DOI:
10.1039/D2SC03093D
(Edge Article)
Chem. Sci., 2022,
13, 9016-9022
Adaptive coordination assemblies based on a flexible tetraazacyclododecane ligand for promoting carbon dioxide fixation†
Received
2nd June 2022
, Accepted 4th July 2022
First published on 5th July 2022
Abstract
Coordination hosts based on flexible ligands have received increasing attention due to their inherent adaptive cavities that often show induced-fit guest binding and catalysis like enzymes. Herein, we report the controlled self-assembly of a series of homo/heterometallic coordination hosts (Me4enPd)2n(ML)n [n = 2/3; M = Zn(II)/Co(II)/Ni(II)/Cu(II)/Pd(II)/Ag(I); Me4en: N,N,N′,N′-tetramethylethylenediamine] with different shapes (tube/cage) from a flexible tetraazacyclododecane-based pyridinyl ligand (L) and cis-blocking Me4enPd(II) units. While the Ag(I)-metalated ligand (AgL) gave rise to the formation of a (Me4enPd)4(ML)2-type cage, all other M(II) ions led to isostructural (Me4enPd)6(ML)3-type tubular complexes. Structural transformations between cages and tubes could be realized through transmetalation of the ligand. The buffering effect on the ML panels endows the coordination tubes with remarkable acid–base resistance, which makes the (Me4enPd)6(ZnL)3 host an effective catalyst for the CO2 to CO32− conversion. Control experiments suggested that the integration of multiple active Zn(II) sites on the tubular host and the perfect geometry match between CO32− and the cavity synergistically promoted such a conversion. Our results provide an important strategy for the design of adaptive coordination hosts to achieve efficient carbon fixation.
Introduction
Carbon dioxide (CO2) in the atmosphere has been rising due to humans' reliance on fossil fuels, causing a range of climate problems such as rising temperature and changing sea-levels. To eliminate such problems, it is necessary to develop efficient and economic carbon fixation technologies.1,2 In order to transform CO2 into valuable compounds, various carbon fixation technologies such as metal-participated chemical conversions,3 electrocatalysis,4 and photocatalysis5,6 have been developed. Ideally, the carbon fixation process itself should avoid CO2 emissions to achieve carbon neutralization. However, it remains a big challenge to develop such carbon fixation processes.
In nature, carbonic anhydrase can convert CO2 into HCO3− under mild conditions. Structural analysis shows that carbonic anhydrase contains a flexible cavity that can hold CO2 and a coordination unsaturated Zn(II) ion acting as the active site.7,8 Inspired by this, many unusual metal complexes which can be used to fix CO2 through binding with carbonate have been reported.9–13 Coordination-directed self-assembly has been proven to be an effective approach toward the predictable construction of multicomponent artificial hosts,14–77 where enhanced guest reactivity,73,74,78–87 stabilization of metastable species,88–90 and unusual reaction pathways78,91–94 have been realized. While atmospheric CO2 sequestration by selective binding of carbonate with coordination hosts has already been reported,95–102 adaptive coordination hosts that contain multiple active metal sites and can reversibly encapsulate and release the carbonate are still rare.
1,4,7,10-Tetraazacyclododecane and its derivatives are well-studied macrocyclic ligands to form stable complexes with various metal ions.103–106 However, its utilization in coordination-directed self-assembly has been hampered due to its structural flexibility.21,23 Herein, we report the synthesis of a series of coordination tubes or cages from a 1,4,7,10-tetraazacyclododecane-N,N′,N′′,N′′′-tetra-p-methylpyridine ligand (L) and cis-blocking Me4enPd units (Scheme 1). The structure of the final assemblies is found to be sensitive to different metallations on L. Moreover, integration of multiple active Zn(II) sites on the (Me4enPd)6(ZnL)3(NO3)18 (1-Zn) tubular host and perfect CO32− encapsulation in the cavity promoted its potential utilization in CO2 fixation.
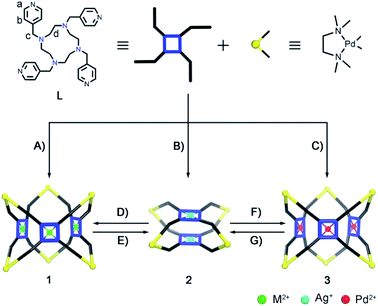 |
| Scheme 1 Controlled self-assembly and transformations between coordination cages and tubes. (A) Coordination tubes [(PdMe4en)6(ML)3]18+ (1-M) (M = Zn, Co, Ni, Cu) by metallation of L with M(II). (B) Coordination cages [(PdMe4en)4(AgL)2]12+ (2) by metallation of L with Ag(I). (C) Coordination tubes [(PdMe4en)6(PdL)3]18+ (3) by metallation of L with Pd(II). (D) Zn(II) induced transformation from 2 to 1-Zn. (E) Ag(I) driven transformation from tube 1-Zn to 2. (F) Pd(II) induced transformation from cage 2 to 3. (G) Ag(I) driven transformation from 3 to 2. | |
Results and discussion
Controlled self-assembly and structural transformations between coordination tubes 1 and 3, and cages 2
The tetraazacyclododecane moiety located at the center of ligand L is prone to being metalated due to the macrocyclic effect. Indeed, treatment of L with Zn(NO3)2 led to the quantitative formation of the ZnL metalloligand, as confirmed by 1HNMR (Fig. 1A and B, S1–S8†) and single crystal X-ray diffraction (SCXRD) studies. In the 1HNMR spectrum of ZnL, the signal of Hd is not only down-field shifted but also splits into two peaks, suggesting that metallation of L by Zn(II) rigidified its conformation in solution. In the crystal structure, four pyridine groups on ZnL are in the same plane rather than the randomly distributed form seen on the free L (Fig. 2A and B, S9 and S10†). Reacting Me4enPd(NO3)2 with ZnL in D2O at 70 °C for 3 h led to formation of one clean complex (1-Zn). 1HNMR analyses show that both the signals of Ha and Hb on 1-Zn shift to the down-field than that on ZnL, due to the coordination of pyridine groups to Pd(II) (Fig. 1C, S11–S13†). Diffusion ordered NMR spectroscopy (DOSY) further indicates the purity and the size (about 2.1 nm) of 1-Zn (Fig. S14†). The X-ray crystal structure of 1-Zn was also obtained, which clearly confirmed its tubular structure [(Me4enPd)6(ZnL)3]18+ (Fig. 2C, S15†). Six Pd(II) capping units are located on the six vertices of the triangular prism, while three ZnL ligands define the three faces of the triangular prism, with one NO3− encapsulated in the tubular cavity. The chelated Zn(II) ion displays a tetragonal pyramid geometry through coordinating with four N atoms from L and one O atom from water. Such coordination mode makes the new-formed metalloligand ZnL adopt the planar conformation, leading to the formation of a tubular structure. The triangular apertures on the tube are about 13.7 Å × 13.7 Å × 13.7 Å. No anion effect was observed on the self-assembly of tube 1-Zn, as isostructural tubular complexes were obtained using other Me4enPd (BF4−, SO42−, ClO4−, SbF6− salts) (Fig. S16–S20†). One-pot self-assembly starting from L, Zn(NO3)2 and Me4enPd salt also leads to the coordination tube 1-Zn (Fig. S21†).
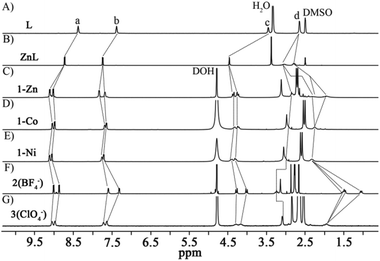 |
| Fig. 1
1HNMR spectra of (A) free ligand L, (B) ZnL, (C) 1-Zn, (D) 1-Co, (E) 1-Ni, (F) 2(BF4−), and (G) 3(ClO4−). | |
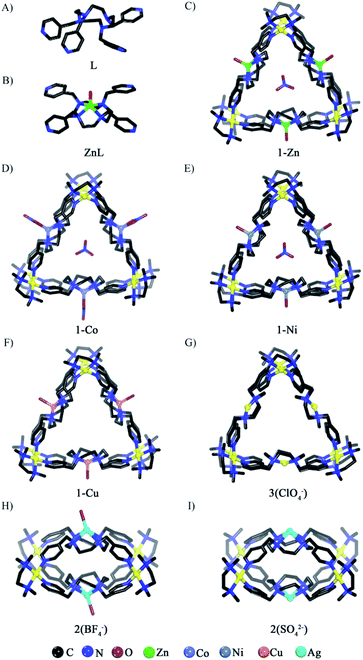 |
| Fig. 2 The crystal structures of (A) free ligand L, (B) ZnL, (C) 1-Zn, (D) 1-Co, (E) 1-Ni, (F) 1-Cu, (G) 3(ClO4−), (H) 2(BF4−), and (I) 2(SO42−). | |
When other transition metal ions M(II) (M = Co, Ni, Cu) that can adopt similar coordination geometries to Zn(II) ions were used, heterometallic coordination tubes [(Me4enPd)6(ML)3]18+ [M = Co2+ (1-Co), Ni2+ (1-Ni), Cu2+ (1-Cu)] were obtained (Fig. 1D and E, S22–S27†). SCXRD analyses confirm that complexes 1-Ni and 1-Cu are isostructural to tube 1-Zn, except that no NO3− can be found in the cavity of 1-Cu (Fig. 2E and F, S29 and S30†). Although the chelated Co(II) ion in 1-Co displays a distorted octahedral configuration, it has the same coordination form with the ligand L as the Zn(II), so the Co(II) ion also induces the formation of a tubular structure (Fig. 2D, S28†). In short, Zn(II)/Co(II)/Ni(II)/Cu(II) ions can be used as templates to induce the self-assembly of heterometallic tubular tubes (1-M) because they can drive the metalloligand MLs to adopt a planar conformation (Scheme 1A).
To our surprise, cage complexes 2 were obtained by the in situ self-assembly of L, PdMe4en(Cl)2 and AgX (X = BF4−, SO42−), as confirmed by 1H and DOSY NMR spectra (Fig. 1F, S31–S34 and S36–S39†). The 1HNMR spectrum of 2(BF4−) is distinct from that of 1 (Fig. 1C and F). DOSY shows that the size of 2 is about 1.28 nm (Fig. S34 and S39†), much smaller than that of 1. SCXRD confirmed that 2 complexes with different counter ions (either BF4− or SO42−) are isostructural coordination cages with the same [(PdMe4en)4(AgL)2]12+ skeleton (Fig. 2H and I). The crystal structures of 2 show that all the ligands L became the metalated AgL. Both structures are composed of two AgL ligands and four Pd(II) nodes with almost no cavity observed (Fig. S35 and S40†). The coordination environments of the Ag(I) centers on the AgL ligands of 2 (BF4− salt) and 2 (SO42− salt) are similar except that one additional axial coordinating H2O was observed on the former (Fig. 2H and I). The longer Ag–N bond length (2.5 Å on average) compared to that of Zn–N (2.1 Å on average) leads to a slight conical conformation of AgL other than the flat conformation observed for ZnL. This conformational change on AgL leads to the formation of the cage structure. Isostructural cages 2 (NO3−, ClO4−) also could be obtained when AgNO3 and AgClO4 were used (Fig. S41†), further confirming that Ag(I) ions can template the self-assembly of coordination cages (2) (Scheme 1B).
It then turns out that the metallation of L was a prerequisite for the successful self-assembly. Trail on direct self-assembly of L with Me4enPd(NO3)2 gave a mixture of products (Fig. S42 and S43†). Interestingly, reacting ligand L with Me4enPd(ClO4)2 led to a new (Me4enPd)6(PdL)3-type tube complex (3), with the in situ metallation of L with Pd(II). The 1HNMR spectrum of 3 is clearly different from those of both 1 and 2 (Fig. 1G and S44–S46†). DOSY shows that the size of 3(ClO4−) is about 2.2 nm (Fig. S47†), very close to that of tube 1-Zn. SCXRD finally confirms that 3(ClO4−) is a Pd-chelated triangular coordination tube [(Me4enPd)6(PdL)3](ClO4)18 (Fig. 2G, S48†). The crystal structure of 3(ClO4−) contains two independent tubular molecules. Both of them have a triangular prism structure similar to that of 1-Zn, where six Pd nodes are located on six vertices of the triangular prism and three PdL metalloligands span three faces of the prism. The Pd(II) ions chelated in the PdL ligands adopt the square-planar coordination geometry. Like ZnL, PdL also adopts a planar conformation, so a similar tube complex is formed. A clean tubular complex 3 (SbF6− salt) could also be obtained when Me4enPd(SbF6)2 was used (Fig. S49–S52†). However, both Me4enPd(BF4)2 and Me4enPdSO4 lead to a mixture of products, demonstrating an obvious anionic effect (Fig. S53†).
With the structure of hetero/homometallic coordination tubes 1 and 3, and cages 2 determined, we then wondered whether controllable structural transformation between cages and tubes can be achieved through transmetalation on the ML. Indeed, the addition of Zn(II) ions to the Ag-chelated cage 2 triggered its complete transformation into Zn-chelated tube 1-Zn (Fig. S54–S56†). Due to the much stronger coordination ability of Zn(II) than Ag(I) with L, it is difficult for Ag(I) ions to drive the back transformation. Even with a large excess of Ag(I) ions, only a partial transformation from tube 1-Zn to cage 2 was observed (Fig. S57–S59†). Similarly, cage 2 can be completely transformed into tube 3 by the addition of Pd(II) salts, where use of Pd(SbF6)2 can greatly promote this transformation (Fig. S60–S62†), while back transformation from tube 3 to cage 2 is successful by the addition of AgBF4 or AgNO3, but not for AgSbF6 (Fig. S63–S65†). We infer that SbF6− may have a stronger interaction with tube 3 than cage 2, so it can promote the conversion of cage 2 to tube 3 but hamper the back transformation. In brief, controllable transformations between cages and tubes can be realized via transmetalation on the ligands with a certain degree of anion effects (Scheme 1D–G).
Fine-tuned shapes and cavities on the tubular complexes
It's worth noting that the utilization of the flexible tetraazacyclododecane-based ligand not only facilitates the incorporation of various metal ions on the coordination tubes, but also provides a good opportunity to fine-tune the volumes of their central cavities. Based on the crystal data, deformations of geometry on L depending on its metallation with different metal ions are clearly observed, leading to gradual volume changes from 316 (1-Ni) to 409 [3(ClO4−)] Å3 (Fig. 3B). For the isostructural heterometallic tubes 1-Zn/Ni/Cu, their central metals share the same coordination environment, and the volumes of the tubes are found to be proportional to the M–N bond lengths on MLs (N-Ni < N-Cu < N-Zn). We infer that shorter coordination bonds make the MLs panels more convex, while such conformational changes do not cause significant changes to the triangular opening (Fig. 3A). 1-Co and 3(ClO4−) with quite different coordination environments on MLs bear much larger volumes than the above three tubes, with the largest volume of 409 Å3 measured for tube 3(ClO4−), which is ca. 1.3 times that for 1-Ni. We infer that the volume increases in these two tubes are due to their less curved ML panels.
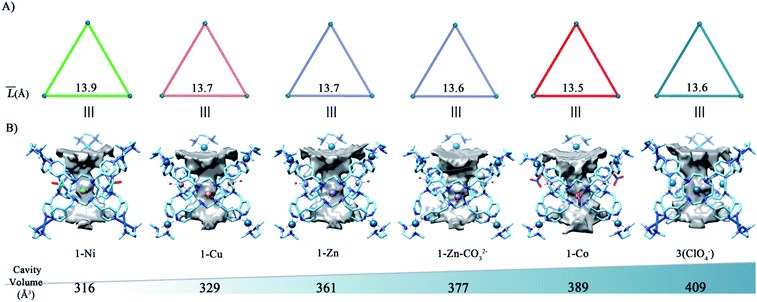 |
| Fig. 3 (A) The average lengths of triangular apertures on different tubular structures. (B) The volumes of cavities on tubes 1-Ni (316 Å3), 1-Cu (329 Å3), 1-Zn (361 Å3), 1-Zn-CO32− (377 Å3), 1-Co (389 Å3), and 3(ClO4−) (409 Å3). | |
Acid–base resistance of coordination tubes
Stability of the coordination assemblies is an important prerequisite for their applications.21,23 Impressively, 1H-NMR for tube 1-Zn in aqueous solutions confirmed that it maintained its structural integrity under both strongly acidic (pH = 1) and basic conditions (pH = 12) but would decompose in stronger acidic (pH = 0) or basic (pH = 13) solutions (Fig. S66†). Tubes 1-Co and 1-Ni also remain stable at pH 12, but their acid resistance is poorer than that of 1-Zn (Fig. S67 and S68†). For comparison, a simple coordination complex (Me4enPd)Py2(NO3)2 (Py = pyridine) was synthesized. 1H-NMR tests indicate that it can only remain intact in a smaller pH range (pH 3–11; Fig. S69†), suggesting that multi-component cooperativity on the tube enhances its structural stability. The buffering effect of ML, i.e., accepting H+ on the N atoms of tetraazacyclododecane and OH− on the metal centers via axial coordination, should also contribute to the observed acid–base resistance of the tube complexes. While many acid or base resistant coordination hosts have been reported, few of them are stable toward both acidic and basic conditions. Coordination hosts built from inert coordination bonds, such as Pt–N, and Zr–O bonds, tend to show enhanced stability.107,108 Compared with the strategy of using stronger coordination bonds, here we improve the acid–base stability of the host by creating a local buffer environment, which provides a useful strategy for the construction of stable coordination hosts.
CO2 fixation promoted by coordination tubes
Considering that the coordination tube 1-Zn contains three Zn active sites and is tolerable toward basic solutions, we then studied its applications in carbonic anhydrase mimicking. The CO2 dissolution experiment indicates that CO2 has better solubility in DMSO/H2O (1
:
2 v/v) mixed solvent than pure water (Fig. S70†). So, a DMSO-d6/D2O mixed solvent containing 1-Zn (5 mM) and NaOH (0.01 M) was used for the CO2 capture reaction. When CO2 gas was continuously injected into this solution at room temperature for 3 h, a signal at 160 ppm assignable to CO32− was clearly observed in 13CNMR (Fig. 4B(c) and (d)), whereas no CO32− was observed without adding 1-Zn, or with 1-Co, 1-Ni as the catalysts (Fig. 4B(a) and (b), S71†). A control experiment with a mononuclear ZnL1(NO3)2 (L1 = 1,4,7,10-tetraazacyclododecane) complex as the catalyst, which contains a similar Zn(II) active site, also showed no CO2 conversion (Fig. S72–S74†). Taking together all these experiments, it is clear that both the existence of the Zn metal sites and the formation of the tubular cavity are indispensable to facilitate the CO2 to CO32− conversion.
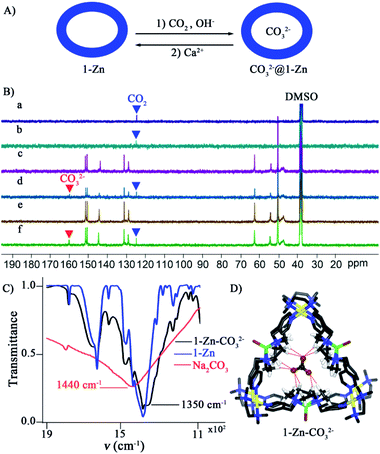 |
| Fig. 4 (A) The 1-Zn tube assisted CO2 to CO32− conversion. (B) The 13C NMR spectra of CO2 (a), the blank experiment (b), 1-Zn (c) and 1-Zn promoted CO2 to CO32− transformation (d), after removing CO32− with Ca2+ (e), and reuse of tube 1-Zn (f). (C) The IR spectra of tube 1-Zn before and after CO2 fixation. (D) The crystal structure of 1-Zn-CO32−. | |
Further studies indicate that adding more NaOH and prolonging the reaction time cannot enhance the yield of CO32−in solution (Fig. S75†). The IR spectra of tube 1-Zn after the reaction show a new absorption peak at 1350 cm−1, which is obviously red-shifted compared with that in Na2CO3 (∼1440 cm−1) (Fig. 4C, S76†). The crystal of the 1-Zn-CO32− host–guest complex was obtained through slowly diffusing THF vapor into the reaction system. SCXRD confirmed that one CO32− is tightly captured inside the tube cavity. Encapsulation of CO32− also causes induced-fit cavity deformations, with the size of the triangular window changing to 13.1 Å × 13.6 Å × 14.0 Å and the volume increasing to 377 Å3 compared to that of 1-Zn (Fig. 3B, S77†). Multiple hydrogen bonds and the existence of strong electrostatic interactions lead to a strong host–guest interaction between CO32− and the 1-Zn host (Fig. 4D). We speculate that the formed 1-Zn-CO32− host–guest complex is inactive and hinders the turnover of further CO2 conversion. Considering that Ca(II) ions can react with CO32− to produce water-insoluble CaCO3 (Ksp = 2.80 × 10−9), they have been used to grab the CO32− encapsulated inside cavity. Further experiments showed that after removal of CO32− encapsulated by the cavity, the tube 1-Zn can be regenerated and reused (Fig. 4B(e) and (f)). Although many coordination hosts that can fix CO2 by binding with carbonate have been reported,95–102 the newly formed strong metal–oxygen interactions make these hosts unable to be reused. Thus, tube 1-Zn represents a rare coordination host that can catalyze CO2 conversion like carbonic anhydrase. In short, efficient and mild CO2 conversion like the carbonic anhydrase can be realized by using an acid–base resistant coordination tube 1-Zn (Fig. 4A). While the efficiency is weaker, our complex is much cheaper and can tolerate harsh conditions.
A possible cavity-assisted CO2 conversion process was proposed as follows (Fig. S78†): (1) Zn(II) ions in tube 1-Zn are coordination unsaturated, which can coordinate with OH− to form Zn–OH units under basic conditions; (2) The Zn–OH group attacks the CO2 in solution to form HCO3−, which is the rate-determining step of the conversion of CO2. Compared to the OH− coordinated to harder Co(II) and Ni(II), the enhanced nucleophilicity of Zn–OH makes it more efficient to attack CO2; (3) HCO3− deprotonates into CO32− due to the pKa-shifting effect inside the cavity of the highly charged cationic host.109 In fact, DFT calculations show that the CO32−⊂1-Zn host–guest complexation energy was as high as −1349 kJ mol−1; (4) the CO32− sequestration by Ca(II) achieves the recycle of the catalyst and facilitates the reaction turnover.
Conclusions
A series of homo/heterometallic coordination assemblies have been synthesized from a tetraazacyclododecane-based ligand. Both self-assembly and structural transformations of coordination tubes/cages can be controlled in a cooperative manner using metal templates and auxiliary anions. The tubular complexes show adaptive cavities depending on the metallation of the ligand and the anions. The Zn-containing heteroleptic tubular complex not only shows impressive acid–base stability but also can catalyze the CO2 to CO32− conversion, standing for a rare example of an efficient and recyclable carbonic anhydrase-mimicking supramolecular system. Mechanistic studies confirm that the integration of multiple active metal sites and an open CO32−-binding pocket is important for the CO2 fixation. This work not only offers a new ligand candidate for the self-assembly of multi-variant coordination hosts, but also provides an important principle for the design of supramolecular catalysts toward CO2 fixation.
Data availability
All experimental and computational data associated with this work are available in the ESI.†
Author contributions
S. Li and C. Liu performed the experiments. F. Jiang and D. Yuan helped with data analysis. Q. Chen, Q.-F. Sun, and M. Hong designed the project. All authors were involved in the preparation of the manuscript.
Conflicts of interest
There are no conflicts to declare.
Acknowledgements
This work was supported by the National Key Research and Development Program of China (2021YFA1500400, 2018YFA0704500, and 2017YFA0206800), the Key Research Program of Frontier Science CAS (QYZDY-SSW-SLH025), and the National Natural Science Foundation of China (21871265, 21731006, and 21825107).
Notes and references
- C. Hepburn, E. Adlen, J. Beddington, E. A. Carter, S. Fuss, N. Mac Dowell, J. C. Minx, P. Smith and C. K. Williams, Nature, 2019, 575, 87–97 CrossRef CAS PubMed.
- G. Mezei, Chem, 2019, 5, 499–501 CAS.
- L. Liang, C. Liu, F. Jiang, Q. Chen, L. Zhang, H. Xue, H.-L. Jiang, J. Qian, D. Yuan and M. Hong, Nat. Commun., 2017, 8, 1233 CrossRef PubMed.
- L. Jiao, J. Zhu, Y. Zhang, W. Yang, S. Zhou, A. Li, C. Xie, X. Zheng, W. Zhou, S.-H. Yu and H.-L. Jiang, J. Am. Chem. Soc., 2021, 143, 19417–19424 CrossRef CAS PubMed.
- L. L. Ling, W. Yang, P. Yan, M. Wang and H. L. Jiang, Angew. Chem., Int. Ed., 2022, 61, e202116396 CAS.
- T. Ouyang, H.-J. Wang, H.-H. Huang, J.-W. Wang, S. Guo, W.-J. Liu, D.-C. Zhong and T.-B. Lu, Angew. Chem., Int. Ed., 2018, 57, 16480–16485 CrossRef CAS PubMed.
- K. K. Kannan, B. Notstrand, K. Fridborg, S. Lövgren, A. Ohlsson and M. Petef, Proc. Natl. Acad. Sci. USA, 1975, 72, 51–55 CrossRef CAS PubMed.
- P. Woolley, Nature, 1975, 258, 677–682 CrossRef CAS PubMed.
- E. Garcia-Espana, P. Gavina, J. Latorre, C. Soriano and B. A. Verdejo, J. Am. Chem. Soc., 2004, 126, 5082–5083 CrossRef CAS PubMed.
- B. Verdejo, J. Aguilar, E. Garcia-Espana, P. Gavina, J. Latorre, C. Soriano, J. M. Llinares and A. Domenech, Inorg. Chem., 2006, 45, 3803–3815 CrossRef CAS PubMed.
- J.-M. Chen, W. Wei, X.-L. Feng and T.-B. Lu, Chem.–Asian J., 2007, 2, 710–719 CrossRef PubMed.
- P. Mukherjee, M. G. B. Drew, M. Estrader and A. Ghosh, Inorg. Chem., 2008, 47, 7784–7791 CrossRef CAS PubMed.
- X. Liu, P. Du and R. Cao, Nat. Commun., 2013, 4, 2375 CrossRef PubMed.
- M. Fujita, M. Tominaga, A. Hori and B. Therrien, Acc. Chem. Res., 2005, 38, 369–378 CrossRef CAS PubMed.
- M. D. Ward, C. A. Hunter and N. H. Williams, Acc. Chem. Res., 2018, 51, 2073–2082 CrossRef CAS PubMed.
- S. Pullen and G. H. Clever, Acc. Chem. Res., 2018, 51, 3052–3064 CrossRef CAS PubMed.
- M. Pan, K. Wu, J.-H. Zhang and C.-Y. Su, Coord. Chem. Rev., 2019, 378, 333–349 CrossRef CAS.
- J. Dong, Y. Liu and Y. Cui, Acc. Chem. Res., 2021, 54, 194–206 CrossRef CAS PubMed.
- L. Chen, Q. Chen, M. Wu, F. Jiang and M. Hong, Acc. Chem. Res., 2015, 48, 201–210 CrossRef CAS PubMed.
- D. L. Caulder and K. N. Raymond, Acc. Chem. Res., 1999, 32, 975–982 CrossRef CAS.
- T. R. Cook and P. J. Stang, Chem. Rev., 2015, 115, 7001–7045 CrossRef CAS PubMed.
- Y. Lu, H.-N. Zhang and G.-X. Jin, Acc. Chem. Res., 2018, 51, 2148–2158 CrossRef CAS PubMed.
- E. G. Percastegui, T. K. Ronson and J. R. Nitschke, Chem. Rev., 2020, 120, 13480–13544 CrossRef CAS PubMed.
- S. M. Jansze and K. Severin, Acc. Chem. Res., 2018, 51, 2139–2147 CrossRef CAS PubMed.
- M. Yoshizawa and L. Catti, Acc. Chem. Res., 2019, 52, 2392–2404 CrossRef CAS PubMed.
- H. Kumari, C. A. Deakyne and J. L. Atwood, Acc. Chem. Res., 2014, 47, 3080–3088 CrossRef CAS PubMed.
- L. J. Jongkind, X. Caumes, A. P. T. Hartendorp and J. N. H. Reek, Acc. Chem. Res., 2018, 51, 2115–2128 CrossRef CAS PubMed.
- L.-J. Chen, H.-B. Yang and M. Shionoya, Chem. Soc. Rev., 2017, 46, 2555–2576 RSC.
- X. Jing, C. He, L. Zhao and C. Duan, Acc. Chem. Res., 2019, 52, 100–109 CrossRef CAS PubMed.
- S. Chakraborty and G. R. Newkome, Chem. Soc. Rev., 2018, 47, 3991–4016 RSC.
- A. Goswami, S. Saha, P. K. Biswas and M. Schmittel, Chem. Rev., 2020, 120, 125–199 CrossRef CAS PubMed.
- S. Durot, J. Taesch and V. Heitz, Chem. Rev., 2014, 114, 8542–8578 CrossRef CAS PubMed.
- Z. He, W. Jiang and C. A. Schalley, Chem. Soc. Rev., 2015, 44, 779–789 RSC.
- M. J. Hardie, Chem. Soc. Rev., 2010, 39, 516–527 RSC.
- S. Pullen, J. Tessarolo and G. H. Clever, Chem. Sci., 2021, 12, 7269–7293 RSC.
- Y. Li, H. Wang and X. Li, Chem. Sci., 2020, 11, 12249–12268 RSC.
- Y. Domoto, M. Abe, K. Yamamoto, T. Kikuchi and M. Fujita, Chem. Sci., 2020, 11, 10457–10460 RSC.
- Y. Domoto, M. Abe and M. Fujita, J. Am. Chem. Soc., 2021, 143, 8578–8582 CrossRef CAS PubMed.
- A. Kumar, R. Saha and P. S. Mukherjee, Chem. Sci., 2021, 12, 5319–5329 RSC.
- P. Howlader, S. Mondal, S. Ahmed and P. S. Mukherjee, J. Am. Chem. Soc., 2020, 142, 20968–20972 CrossRef CAS PubMed.
- J.-H. Zhang, H.-P. Wang, L.-Y. Zhang, S.-C. Wei, Z.-W. Wei, M. Pan and C.-Y. Su, Chem. Sci., 2020, 11, 8885–8894 RSC.
- K. Wu, K. Li, S. Chen, Y.-J. Hou, Y.-L. Lu, J.-S. Wang, M.-J. Wei, M. Pan and C.-Y. Su, Angew. Chem., Int. Ed., 2020, 59, 2639–2643 CrossRef CAS PubMed.
- X. Zhang, X. Dong, W. Lu, D. Luo, X.-W. Zhu, X. Li, X.-P. Zhou and D. Li, J. Am. Chem. Soc., 2019, 141, 11621–11627 CrossRef CAS PubMed.
- D. Luo, X.-Z. Wang, C. Yang, X.-P. Zhou and D. Li, J. Am. Chem. Soc., 2018, 140, 118–121 CrossRef CAS PubMed.
- M. D. Ludden, C. G. P. Taylor and M. D. Ward, Chem. Sci., 2021, 12, 12640–12650 RSC.
- M. D. Ludden, C. G. P. Taylor, M. B. Tipping, J. S. Train, N. H. Williams, J. C. Dorrat, K. L. Tuck and M. D. Ward, Chem. Sci., 2021, 12, 14781–14791 RSC.
- S. P. Argent, F. C. Jackson, H. M. Chan, S. Meyrick, C. G. P. Taylor, T. K. Ronson, J. P. Rourke and M. D. Ward, Chem. Sci., 2020, 11, 10167–10174 RSC.
- J. Tessarolo, H. Lee, E. Sakuda, K. Umakoshi and G. H. Clever, J. Am. Chem. Soc., 2021, 143, 6339–6344 CrossRef CAS PubMed.
- H. Wang, K. Wang, Y. Xu, W. Wang, S. Chen, M. Hart, L. Wojtas, L.-P. Zhou, L. Gan, X. Yan, Y. Li, J. Lee, X.-S. Ke, X.-Q. Wang, C.-W. Zhang, S. Zhou, T. Zhai, H.-B. Yang, M. Wang, J. He, Q.-F. Sun, B. Xu, Y. Jiao, P. J. Stang, J. L. Sessler and X. Li, J. Am. Chem. Soc., 2021, 143, 5826–5835 CrossRef CAS PubMed.
- Y. Li, S. S. Rajasree, G. Y. Lee, J. Yu, J.-H. Tang, R. Ni, G. Li, K. N. Houk, P. Deria and P. J. Stang, J. Am. Chem. Soc., 2021, 143, 2908–2919 CrossRef CAS PubMed.
- C. T. McTernan, T. K. Ronson and J. R. Nitschke, J. Am. Chem. Soc., 2021, 143, 664–670 CrossRef CAS PubMed.
- S. Sudan, R.-J. Li, S. M. Jansze, A. Platzek, R. Rudolf, G. H. Clever, F. Fadaei-Tirani, R. Scopelliti and K. Severin, J. Am. Chem. Soc., 2021, 143, 1773–1778 CrossRef CAS PubMed.
- K. Omoto, S. Tashiro and M. Shionoya, J. Am. Chem. Soc., 2021, 143, 5406–5412 CrossRef CAS PubMed.
- J.-F. Ayme, J.-M. Lehn, C. Bailly and L. Karmazin, J. Am. Chem. Soc., 2020, 142, 5819–5824 CrossRef CAS PubMed.
- L. S. Lisboa, J. A. Findlay, L. J. Wright, C. G. Hartinger and J. D. Crowley, Angew. Chem., Int. Ed., 2020, 59, 11101–11107 CrossRef CAS PubMed.
- S. Samantray, S. Krishnaswamy and D. K. Chand, Nat. Commun., 2020, 11, 880 CrossRef CAS PubMed.
- D. Liu, M. Chen, K. Li, Z. Li, J. Huang, J. Wang, Z. Jiang, Z. Zhang, T. Xie, G. R. Newkome and P. Wang, J. Am. Chem. Soc., 2020, 142, 7987–7994 CrossRef CAS PubMed.
- S. Oldknow, D. R. Martir, V. E. Pritchard, M. A. Blitz, C. W. G. Fishwick, E. Zysman-Colman and M. J. Hardie, Chem. Sci., 2018, 9, 8150–8159 RSC.
- M. Hardy, N. Struch, J. J. Holstein, G. Schnakenburg, N. Wagner, M. Engeser, J. Beck, G. H. Clever and A. Luetzen, Angew. Chem., Int. Ed., 2020, 59, 3195–3200 CrossRef CAS PubMed.
- A. N. Oldacre, A. E. Friedman and T. R. Cook, J. Am. Chem. Soc., 2017, 139, 1424–1427 CrossRef CAS PubMed.
- L. He, L.-X. Cai, M.-H. Li, G.-L. Zhang, L.-P. Zhou, T. Chen, M.-J. Lin and Q.-F. Sun, Chem. Sci., 2020, 11, 7940–7949 RSC.
- L. Yang, X. Jing, B. An, C. He, Y. Yang and C. Duan, Chem. Sci., 2018, 9, 1050–1057 RSC.
- S. Bai, L.-L. Ma, T. Yang, F. Wang, L.-F. Wang, F. E. Hahn, Y.-Y. Wang and Y.-F. Han, Chem. Sci., 2021, 12, 2165–2171 RSC.
- Y. Lu, D. Liu, Y.-J. Lin and G.-X. Jin, Chem. Sci., 2020, 11, 11509–11513 RSC.
- W. M. Hart-Cooper, C. Zhao, R. M. Triano, P. Yaghoubi, H. L. Ozores, K. N. Burford, F. D. Toste, R. G. Bergman and K. N. Raymond, Chem. Sci., 2015, 6, 1383–1393 RSC.
- A. J. Plajer, F. J. Rizzuto, L. K. S. von Krbek, Y. Gisbert, V. Martinez-Agramunt and J. R. Nitschke, Chem. Sci., 2020, 11, 10399–10404 RSC.
- S. M. Jansze, G. Cecot and K. Severin, Chem. Sci., 2018, 9, 4253–4257 RSC.
- T. Tsutsui, L. Catti, K. Yoza and M. Yoshizawa, Chem. Sci., 2020, 11, 8145–8150 RSC.
- X. Hu, S. Feng, J. Du, L. Shao, J. Lang, C. Zhang, S. P. Kelley, J. Lin, S. J. Dalgarno, D. A. Atwood and J. L. Atwood, Chem. Sci., 2020, 11, 12547–12552 RSC.
- E. O. Bobylev, D. A. Poole III, B. de Bruin and J. N. H. Reek, Chem. Sci., 2021, 12, 7696–7705 RSC.
- R. Sumida, Y. Tanaka, K. Niki, Y. Sei, S. Toyota and M. Yoshizawa, Chem. Sci., 2021, 12, 9946–9951 RSC.
- V. Marti-Centelles, R. L. Spicer and P. J. Lusby, Chem. Sci., 2020, 11, 3236–3240 RSC.
- C. Tan, J. Jiao, Z. Li, Y. Liu, X. Han and Y. Cui, Angew. Chem., Int. Ed., 2018, 57, 2085–2090 CrossRef CAS PubMed.
- J. Jiao, C. Tan, Z. Li, Y. Liu, X. Han and Y. Cui, J. Am. Chem. Soc., 2018, 140, 2251–2259 CrossRef CAS PubMed.
- J. Tian, L. Liu, K. Zhou, Z. Hong, Q. Chen, F. Jiang, D. Yuan, Q. Sun and M. Hong, Chem. Sci., 2020, 11, 9818–9826 RSC.
- G. Mezei, C. M. Zaleski and V. L. Pecoraro, Chem. Rev., 2007, 107, 4933–5003 CrossRef CAS PubMed.
- B. M. Ahmed and G. Mezei, Chem. Commun., 2017, 53, 1029–1032 RSC.
- M. Yoshizawa, M. Tamura and M. Fujita, Science, 2006, 312, 251–254 CrossRef CAS PubMed.
- W. Cullen, M. C. Misuraca, C. A. Hunter, N. H. Williams and M. D. Ward, Nat. Chem., 2016, 8, 231–236 CrossRef CAS PubMed.
- Q.-Q. Wang, S. Gonell, S. H. A. M. Leenders, M. Duerr, I. Ivanovic-Burmazovic and J. N. H. Reek, Nat. Chem., 2016, 8, 225–230 CrossRef CAS PubMed.
- H. Takezawa, K. Shitozawa and M. Fujita, Nat. Chem., 2020, 12, 574–578 CrossRef CAS PubMed.
- D. M. Kaphan, M. D. Levin, R. G. Bergman, K. N. Raymond and F. D. Toste, Science, 2015, 350, 1235–1238 CrossRef CAS PubMed.
- W. Cullen, A. J. Metherell, A. B. Wragg, C. G. P. Taylor, N. H. Williams and M. D. Ward, J. Am. Chem. Soc., 2018, 140, 2821–2828 CrossRef CAS PubMed.
- D. A. Poole III, S. Mathew and J. N. H. Reek, J. Am. Chem. Soc., 2021, 143, 16419–16427 CrossRef CAS PubMed.
- V. Martí-Centelles, A. L. Lawrence and P. J. Lusby, J. Am. Chem. Soc., 2018, 140, 2862–2868 CrossRef PubMed.
- J. Wang, T. A. Young, F. Duarte and P. J. Lusby, J. Am. Chem. Soc., 2020, 142, 17743–17750 CrossRef CAS PubMed.
- K. Li, K. Wu, Y. L. Lu, J. Guo, P. Hu and C. Y. Su, Angew. Chem., Int. Ed., 2022, 61, e202114070 CAS.
- H. Takezawa, T. Murase and M. Fujita, J. Am. Chem. Soc., 2012, 134, 17420–17423 CrossRef CAS PubMed.
- P. Mal, B. Breiner, K. Rissanen and J. R. Nitschke, Science, 2009, 324, 1697–1699 CrossRef CAS PubMed.
- S. Hasegawa, S. L. Meichsner, J. J. Holstein, A. Baksi, M. Kasanmascheff and G. H. Clever, J. Am. Chem. Soc., 2021, 143, 9718–9723 CrossRef CAS PubMed.
- D. M. Kaphan, F. D. Toste, R. G. Bergman and K. N. Raymond, J. Am. Chem. Soc., 2015, 137, 9202–9205 CrossRef CAS PubMed.
- J. Wei, L. Zhao, C. He, S. Zheng, J. N. H. Reek and C. Duan, J. Am. Chem. Soc., 2019, 141, 12707–12716 CrossRef CAS PubMed.
- L. J. Jongkind, J. A. A. W. Elemans and J. N. H. Reek, Angew. Chem., Int. Ed., 2019, 58, 2696–2699 CrossRef CAS PubMed.
- J. Guo, Y.-Z. Fan, Y.-L. Lu, S.-P. Zheng and C.-Y. Su, Angew. Chem., Int. Ed., 2020, 59, 8661–8669 CrossRef CAS PubMed.
- X.-L. Tang, W.-H. Wang, W. Dou, J. Jiang, W.-S. Liu, W.-W. Qin, G.-L. Zhang, H.-R. Zhang, K.-B. Yu and L.-M. Zheng, Angew. Chem., Int. Ed., 2009, 48, 3499–3502 CrossRef CAS PubMed.
- B. Wang, B. Ma, Z. Wei, H. Yang, M. Wang, W. Yin, H. Gao and W. Liu, Inorg. Chem., 2021, 60, 2764–2770 CrossRef CAS PubMed.
- S. D. Bian, J. H. Jia and Q. M. Wang, J. Am. Chem. Soc., 2009, 131, 3422–3423 CrossRef CAS PubMed.
- D. Sun, G.-G. Luo, N. Zhang, R.-B. Huang and L.-S. Zheng, Chem. Commun., 2011, 47, 1461–1463 RSC.
- C. Browne, W. J. Ramsay, T. K. Ronson, J. Medley-Hallam and J. R. Nitschke, Angew. Chem., Int. Ed., 2015, 54, 11122–11127 CrossRef CAS PubMed.
- W. A. Al Isawi, M. Zeller and G. Mezei, Inorg. Chem., 2021, 60, 13479–13492 CrossRef CAS PubMed.
- W. A. Al Isawi and G. Mezei, Molecules, 2021, 26, 3083 CrossRef CAS PubMed.
- X. Li, J. Wu, C. He, R. Zhang and C. Duan, Chem. Commun., 2016, 52, 5104–5107 RSC.
- A. Martinez-Camarena, A. Liberato, E. Delgado-Pinar, A. G. Algarra, J. Pitarch-Jarque, J. M. Llinares, M. Angeles Manez, A. Domenech-Carbo, M. G. Basallote and E. Garcia-Espana, Inorg. Chem., 2018, 57, 10961–10973 CrossRef CAS PubMed.
- A. Martinez-Camarena, P. A. Sanchez-Murcia, S. Blasco, L. Gonzalez and E. Garcia-Espana, Chem. Commun., 2020, 56, 7511–7514 RSC.
- J.-W. Wang, W.-J. Liu, D.-C. Zhong and T.-B. Lu, Coord. Chem. Rev., 2019, 378, 237–261 CrossRef CAS.
- J.-W. Wang, C. Hou, H.-H. Huang, W.-J. Liu, Z.-F. Ke and T.-B. Lu, Catal. Sci. Technol., 2017, 7, 5585–5593 RSC.
- F. Ibukuro, T. Kusukawa and M. Fujita, J. Am. Chem. Soc., 1998, 120, 8561–8562 CrossRef CAS.
- G. Liu, Y. Di Yuan, J. Wang, Y. Cheng, S. B. Peh, Y. Wang, Y. Qian, J. Dong, D. Yuan and D. Zhao, J. Am. Chem. Soc., 2018, 140, 6231–6234 CrossRef CAS PubMed.
- C. Ngai, H.-T. Wu, B. da Camara, C. G. Williams, L. J. Mueller, R. R. Julian and R. J. Hooley, Angew. Chem., Int. Ed., 2022, 61, e202117011 CrossRef CAS PubMed.
|
This journal is © The Royal Society of Chemistry 2022 |