a formal approach to aliphatic C–H carboxylation as benzoxazoles can be hydrolyzed to carboxylic acids.16 1,2-Azoles were also easily transferred, as demonstrated by the successful couplings of thiazole and oxazole to deliver products 20 and 23, respectively. In addition, oxadiazole was an excellent coupling partner (21, 71% isolated yield).
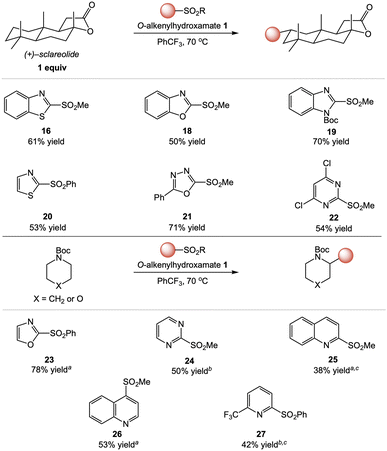 |
| Fig. 3 C–H heteroarylations featuring a variety of heterocyclic sulfones. All reactions were performed with 1 equiv. of substrate, 2 equiv. of 1, and 4 equiv. of the corresponding heteroaryl sulfone in PhCF3. All yields are of isolated product unless otherwise indicated. aReaction using N-Boc piperidine as substrate. bReaction using N-Boc morpholine as substrate. cReaction yield determined by 1H NMR using an internal standard. | |
Our studies continued with pyrimidine and quinoline heterocycles known to present regioselectivity challenges in Minisci-type radical addition processes.17,18 In particular, C–H heteroarylations involving pyrimidinyl sulfones proceeded with complete regioselectivity for the 2-position to deliver 22 and 24, in contrast with Minisci-type heteroarylations of pyrimidines which produce regioisomeric mixtures.17 Furthermore, reactions of both quinolines also delivered C–H heteroarylation products (25 and 26) with high regioselectivity for the sulfone substituted position of the heteroarene. As with pyrimidines, Minisci-type heteroarylations involving quinolines typically produce regioisomeric mixtures or dialkylation products unless sites are blocked by substitution.13,18 Attempts to extend the heteroarene scope to pyridines were initially unsuccessful, as reactions using either 2-(methylsulfonyl)- or 2-(phenylsulfonyl)pyridine proceeded in low yields (<15%). We hypothesized that the use of a more electron-deficient pyridine could facilitate alkyl radical addition and heterocycle transfer, and heteroarylation with 2-(phenylsulfonyl)-6-(trifluoromethyl)pyridine was indeed more efficient (27, 42% yield).
We propose that the C–H heteroarylation proceeds via hydrogen atom abstraction by an amidyl radical followed by heteroaryl group transfer (Scheme 1). Upon heating, N–O bond homolysis delivers the amidyl radical which can abstract a strong aliphatic C–H bond in a site-selective manner. The resulting carbon-centered radical participates in a homolytic aromatic substitution with the heteroaryl sulfone partner to deliver product and the chain-carrying sulfonyl radical. The sulfonyl radical adds to the O–alkenylhydroxamate reagent 1 to furnish an amidyl radical and propagate the chain process.19
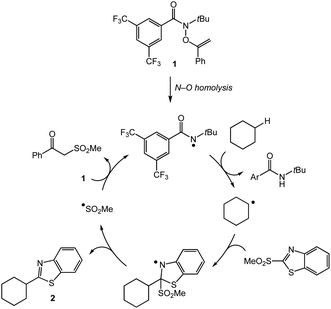 |
| Scheme 1 Proposed radical chain mechanism for the C–H heteroarylation. | |
Conclusions
In conclusion, we have developed a practical approach to the direct C–H heteroarylation of unactivated aliphatic C–H bonds using easily accessed heteroaryl sulfones and a commercially available O-alkenylhydroxamate reagent. A diverse array of synthetically and medicinally relevant heteroarenes are efficient coupling partners, providing a broad platform for molecular diversification. Importantly, all examples herein use the substrate as limiting reagent, essential to applications in the late-stage functionalization of complex molecules. We anticipate that this C–H heteroarylation will prove useful in chemical synthesis across a range of contexts.
Data availability
Experimental procedures, reaction optimization, compound characterization, NMR spectra, and additional data are located within the ESI.†
Author contributions
E. J. A. and A. S. M. conceived of the work and designed the experiments. A. S. M. performed and analyzed the experiments. All authors prepared and reviewed the manuscript. E. J. A. acquired the funding to support the work.
Conflicts of interest
There are no conflicts to declare.
Acknowledgements
This work was supported by Award No. R35 GM131708 from the National Institute of General Medical Sciences. We thank the University of North Carolina's Department of Chemistry NMR Core Laboratory for the use of their NMR spectrometers, and this material is based upon work supported by the National Science Foundation under Grant No. CHE-0922858. In addition, we thank the University of North Carolina's Department of Chemistry Mass Spectrometry Core Laboratory, especially Dr Brandie Ehrmann and Diane Witherspoon, for their assistance with mass spectrometry analysis supported by the National Science Foundation under Grant No. CHE-1726291.
Notes and references
-
(a) J. F. Hartwig, J. Am. Chem. Soc., 2016, 138, 2–24 CrossRef CAS;
(b) D. J. Abrams, P. A. Provencher and E. J. Sorensen, Chem. Soc. Rev., 2018, 47, 8925–8967 RSC;
(c) W. R. Gutekunst and P. S. Baran, Chem. Soc. Rev., 2011, 40, 1976–1991 RSC;
(d) H. M. L. Davies, J. Org. Chem., 2019, 84, 12722–12745 CrossRef CAS PubMed.
- For representative examples of C–H functionalization involving metal-oxo complexes, see:
(a) M. C. White, Science, 2012, 335, 807–809 CrossRef CAS PubMed;
(b) W. Liu and J. T. Groves, Acc. Chem. Res., 2015, 48, 1727–1735 CrossRef CAS PubMed;
(c) M. Costas, Coord. Chem. Rev., 2011, 255, 2912–2932 CrossRef CAS;
(d) A. Sharma and J. F. Hartwig, Nature, 2015, 517, 600–604 CrossRef CAS PubMed;
(e) T. J. Osberger, D. C. Rogness, J. T. Kohrt, A. F. Stepan and M. C. White, Nature, 2016, 537, 214–219 CrossRef CAS PubMed;
(f) J. R. Frost, S. M. Huber, S. Breitenlechner, C. Bannwarth and T. Bach, Angew. Chem., Int. Ed., 2015, 54, 691–695 CAS;
(g) M. Milan, M. Bietti and M. Costas, ACS Cent. Sci., 2017, 3, 196–204 CrossRef CAS PubMed.
- For representative examples of C–H functionalization involving rhodium carbenoids, see:
(a) R. J. Manning and H. M. L. Davies, Nature, 2008, 451, 417–424 CrossRef PubMed;
(b) M. Peña−López and M. Beller, Angew. Chem., Int. Ed., 2017, 56, 46–48 CrossRef PubMed;
(c) K. Liao, S. Negretti, D. G. Musaev, J. Bacsa and H. M. L. Davies, Nature, 2016, 533, 230–234 CrossRef CAS PubMed.
- For representative examples of C–H functionalization involving polyoxometalate complexes, see:
(a) L. Capaldo and D. Ravelli, Eur. J. Org. Chem., 2017, 15, 2056–2071 CrossRef;
(b) P. J. Sarver, V. Bacauana, D. M. Schultz, D. A. DiRocco, Y. H. Lam, E. C. Sherer and D. W. C. MacMillan, Nature, 2020, 12, 459–467 CAS;
(c) S. D. Halperin, H. Fan, S. Chang, R. E. Martin and R. Britton, Angew. Chem., Int. Ed., 2014, 53, 4690–4693 CrossRef CAS PubMed;
(d) P. J. Sarver, N. B. Bissonnette and D. W. C. MacMillan, J. Am. Chem. Soc., 2021, 143, 9737–9743 CrossRef CAS PubMed;
(e) D. Ravelli, M. Fagnoni, T. Fukuyama, T. Nishikawa and I. Ryu, ACS Catal., 2018, 8, 701–713 CrossRef CAS.
-
(a) T. J. Fazekas, J. W. Alty, E. K. Neidhart, A. S. Miller, F. A. Leibfarth and E. J. Alexanian, Science, 2022, 375, 545–550 CrossRef CAS PubMed;
(b) V. A. Schmidt, R. K. Quinn, A. T. Brusoe and E. J. Alexanian, J. Am. Chem. Soc., 2014, 136, 14389–14392 CrossRef CAS PubMed;
(c) R. K. Quinn, Z. Konst, S. Michalak, Y. Schmidt, A. Szklarksi, Z. Flores, S. Nam, D. Horne, C. Vanderwal and E. J. Alexanian, J. Am. Chem. Soc., 2016, 138, 696–702 CrossRef CAS PubMed;
(d) W. L. Czaplyski, C. G. Na and E. J. Alexanian, J. Am. Chem. Soc., 2016, 138, 13854–13857 CrossRef CAS PubMed;
(e) J. B. Williamson, J. W. L. Czaplyski, E. J. Alexanian and F. A. Leibfarth, Angew. Chem., Int. Ed., 2018, 57, 6261–6265 CrossRef CAS;
(f) A. M. Carestia and E. J. Alexanian, Chem. Sci., 2018, 9, 5360–5365 RSC.
-
(a) R. Jana, T. P. Pathak and M. S. Sigman, Chem. Rev., 2011, 111, 1417–1492 CrossRef CAS PubMed;
(b) S. Z. Tasker, E. A. Standley and T. F. Jamison, Nature, 2014, 509, 299–309 CrossRef CAS;
(c) Z. Zuo and D. W. C. MacMillan, J. Am. Chem. Soc., 2014, 136, 5257–5260 CrossRef CAS PubMed;
(d) J. C. Lo, J. Gui, Y. Yabe, C. M. Pan and P. S. Baran, Nature, 2014, 516, 343–348 CrossRef CAS PubMed.
-
(a) S. Liu, A. Liu, Y. Zhang and W. Wang, Chem. Sci., 2017, 8, 4044–4050 RSC;
(b) T. He, L. Yu, L. Zhang, L. Wang and M. Wang, Org. Lett., 2011, 13, 5016–5019 CrossRef CAS;
(c) A. Lipp, G. Lahm and T. Opatz, J. Org. Chem., 2016, 81, 4890–4897 CrossRef CAS PubMed;
(d) W. Zhang, L. Wu, P. Chen and G. Liu, Angew. Chem., Int. Ed., 2019, 58, 6425–6429 CrossRef CAS;
(e) K. Qvortrup, D. A. Rankic and D. W. C. MacMillan, J. Am. Chem. Soc., 2014, 136, 626–629 CrossRef CAS PubMed;
(f) J. Jin and D. W. C. MacMillan, Angew. Chem., Int. Ed., 2015, 54, 1565–1569 CrossRef CAS.
- I. B. Perry, T. F. Brewer, P. J. Sarver, D. M. Schultz, D. A. DiRocco and D. W. C. MacMillan, Nature, 2018, 560, 70–75 CrossRef CAS.
-
(a) H. Tian, H. Yang, C. Tian, G. An and G. Li, Org. Lett., 2020, 22, 7709–7715 CrossRef CAS PubMed;
(b) R. Xia, H.-Y. Niu, G.-R. Qu and H.-M. Guo, Org. Lett., 2012, 14, 5546–5549 CrossRef CAS PubMed.
-
(a) X. Shao, X. Wu, S. Wu and C. Zhu, Org. Lett., 2020, 22, 7450–7454 CrossRef CAS PubMed;
(b) L. Zhang, B. Pfund, O. S. Wenger and X. Hu, Angew. Chem., Int. Ed., 2022, 61, e202202649 CAS;
(c) L. Zhou and H. Togo, Eur. J. Org. Chem., 2019, 2019, 1627–1634 CrossRef CAS.
-
(a) Y. Ikeda, Y. Matsukawa, K. Yonekura and E. Shirakawa, Eur. J. Org. Chem., 2021, 2021, 794–797 CrossRef CAS;
(b) S. Kamijo, K. Kamijo and T. Murafuji, J. Org. Chem., 2017, 82, 2664–2671 CrossRef CAS PubMed;
(c) X.-Z. Fan, J.-W. Rong, H.-L. Wu, Q. Zhou, H.-P. Deng, J. D. Tan, C.-W. Xue, L.-Z. Wu, H.-R. Tao and J. Wu, Angew. Chem., Int. Ed., 2018, 57, 8514–8518 CrossRef CAS PubMed.
- For a recent study achieving several C–H functionalizations using substrate as limiting reagent, including C–H heteroarylations introducing pyridines and quinolines, see: H. Cao, D. Kong, L.-C. Yang, S. Chanmungkalakul, T. Liu, J. L. Piper, Z. Peng, L. Gao, Z. Lie, X. Hong and J. Wu, Nat. Synth., 2022 DOI:10.1038/s44160-022-00125-1.
- For reviews, see:
(a) R. S. J. Proctor and R. J. Phipps, Angew. Chem., Int. Ed., 2019, 58, 13666–13699 CrossRef CAS PubMed . For representative examples, see:;
(b) A. P. Antonchick and L. Burgmann, Angew. Chem., Int. Ed., 2013, 52, 3267–3271 CrossRef CAS PubMed;
(c) X. Wu, J. W. T. See, K. Xu, H. Hirao, J. Roger, J.-C. Hierso and J. (Steve) Zhou, Angew. Chem., Int. Ed., 2014, 53, 13573–13577 CrossRef CAS PubMed;
(d) G.-X. Li, X. Hu, G. He and G. Chen, ACS Catal., 2018, 8, 11847–11853 CrossRef CAS.
-
(a) K. Feng, R. E. Quevedo, J. T. Kohrt, M. S. Oderinde, U. Reilly and M. C. White, Nature, 2020, 580, 621–627 CrossRef CAS;
(b) B. Chandra, K. K. Singh and S. S. Gupta, Chem. Sci., 2017, 8, 7545–7551 RSC.
- The substrates chosen for C–H heteroarylation in Fig. 3 were selected to facilitate the isolation of products, which was challenging in certain instances.
-
(a) J. Kumar, A. Gupta and S. Bhadra, Org. Biomol. Chem., 2019, 17, 3314–3318 RSC;
(b) Z. D. Miller, B. J. Lee and T. P. Yoon, Angew. Chem., Int. Ed., 2017, 56, 11891–11895 CrossRef CAS.
- G.-X. Li, C. A. Morales-Rivera, Y. Wang, F. Gao, G. He, P. Liu and G. Chen, Chem. Sci., 2016, 7, 6407–6412 RSC.
- J. Dong, X. Lyu, Z. Wang, X. Wang, H. Song, Y. Liu and Q. Wang, Chem. Sci., 2019, 10, 976–982 RSC.
- The sulfonyl radical addition byproduct from the reaction with (+)-sclareolide to form 16 was isolated and characterized. See the ESI† for details.
|
This journal is © The Royal Society of Chemistry 2022 |