DOI:
10.1039/D1SC07157B
(Edge Article)
Chem. Sci., 2022,
13, 3533-3538
π-Conjugated redox-active two-dimensional polymers as organic cathode materials†
Received
22nd December 2021
, Accepted 2nd March 2022
First published on 8th March 2022
Abstract
Redox-active two-dimensional polymers (RA-2DPs) are promising lithium battery organic cathode materials due to their regular porosities and high chemical stabilities. However, weak electrical conductivities inherent to the non-conjugated molecular motifs used thus far limit device performance and the practical relevance of these materials. We herein address this problem by developing a modular approach to construct π-conjugated RA-2DPs with a new polycyclic aromatic redox-active building block PDI-DA. Efficient imine-condensation between PDI-DA and two polyfunctional amine nodes followed by quantitative alkyl chain removal produced RA-2DPs TAPPy-PDI and TAPB-PDI as conjugated, porous, polycrystalline networks. In-plane conjugation and permanent porosity endow these materials with high electrical conductivity and high ion diffusion rates. As such, both RA-2DPs function as organic cathode materials with good rate performance and excellent cycling stability. Importantly, the improved design enables higher areal mass-loadings than were previously available, which drives a practical demonstration of TAPPy-PDI as the power source for a series of LED lights. Collectively, this investigation discloses viable synthetic methodologies and design principles for the realization of high-performance organic cathode materials.
Introduction
Organic energy storage materials offer the prospect of high gravimetric energy storage with rapid charging and discharging rates without the need for costly, energy intensive and destructive resource acquisition.1–3 Redox-active two-dimensional polymers (RA-2DPs, also referred to as covalent organic frameworks4,5) are among the most promising candidates as faradaic charge storage materials because of their tailored topologies and resultant regular porosities.6–12 However, RA-2DPs have not reached their potential because the typical structures exhibit poor electrical conductivity13,14 and their electro-inactive solubilizing moieties reduce the gravimetric energy storage performance.15 This realization has led researchers to explore incorporating conducting additives16–18 and exfoliating 2DPs into the few-layer limit19 with the goal of increasing electrochemical accessibility. While these investigations demonstrate that increased electrochemical accessibility can improve faradaic performance in RA-2DPs, these strategies require laborious post-processing, expensive substrates and valuable additives. More importantly, the mass-loading of active materials in those devices is generally low9 due to poor intrinsic electrical conductivity, which limits their deployment in practical devices.
In this study, we systematically examine the molecular design principles to improve faradaic charge storage in RA-2DPs. Specifically, we engineer a new redox-active motif: perylene diimide dibenzoaldehyde (PDI-DA, Fig. 1). PDI subunits can reversibly accept two electrons and have been widely used as acceptor materials for photovoltaics,20 photodetectors,21 redox flow batteries22 and pseudocapacitors.23 Conventionally, diimide-containing RA-2DPs are mostly extended through the imide nitrogen,24 resulting in non-conjugated structures with limited electrical conductivity. Furthermore, these RA-2DPs frequently incorporate contorted phenyl spacers that diminish in-plane conjugation.15,25 The PDI-DA unit studied here is bay connected through a fused aromatic ring that simultaneously addresses both limitations of previous molecular designs. To demonstrate the generality of these findings, we study two RA-2DPs prepared from different polyfunctional amine nodes: 1,3,6,8-tetrakis(4-aminophenyl)pyrene (TAPPy) and 1,3,5-tris(4-aminophenyl)benzene (TAPB). Following polymerization into crystalline, porous networks, the solubilizing alkyl side chains are quantitatively removed via thermolysis without disrupting the periodic network structures. This chemical transformation improves battery performance by decreasing the electrochemically non-participating mass and enhancing the lithium-ion permeability of the networks. As a result, RA-2DPs produced using the design principles uncovered here approach their theoretical capacity with excellent cycling stability.
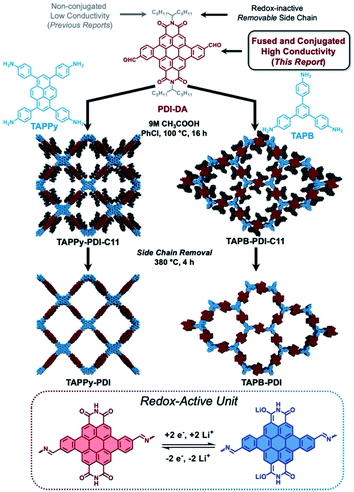 |
| Fig. 1 Structure design of redox-active conjugated building blocks PDI-DA (top), synthesis of TAPPy-PDI (left) and TAPB-PDI (right) and depiction of their redox activity (bottom). | |
Results and discussion
Synthesis of RA-2DPs
Fig. 1 displays the synthesis of RA-2DPs. Details for the synthesis of PDI-DA can be found in the ESI (Section 2†). RA-2DPs TAPPy-PDI-C11 and TAPB-PDI-C11 were then synthesized by acid catalyzed imine-condensation between PDI-DA and the corresponding polyamines. Both RA-2DPs were isolated in high yield (>90%) as crystalline, high surface area powders (vide infra). While performing thermogravimetric analysis (TGA) of these two polymers, we observed a ∼30% sample mass loss for both 2DPs at ∼380 °C (Fig. S1†). Previously, we have attributed this mass loss to thermal removal of the solubilizing side chains.26 As such, alkyl chains were removed by heating at 380 °C under vacuum for 4 hours to yield TAPPy-PDI and TAPB-PDI, without disrupting the 2DP structures or imine-polymerization chemistry as determined by elemental analysis, infrared spectroscopy (see ESI†), X-ray diffraction, and nitrogen porosimetry.
Characterization of RA-2DPs
Fourier-transform infrared (FT-IR) spectroscopy shows that imine-condensation proceeded efficiently with high consumption of monomer functionality and thermolytic cleavage of the alkyl chains did not damage other chemical motifs within the networks. To better understand our FT-IR results, we prepared an imine-linked PDI-containing model compound (S4) by condensing PDI-DA with aniline under the same reaction conditions. Following imine condensation, the C
O stretch (1688 cm−1) of the aldehyde and the N–H vibrational feature (3200 cm−1) of the amine were found to disappear in both RA-2DPs and the model compound S4 (Fig. S2†). The imine C
N stretch (∼1620 cm−1) and the imide C
O stretch (∼1607 cm−1) are observed to overlap, which prevents definitive observation of the appearance of the expected polymerization chemistry.15 Collectively, the FT-IR changes observed following polymerization confirm the imine-condensation product of aniline and PDI-DA. Thermolytic alkyl chain removal was verified by the disappearance of the alkyl vibrational modes (2800–3000 cm−1) and concomitant increase of the N–H peak (3200 cm−1). Importantly, the features associated with imide and imine moieties are found to be unaffected by the thermolysis procedure. This is consistent with the finding that the mass retention following thermolysis (70%) agrees well with the calculated mass retention of losing the side chains (73%). The FT-IR features observed throughout these chemical transformations correlate well with calculated vibrational spectra for each of these species (Fig. S3†).
Powder X-ray diffraction (PXRD) reveals that the TAPPy-PDI-C11 and TAPB-PDI-C11 and their thermolysis products were all isolated as polycrystalline powders (Fig. 2A). For both TAPPy-PDI-C11 and TAPB-PDI-C11, sharp diffraction features were observed at low two theta values. We assigned these features as the 〈100〉 Bragg scattering features of a layered tetragonal and hexagonal network for TAPPy-PDI-C11 and TAPB-PDI-C11, respectively. For both networks we also observed a diffuse scattering feature at high two theta values that we assign to the interlayer spacing between 2DP sheets in the solid-state. For TAPPy-PDI-C11, we find that the experimental pattern matches well with a simulated C2/m framework with lattice parameters of a = b = 44.0 Å, c = 2.8 Å with sharp diffraction features at 2.9°, 3.9° and 5.6° that correspond to the 〈100〉, 〈200〉 and 〈220〉 Bragg direction, respectively. For TAPB-PDI-C11, a simulated P6 framework is well matched with lattice parameters of a = b = 46.8 Å, c = 3.5 Å with sharp diffraction features at 2.9°, and 4.4° that correspond to the reflections along the 〈100〉 and 〈200〉 planes. In both cases, the observed distances match well with the expected distances of the spatial distribution between the nodes. Following thermolysis both RA-2DPs exhibited practically unchanged diffraction patterns, which demonstrates that the structurally regular 2DP network is unperturbed after the side chain removal. All the experimental PXRD patterns were refined with finite crystal size broadening that revealed an average crystallite size >50 nm, consistent with other reports of high-quality polycrystalline 2DP powders.27 These results are consistent with direct imaging via scanning electron microscope showing that RA-2DPs are isolated as polycrystalline powders (Fig. S5†).
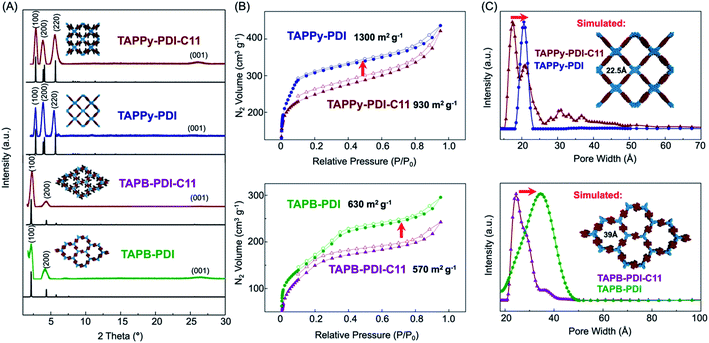 |
| Fig. 2 (A) Background subtracted experimental (colored) and predicted (black) PXRD patterns, (B) nitrogen adsorption isotherms and (C) pore size distribution of RA-2DPs. | |
Nitrogen sorption isotherms in Fig. 2B establish that all four RA-2DPs were porous polymer networks. High Brunauer–Emmett–Teller (BET) surface areas of 930 m2 g−1 and 570 m2 g−1 were extracted for TAPPy-PDI-C11 and TAPB-PDI-C11, respectively. Pore size distributions (Fig. 2C) were centered at 17 Å for TAPPy-PDI-C11 and 24 Å for TAPB-PDI-C11. We attributed the relatively broad distribution to the disordered packing of the alkyl chains in the 2DP structures. Following thermolysis to remove the alkyl side chains, the BET surface areas of TAPPy-PDI and TAPB-PDI were increased to 1300 m2 g−1 and 630 m2 g−1. Increases in the BET surface area were accompanied by narrowing and increasing pore size distributions from 17 Å to 21 Å for TAPPy-PDI and 24 Å to 35 Å for TAPB-PDI, which correlates well with the structures determined from PXRD. Taken together, these results reveal that the RA-2DPs, in all forms, are isolated as structurally regular, permanently porous networks.
Electrochemical performance
Using these four crystalline, porous RA-2DPs, we performed comparative electrochemical experiments with lithium metal half-cells. The working electrodes were fabricated by drop casting a slurry of 70 wt% RA-2DPs, 20 wt% carbon black (as conductive agent, see Fig. S15† for lower mass loadings of conductive additive) and 10 wt% polyvinylidene fluoride (PVDF; as binder) in N-methyl-2-pyrrolidone onto a carbon paper. We then assembled the cell using working electrodes and lithium metal in 2032 coin cells with 1 M LiPF6 in diethyl carbonate (DEC)/ethylene carbonate (EC) (1
:
1 vol) electrolyte. Cyclic voltammetry (CV) was first conducted on four RA-2DP electrodes with a scan rate of 1 mV s−1 (Fig. 3A). All the RA-2DPs exhibited fully reversible peaks centered at 2.2–2.3 V vs. Li/Li+, corresponding to the redox reaction from PDI subunits. Under the same scan rate, TAPPy-PDI and TAPB-PDI showed much higher current and slightly more positive redox potentials than TAPPy-PDI-C11 and TAPB-PDI-C11. This enhanced electrochemical performance after alkyl chain removal is likely attributed to the reduction of electrochemically inactive components, the increase of electrical conductivity and lithium-ion diffusion within the network (vide infra). It is noteworthy that the alkyl chain removal protocol should be applicable to many other RA-2DPs with solubilizing side chains to improve their device performance.15,25 Because the RA-2DPs without the alkyl chains exhibit much higher capacity, we focused on investigating the device performance of TAPPy-PDI and TAPB-PDI.
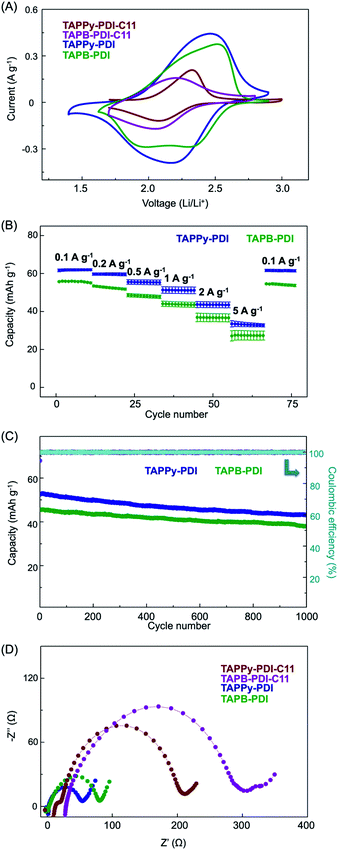 |
| Fig. 3 The electrochemical performance of RA-2DPs. (A) CV profiles at 1 mV s−1 in 1.0 M LiPF6 in DEC/EC (1 : 1 vol) electrolyte, (B) rate performance at different discharge rates, (C) cycling stability at 0.5 A g−1 and coulombic efficiency (TAPPy-PDI in purple circle and TAPB-PDI in cyan square) over 1000 cycles, and (D) EIS of RA-2DPs. | |
The rate performance of TAPPy-PDI and TAPB-PDI were evaluated under different discharge rates from 0.1 A g−1 to 5 A g−1 (Fig. 3B). TAPPy-PDI delivered an initial capacity of 61 mA h g−1 at 0.1 A g−1 (1.5C), corresponding to 96% of the theoretical capacity. TAPB-PDI, on the other hand, exhibited slightly lower capacity (56 mA h g−1) at the same rate, corresponding to 83% of its theoretical capacity. Both polymers showed outstanding rate performance due to their conjugated and porous structures. Even with a high current density of 5 A g−1 (80C), TAPPy-PDI and TAPB-PDI still retained 55% and 44% of their theoretical capacity, respectively. Such retention rates are much higher than those of conventional lithium-ion cathode materials.1 These results show that we are able to fully unlock all the redox functional groups and significantly improve the rate performance by constructing the fully π-conjugated and porous network structures (see Table S1† for comparison with other materials).
Beyond the excellent rate performance, both RA-2DPs also possessed high cycling stability (Fig. 3C). At 0.5 A g−1 (7.5C), TAPPy-PDI showed a capacity of 52 mA h g−1 and an exceptional capacity retention of 83% after 1000 cycles, with an average coulombic efficiency of 100%. TAPB-PDI exhibited a capacity of 45 mA h g−1 with 84% capacity retention after 1000 cycles at the same discharging rate. This superb cycling stability for both RA-2DPs can be attributed to the chemically and structurally robust two-dimensional networks.
To better understand the excellent device performance of TAPPy-PDI and TAPB-PDI, we performed electrochemical impedance spectroscopy (EIS) on all four 2DPs (Fig. 3D). The charge-transfer resistance (Rct) of TAPPy-PDI and TAPB-PDI were determined as 57 Ω and 80 Ω, respectively, which are much lower than 2DPs with alkyl chains and those of similar polymer networks.17 These low Rct values of TAPPy-PDI and TAPB-PDI reveal high electrical conductivity (Table S2†) within the networks after alkyl chain removal, which may result from the full in-plane conjugation as well as the intimate π–π stacking of 2D sheets, as indicated by structural characterization data. To evaluate the lithium-ion diffusion within the networks, we analyzed the CV profiles at different scan rates for all four RA-2DPs. Using the Randles–Ševčík equation, the diffusion coefficient for TAPPy-PDI, TAPB-PDI, TAPPy-PDI-C11 and TAPB-PDI-C11 were determined to be 4.6 × 10−9 cm2 s−1, 3.6 × 10−9 cm2 s−1, 1.7 × 10−10 cm2 s−1 and 4.7 × 10−11 cm2 s−1, respectively. These higher diffusion coefficients for both RA-2DPs without the alkyl chains likely result from the immense porosities of these networks, which facilitates lithium-ion diffusion. We attribute the better electrochemical performance of TAPPy-PDI than TAPB-PDI to the higher electrical conductivity and higher ionic diffusivity of TAPPy-PDI. Such high electrical conductivity and high lithium-ion diffusivity of TAPPy-PDI allows us to investigate the battery performance of cells with higher active material loading. As shown in Fig. S15,†TAPPy-PDI battery with >80% active material portion exhibited similar performance at charging rates below 10C. In addition, the cell with 80% active loading showed exceptional cycling stability, with a capacity retention of 83% after 1000 cycles at 0.5 A g−1 (Fig. S16†).
Necessity of the 2D crystalline structure
To demonstrate the necessity of the porous, two-dimensional and crystalline structure, we synthesized an amorphous TAPPy-PDI (amp-TAPPy-PDI) using cis-PDI-DA and TAPPy under the same protocol we developed (ESI, Section 4.3†). Amp-TAPPy-PDI exhibited no Bragg scattering and no BET surface area due to the amorphous structure. Despite the same chemical composition, amp-TAPPy-PDI exhibited much lower capacity (37 mA h g−1 at 0.1 A g−1), rate performance (12 mA h g−1 at 0.5 A g−1) compared to TAPPy-PDI. Correspondingly, the diffusion coefficient of amp-TAPPy-PDI was determined to be 1.2 × 10−10 cm2 s−1, which is an order of magnitude lower than of the structurally regular TAPPy-PDI. Therefore, we attribute the outstanding performance of TAPPy-PDI and TAPB-PDI to both high electrical conductivities resulting from the π-conjugated structures and the enhanced ion diffusion rates from the high porosity.
Practical demonstration
Given TAPPy-PDI's superior device performance, we next evaluated it as high mass-loading charge storage media for practical applications. TAPPy-PDI was fabricated into electrodes with a mass-loading of ∼5 mg cm−2. Remarkably, the coin cells with high mass-loading electrodes still exhibited similar capacity (52 mA h g−1 at 0.2 A g−1) to the lower mass-loading cells. We then used this coin cell battery to power up a light-emitting diode (LED) array to examine its power ability. As shown in Fig. 4A, the array was constructed with ten red LEDs and six orange LEDs. Using a potentiostat, the working currents of the red and orange LED bulbs were determined to be 0.1–0.2 mA. The forward voltage of red and orange LEDs was determined to be 2.0 V and 2.3 V respectively. Accordingly, the overall working current for the array is around 1.6–3.2 mA, corresponding to 5–10C of the battery. After being charged to 3 V at 5C, the battery was able to light up the array for 10 minutes (Fig. 4B and ESI video†). To note, the red LEDs were able to last even longer because of the lower required forward voltage. Therefore, we demonstrated the practical applications of these fully π-conjugated RA-2DPs by high mass-loading electrode coin cells.
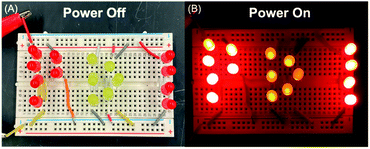 |
| Fig. 4 Practical demonstration of TAPPy-PDI electrode coin cells: (A) power off and (B) power on status of the LED array. | |
Conclusions
To conclude, we have designed and synthesized new redox-active two-dimensional polymers by an efficient two-step approach with PDI-DA as the redox-active building block. All RA-2DPs were π-conjugated, polycrystalline and exhibited high porosity and high electrical conductivity. After alkyl chain removal, TAPPy-PDI and TAPB-PDI showed great potential as cathode materials in lithium batteries with good rate performance and excellent cycling stability. We attribute this to both high electrical conductivity and great ion diffusion rate, resulting from the in-plane conjugated and porous network structures. Finally, we demonstrated the practical applications of these new RA-2DPs by high mass-loading cells and using them to power up a LED array. Collectively, this study highlights the critical design principles and synthetic strategies to produce RA-2DPs as high-performance organic cathode materials.
Data availability
Detailed experimental procedures are included in the ESI.†
Author contributions
Conceptualization: Z. J., Q. C. and C. N.; methodology: Z. J. and Q. C.; investigation and formal analysis: Z. J., Q. C., A. M. E. and J. G.; validation: R. Z., S. T. B. and F. W.; writing – original draft: Z. J., Q. C. and A. M. E.; writing – review & editing: Y. Y. and C. N.; funding acquisition and supervision: L. V., Y. Y. and C. N.
Conflicts of interest
There are no conflicts to declare.
Acknowledgements
Primary support for this research was provided by the National Science Foundation under award DMR-2002634. The Columbia University Shared Materials Characterization Laboratory was used extensively for this research. We are grateful to Columbia University for support of this facility. C. N. thanks Sheldon and Dorothea Buckler for their generous support. A. M. E. is supported by the Schmidt Science Fellows, in partnership with the Rhodes Trust. We thank Dr Xiao Xiao and Dr Jingjing Yang for valuable discussions.
Notes and references
- Y. Lu and J. Chen, Nat. Rev. Chem., 2020, 4, 127–142 CrossRef CAS.
- H. Lyu, X.-G. Sun and S. Dai, Adv. Energy Sustain. Res., 2021, 2, 2000044 CrossRef.
- M. E. Bhosale, S. Chae, J. M. Kim and J. Y. Choi, J. Mater. Chem. A, 2018, 6, 19885–19911 RSC.
- N. W. Ockwig, A. P. Co, M. O. Keeffe, A. J. Matzger and O. M. Yaghi, Science, 2005, 310, 1166–1171 CrossRef PubMed.
- K. Geng, T. He, R. Liu, S. Dalapati, K. T. Tan, Z. Li, S. Tao, Y. Gong, Q. Jiang and D. Jiang, Chem. Rev., 2020, 120, 8814–8933 CrossRef CAS PubMed.
- A. M. Evans, M. J. Strauss, A. R. Corcos, Z. Hirani, W. Ji, L. S. Hamachi, X. Aguilar-Enriquez, A. D. Chavez, B. J. Smith and W. R. Dichtel, Chem. Rev., 2022, 122, 442–564 CrossRef CAS PubMed.
- C. S. Diercks and O. M. Yaghi, Science, 2017, 355, eaal1585 CrossRef PubMed.
- Y. Zhang, S. N. Riduan and J. Wang, Chem.–Eur. J., 2017, 23, 16419–16431 CrossRef CAS PubMed.
- V. Singh and H. R. Byon, Mater. Adv., 2021, 2, 3188–3212 RSC.
- S. Kandambeth, V. S. Kale, O. Shekhah, H. N. Alshareef and M. Eddaoudi, Adv. Energy Mater., 2021, 2100177 Search PubMed.
- Z. Meng, J. Luo, W. Li and K. A. Mirica, J. Am. Chem. Soc., 2020, 142, 21656–21669 CrossRef CAS PubMed.
- Z. Wang, S. Zhang, Y. Chen, Z. Zhang and S. Ma, Chem. Soc. Rev., 2020, 49, 708–735 RSC.
- N. Huang, P. Wang and D. Jiang, Nat. Rev. Mater., 2016, 1, 16068 CrossRef CAS.
- Y. Jin, Y. Hu and W. Zhang, Nat. Rev. Chem., 2017, 1, 1–11 CrossRef.
- S. Jhulki, C. H. Feriante, R. Mysyk, A. M. Evans, A. Magasinski, A. S. Raman, K. Turcheniuk, S. Barlow, W. R. Dichtel, G. Yushin and S. R. Marder, ACS Appl. Energy Mater., 2021, 4, 350–356 CrossRef CAS.
- C. R. Mulzer, L. Shen, R. P. Bisbey, J. R. McKone, N. Zhang, H. D. Abruña and W. R. Dichtel, ACS Cent. Sci., 2016, 2, 667–673 CrossRef CAS PubMed.
- E. Vitaku, C. N. Gannett, K. L. Carpenter, L. Shen, H. D. Abruña and W. R. Dichtel, J. Am. Chem. Soc., 2020, 142, 16–20 CrossRef CAS PubMed.
- F. Xu, S. Jin, H. Zhong, D. Wu, X. Yang, X. Chen, H. Wei, R. Fu and D. Jiang, Sci. Rep., 2015, 5, 1–6 Search PubMed.
- S. Wang, Q. Wang, P. Shao, Y. Han, X. Gao, L. Ma, S. Yuan, X. Ma, J. Zhou, X. Feng and B. Wang, J. Am. Chem. Soc., 2017, 139, 4258–4261 CrossRef CAS PubMed.
- Y. Zhong, M. T. Trinh, R. Chen, G. E. Purdum, P. P. Khlyabich, M. Sezen, S. Oh, H. Zhu, B. Fowler, B. Zhang, W. Wang, C. Y. Nam, M. Y. Sfeir, C. T. Black, M. L. Steigerwald, Y. L. Loo, F. Ng, X. Y. Zhu and C. Nuckolls, Nat. Commun., 2015, 6, 1–8 Search PubMed.
- Y. Zhong, T. J. Sisto, B. Zhang, K. Miyata, X.-Y. Zhu, M. L. Steigerwald, F. Ng and C. Nuckolls, J. Am. Chem. Soc., 2017, 139, 5644–5647 CrossRef CAS PubMed.
- M. Milton, Q. Cheng, Y. Yang, C. Nuckolls, R. Hernández Sánchez and T. J. Sisto, Nano Lett., 2017, 17, 7859–7863 CrossRef CAS PubMed.
- J. C. Russell, V. A. Posey, J. Gray, R. May, D. A. Reed, H. Zhang, L. E. Marbella, M. L. Steigerwald, Y. Yang, X. Roy, C. Nuckolls and S. R. Peurifoy, Nat. Mater., 2021, 20, 1136–1141 CrossRef CAS PubMed.
- S. Jin, K. Furukawa, M. Addicoat, L. Chen, S. Takahashi, S. Irle, T. Nakamura and D. Jiang, Chem. Sci., 2013, 4, 4505–4511 RSC.
- Y. Liang, Z. Chen, Y. Jing, Y. Rong, A. Facchetti and Y. Yao, J. Am. Chem. Soc., 2015, 137, 4956–4959 CrossRef CAS PubMed.
- S. R. Peurifoy, J. C. Russell, T. J. Sisto, Y. Yang, X. Roy and C. Nuckolls, J. Am. Chem. Soc., 2018, 140, 10960–10964 CrossRef CAS PubMed.
- M. C. Daugherty, E. Vitaku, R. L. Li, A. M. Evans, A. D. Chavez and W. R. Dichtel, Chem. Commun., 2019, 55, 2680–2683 RSC.
Footnotes |
† Electronic supplementary information (ESI) available. See DOI: 10.1039/d1sc07157b |
‡ These authors contributed equally to this work. |
|
This journal is © The Royal Society of Chemistry 2022 |
Click here to see how this site uses Cookies. View our privacy policy here.