Naphtha catalytic cracking to olefins over zirconia–titania catalyst†
Received
19th July 2021
, Accepted 22nd September 2021
First published on 27th September 2021
Abstract
A zirconia–titania-based catalyst was synthesized by a co-participation method to study the catalytic cracking of heavy naphtha (dodecane) into high value-added olefins. The nanocrystal-sized catalysts were characterized and the cluster consisted of tetragonal ZrO2 incorporated into anatase TiO2. The catalyst was stable from hydrogen reduction while the surface acidity was increased as more ZrO2 was incorporated into the TiO2 framework. The catalytic cracking of naphtha was increased as the moderate catalytic acidity strength increased and the 50% Zr–Ti oxide catalyst delivered higher conversion between 85–95% with olefin yield around 55–60%. The surface acidity of the Zr–Ti catalyst promoted more cyclization reactions to produce benzene, toluene, and xylene (BTX). The study investigated steam catalytic cracking, which was slightly improved using a lower acidic Zr–Ti catalyst, while the propylene/ethylene ratio was unchanged. The time-on-stream study highlighted the stability of the catalyst with ZrO2 incorporated into the TiO2 framework, to promote olefin yield from naphtha catalytic cracking.
1. Introduction
Naphtha obtained from fossil fuels for transportation is affected by electrical vehicle policies (EVs).1 The utilization of naphtha feedstocks in high added-value chemicals will be increased for the long-term conversion to the higher demand chemicals such as olefins and aromatics.2 The catalytic cracking of naphtha is one of the key processes for producing olefins (ethylene, propylene, and butenes), which are essential intermediate components for the petrochemical industry.3,4 There are two known methods in the industry that are applied to convert naphtha with and without catalysts. Steam cracker units are commercially utilized in the petrochemical industry and these units are unselective4 and operate at high temperatures in the range of 750–820 °C with higher GHGs emission.5
Several studies have investigated the use of several catalysts for naphtha cracking to produce light olefins.5–7 The zeolite catalysts based on USY zeolite,4 MFI,7 nano zeolite of BEA,6 ZSM-22,8 La, Ce-ZSM-12,9 La-MTT,10 and SSZ-54 (ref. 11) were studied and considered to be reactive catalysts due to the substantially high acidic catalyst characteristics for the hydrocarbon dissociation reactions and shape selectivity.6,10,12 Several zeolite catalysts were found to have low catalytic conversion cycles and stability due to coke deposition in the internal pores.9,10,13 Other zeolite catalysts showed the dealumination of the zeolite framework in the presence and absence of steam during catalytic cracking, which is an irreversible defect in the zeolite framework and reduced the catalytic ability of naphtha conversion.7,14 Zeolite impregnated with phosphorus was studied in steam-assisted methods at different steam/HC ratios for the catalytic cracking of hexane and conversion to olefin at 650 °C,15,16 and they concluded that more olefins were produced in the presence of steam and the cyclization reaction over the catalyst was less favorable. The higher steam/HC ratios in the range of 0.5 to 1 showed considerable benefits to reduce coke participation and the catalytic cracking would contribute to the promotion of more primary cracking to produce light olefins.14
Several studies reported that bimetallic and trimetallic oxide catalysts were found to be reactive catalytic materials for naphtha cracking. However, the catalysts based on catalytic oxidative cracking, such as V–Sr–Al,17 Mo–Li–MgO,18,19 CaMn–NaW,20 SrMn–NaW,20 Li/Mg/Mo,18 Li/Mg/Bi,18 BaFeAl (ref. 21) and NaW/BaFe,21 showed higher yields of light naphtha to olefin. These catalysts are oxygen carriers and known as redox catalysts, which makes them reactive for the C–H and C–C bond-breaking of naphtha to produce olefins, but with a time-on-stream limited to few minutes. On the other hand, non-redox catalysts are reactive catalysts such as Zr–Ti in Al2O3 for n-decane cracking at 600–750 °C,22 Pt–S–Zr, Al–S–Zr and Ga–S–Zr for isomerization reactions23,24 and Zr–Ti catalyst dehydrogenation of ethylbenzene reaction.25 These catalysts have significant surface basic–acidic sites26,27 for hydrocarbon reactions and among them are the zirconia–titania-based catalysts. They have moderate catalytic acidic strength which can be improved with increases in the ZrO2 concentration in the TiO2 framework.26,28,29 The pyridine adsorption analysis demonstrated that the catalyst Zr–Ti oxide consists of Brønsted and Lewis acid sites as measured by FTIR,26 which suggested that these acidic catalysts have good potential to be utilized in hydrocarbon cracking reactions.30
There are a few studies that utilized ZrO2/TiO2 catalyst for hexane/hexene cyclization.29 Also, in these studies, a third metal was added to the catalyst to improve its acidity, including Mo, Mn, Fe, and W over Zr–Ti–Al for n-decane conversion at high temperature (600–750 °C) and pressure (2.5 MPa).22 It was observed that adding the extra metals led to increasing the surface acid sites.22,29 Although some studies have tested the bimetallic catalyst of ZrO2–TiO2, its role in naphtha cracking to olefins is not fully understood.29 In this study, dodecane was employed as a model compound for heavy naphtha to thoroughly explore hydrocarbon cracking over the bimetallic catalyst of ZrO2–TiO2. The effect of steam on the catalyst and the selectivity towards ethylene and propylene were also studied.
2. Experimental
2.1 Catalyst preparation and characterization
The chemical materials were acquired from Sigma Aldrich. Titanium chloride (TiCl4) and zirconyl chloride octahydrate (ZrOCl2·8H2O) were used for catalyst synthesis. The catalyst precursor was synthesized using a co-precipitation method,31 and to prepare 50% Zr–Ti oxide, TiCl4 (8.0 g) was added dropwise to the ammonia solution (200 ml, 0.15 N) with rapid stirring, then 7.2 g of ZrOCl2 was added. Dilute ammonia solution (1N) was added to adjust the pH to 7 and the solution was stirred continuously for 6 hours at room temperature. The obtained solution was centrifuged to separate the generated powder. The catalyst precursor is washed with RO water 4 times and dried in the oven at 120 °C for 18 hours. Finally, the powder was calcined at 720 °C for 5 hours.
2.2 Catalyst characterization
The prepared Zr–Ti oxide catalysts were characterized by transmission electron microscopy (TEM) using a JEM-2100F to determine the catalyst particle topography. X-ray diffraction (XRD) was performed using a Panalytical X'Pert PRO diffractometer to determine the crystallinity of the Zr–Ti oxide catalyst. The hydrogen temperature-programmed reduction (H2-TPR) analysis was carried out using a Micromeritics AutoChem 2910 instrument. Ammonia temperature-programmed desorption (NH3-TPD) was used to analyse the catalyst acidity using a CATLAB-HIDEN unit. The catalyst textural properties were investigated via N2 adsorption by utilizing the Micromeritics ASAP 2420. The pyridine-FTIR was applied to determine Brønsted and Lewis acid sites of the catalyst and the concentration of each acidic site species.32 The Zr–Ti oxide catalyst surface acidity was measured via Fourier transform infrared (FTIR) spectroscopy using a Nicolet-6700 spectrometer.33 A weight of 30 mg of catalyst powder was pressed into a self-supported wafer and placed inside a ZnSe IR cell at 400 °C for 45 min under vacuum to remove the water from the catalyst. Then the sample wafer was cooled to 150 °C and the FTIR spectrum of pyridine vapour was introduced at 150 °C to determine the interaction with the catalyst and the nature of the acidity. The Brønsted and Lewis acid sites were quantified from the peaks of the adsorption of pyridine at 1544 cm−1 and 1450 cm−1,34 respectively.
2.3 The catalytic reactor apparatus
The catalytic cracking apparatus consisted of a packed bed reactor installed in a high-temperature vertical furnace as shown in Fig. 1. The catalyst bed weight was 1.2 g and bed volume was 3 cm3, with a pre-heating zone of quartz chips on the top of the catalyst bed and no catalyst dilution. For thermal cracking without catalyst, quartz wool was applied and adjusted with the pre-heating zone. Dodecane was used as a model compound of heavy naphtha and was injected via an HPLC pump at a liquid hourly space velocity (LHSV) of 0.5 h−1. In the steam catalytic cracking case, another HPLC pump was used to inject water at a similar LHSV to the hydrocarbon and an HC/steam ratio of 1
:
1 was applied. The furnace temperature was adjusted based on the required reaction temperature in the range of 550–625 °C. The products were sent to a cold trap at a temperature of −10 °C and gas was sent to a gas chromatograph (GC) equipped with a flame ionization detector (FID) and thermal conductivity detector (TCD) for gas analysis. The liquid product was weighed and sent to a gas chromatograph equipped with a mass spectrometer (GC-MS) to obtain benzene, toluene, and xylene (BTX). GC-MS35,36 utilized known concentrations of paraffins, isoparaffins, aromatics, naphthenes, and olefin compounds (PIANO) in a gasoline sample analysed by a calibrated GC-FID-PIONA according to ASTM method D5134 to quantify the components of the liquid product formulated from catalytic conversion. The hydrocarbon conversion, product selectivity and yields were calculated according to eqn (1)–(3) as shown below. |  | (1) |
|  | (2) |
| Product Yield wt% = Conversion C12 × Selectivity of product ÷ 100 | (3) |
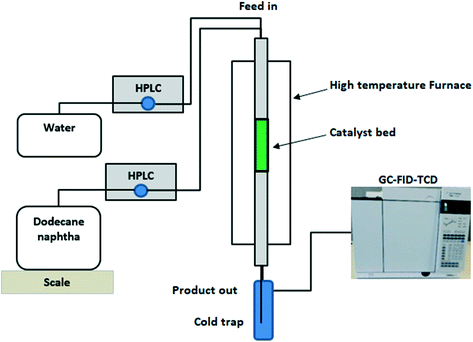 |
| Fig. 1 The catalytic packed bed reactor connected with GC-FID-TCD. | |
3. Results and discussion
3.1 Phase identification, morphology and textural properties
The TEM topography images are shown in Fig. 2[A] and [B] and the images are for 10% Zr–Ti and 50% Zr–Ti oxide catalysts. The particle size of the 10% Zr–Ti oxide catalyst was in the nanoscale particle size range, around 27 nm, which was slightly smaller than the 50% Zr–Ti oxide that was estimated to be around 30 nm, which indicates that more zirconia was dissolved with the titania framework. The practical shapes of both catalysts were rounded squares.
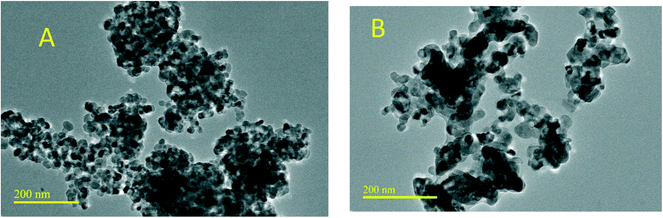 |
| Fig. 2 TEM image of catalysts: [A] 10% Zr–Ti oxide [B] 50% Zr–Ti oxide. | |
The XRD patterns for 10% and 50% Zr–Ti oxide catalysts obtained by X-ray diffraction analysis are shown in Fig. 3. TiO2 was used as a reference and the analysis illustrated that the catalyst was mainly made of the anatase crystalline phase. XRD patterns of the 10% Zr–Ti oxide catalyst showed smaller peaks of ZrO2 and the diffraction peaks detected at 28.5–30.5°, 50–51° and 60° are mainly for the tetragonal phase and a little of the monoclinic phase, with a high diffraction of anatase phase from titania. It was observed that increasing the loading of ZrO2 to 50% led to the peaks for the tetragonal phase of zirconia being more dominant than the other peaks of the monoclinic phase as seen in Fig. 3. The observed diffraction patterns of the synthesized Zr–Ti oxide catalysts are similar to those observed and described elsewhere.28,37,38 Although the synthesised Zr–Ti oxide catalysts have low textural properties, the surface area of the catalyst increased with the increasing weight of ZrO2 added to TiO2 as shown in Table 1. The pore volume of Zr–Ti oxide increased as more ZrO2 was incorporated into the TiO2 framework.39 The catalysts of 10% and 50% Zr–Ti oxide were regenerated and re-used, and the in situ decoking was conducted under air at 650 °C after each activity test and characterized the textural properties. Table S1† shows a decline in the surface area and pore volume of the regenerated catalyst as compared to the fresh catalyst. The crystallite diameters of Zr–Ti oxide catalysts estimated by using the Scherrer equation are presented in Table 1. The average diameter of TiO2 estimated at around 13 nm increased to 32 nm for the 10% Zr–Ti oxide catalyst, and 24 nm for the 50% Zr–Ti oxide catalyst, much like the crystallite size range observed by TEM.39
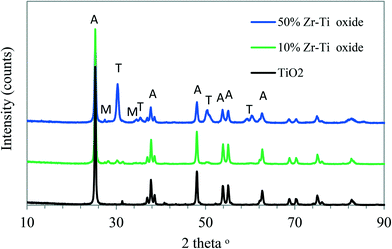 |
| Fig. 3 XRD diagram of Zr–Ti oxide catalysts synthesized by co-precipitation. (A): anatase phase, (T): tetragonal phase (M): monoclinic phase. | |
Table 1 The textural properties, NH3-TPD and H2 uptake of the synthesized Zr–Ti catalyst
Catalyst |
Surface area, m2 g−1 |
Pore volume cm3 g−1 |
Average pore diameter, Å |
H2 uptake cm3 g−1 STP |
NH3 adsorbed, μmol g−1 |
Crystallite size (nm) |
H2 uptake at the peak of Tmax 785 °C.
|
TiO2 |
11 |
0.10 |
38 |
1.4a |
45 |
13 |
10% Zr–Ti oxide |
15.7 |
0.15 |
39 |
0.08 |
215 |
32 |
50% Zr–Ti oxide |
48.7 |
0.33 |
27 |
0.31 |
395 |
24 |
3.2 Hydrogen reduction and surface acidity of the Zr–Ti oxide framework
Fig. 4 displays the H2-TPR profile for TiO2 used as a reference and the synthesized Zr–Ti oxide catalysts. Table 1 shows the low H2 consumption by the catalysts where ZrO2 was incorporated into the TiO2 framework, synthesized by co-participation as compared with the primary oxide of TiO2. This indicated the stable framework structure of Zr–O–Ti–O, similar to previous reports.39,40 The acidity of the synthesized catalyst of Zr–Ti oxide was characterized by using NH3-TPD. Fig. 5 shows a broader peak of NH3 desorption for the synthesized Zr–Ti oxide catalysts and the catalyst acidity was increased as more ZrO2 was incorporated into the TiO2 framework; similar observations were reported elsewhere.41Table 1 shows that the total acidity of catalysts increased for 10% Zr–Ti and 50% Zr–Ti oxides to 215 and 395 μmol g−1, respectively, as compared to the low acidity of the primary oxide of TiO2 in the range of 45 μmol g−1. Table S2 and Fig. S1† showed the pyridine-FTIR analysis of Zr–Ti oxide catalysts and Lewis acid sites increased with ZrO2 addition to TiO2 oxide, similar to findings by Manríquez et al.26 Overall, the catalyst characterization showed a stable framework of Zr–Ti oxide as pointed out by hydrogen reduction analysis, and the catalyst surface acidity was increased as more ZrO2 was incorporated into the TiO2 framework. The XRD analysis illustrated that the crystalline phases of the Zr–Ti oxide framework were mainly anatase and tetragonal phases. The crystallite diameter is associated with the surface acidity of combined metal oxide-based catalysts and the lower diameter crystal catalyst afforded more catalytic acidity as demonstrated in Table 1 and Fig. 5; a similar observation was made by Rao et al. (2010).39,42
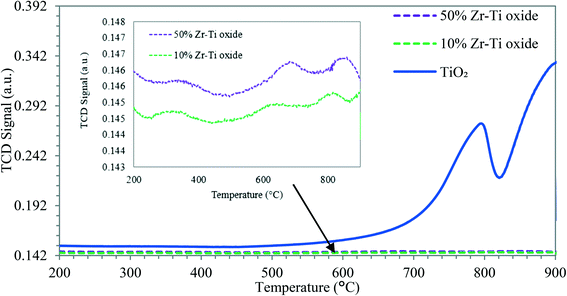 |
| Fig. 4 H2-TPR analysis of TiO2 (reference) and the Zr–Ti oxide synthesized by co-precipitation. | |
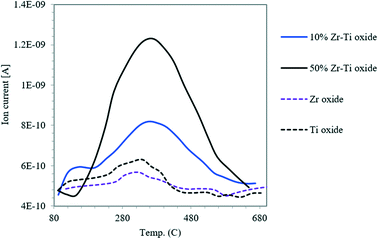 |
| Fig. 5 NH3-TPD analysis of Zr–Ti oxide catalysts. | |
3.3 Naphtha catalytic cracking reaction
3.3.1 Catalytic cracking (without steam).
In this study, dodecane catalytic cracking to olefins was investigated by using the Zr–Ti oxide catalyst. The catalytic selectivity was studied while changing the surface acidity by increasing the ZrO2 concentration in the catalyst.26 In order to evaluate the catalytic cracking performance, thermal cracking22 of dodecane was investigated and correlated with the catalysts used in this study. Fig. 6 shows that increasing the reaction temperature resulted in improved dodecane conversion. Thermal cracking delivered moderate conversion of dodecane and achieved 40–55% at reaction temperatures of 600 and 625 °C. The catalytic conversion of dodecane was increased by incorporating more ZrO2 into the TiO2 framework. The 50% Zr–Ti oxide catalyst delivered the highest conversion and achieved 86–95% at 600 and 625 °C, while lower conversion (70–77%) was achieved by the 10% Zr–Ti catalyst. Fig. 6 illustrates the gas yield obtained from the catalytic cracking of dodecane and it shows that the 50% Zr–Ti oxide catalyst produced a higher gas yield than the 10% Zr–Ti oxide catalyst. A higher gas yield could potentially mean that high catalytic activity involves ion abstraction of the hydrocarbon via carbenium ion and undertakes C–C cracking through the β-scission mechanism as exemplified by Fogassy et al. (2011),43 resulting in more light olefins.44
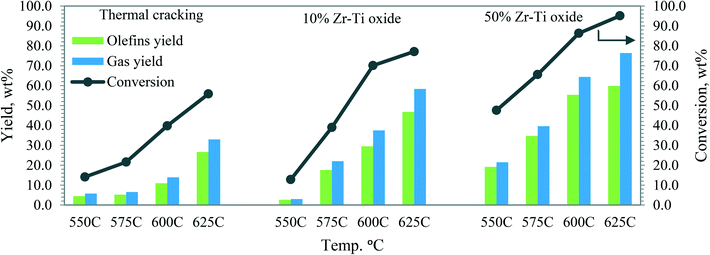 |
| Fig. 6 Dodecane conversion, olefin, and gas yields by using Zr–Ti oxide catalysts as compared with thermal cracking vs. reactor temperature, LHSV 0.5 h−1. | |
The olefins produced from catalytic cracking were studied and Fig. 6 shows that the lowest olefin yield was obtained through thermal cracking. The 50% Zr–Ti oxide showed the highest selectivity to olefin and achieved 55.4–59.8% yield at reactor temperatures of 600 and 625 °C, respectively, which is close to the reported olefin yield from renewable naphtha conversion by the steam cracker pilot-plant at 800 and 840 °C.45 Due to the lower acidity of 10% Zr–Ti oxide, the catalyst showed lower selectivity to olefins, delivering a yield in the range of 29.5–46.8% at 600 and 625 °C. The catalytic cracking of dodecane to olefin with selectivity for propylene and ethylene was affected by the catalyst composition and reaction temperature (Fig. S2†).
Olefins obtained from catalytic cracking as well as thermal cracking of dodecane were investigated. It was observed that the lowest total olefins yield was delivered by thermal cracking as seen in Fig. 6. Increasing the temperature from 550 to 625 °C led to producing more ethylene (12%) than propylene and butenes as demonstrated in Fig. 7. The catalytic cracking via 10% Zr–Ti oxide catalyst showed an increase in the olefin yield and ethylene was higher than propylene and butenes. The achieved yields were higher than those attained by thermal cracking as shown in Fig. 7. At 600 and 625 °C, the ethylene yields were 9% and 16%, respectively, a slightly higher yield than the propylene yield at 10% and 16%, respectively. On the other hand, the 50% Zr–Ti oxide catalyst (Fig. 7) delivered the highest yields of olefin due to the higher and broader peak of surface acidity than 10% Zr–Ti oxide, which resulted in higher conversion. The ethylene yield was increased with increased reactor temperature. At 600 °C, around 8.3% ethylene was produced while propylene and butene yields were 24% and 23%, respectively. Increasing the catalytic cracking temperature to 625 °C resulted in a decline in the propylene (23%) and butene (19%) yield. The ethylene yield increased to 17.5% and indicated further carbenium ion cracking44 of co-products from dodecane to yield more ethylene.
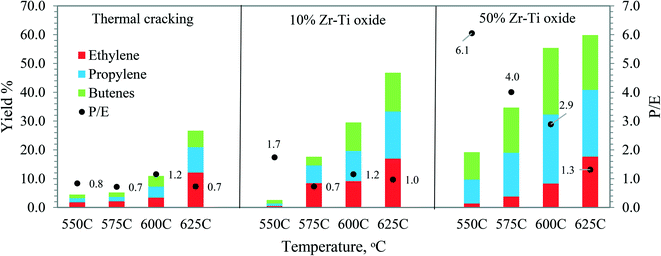 |
| Fig. 7 Light olefin yield (ethylene, propylene and butenes) and P/E ratio by using Zr–Ti oxide catalysts as compared with thermal cracking vs. reactor temperature, LHSV 0.5 h−1. | |
Fig. 7 shows the propylene/ethylene (P/E) ratio where it was low at 0.7–0.8 and 1.2 in the case of thermal cracking, suggesting that more ethylene was produced to facilitate more free radical cracking reactions of dodecane.46 The catalyst selectivity of 10% Zr–Ti oxide was different from 50% Zr–Ti oxide and the P/E ratio obtained was in the range of 0.7, 1.0, 1.2, and 1.7, which is a little higher than thermal cracking. Although the 50% Zr–Ti oxide showed the highest yield of olefins, the P/E ratio of 6.1 was achieved at 550 °C and indicated greater favorability for carbenium ions through the β-scission mechanism via catalyst, whereas it decreased to 1.3 on increasing the temperature, suggesting that more ethylene was produced at higher cracking temperatures. Severe catalytic cracking of Fischer–Tropsch produced synthetic oil of naphtha feedstock using a mixture of Y-zeolite with ZSM-5 catalyst was reported with a P/E ratio of 1.6 by Dement'ev et al. (2020).47
The study was extended to analyze hydrocarbon naphtha products collected from the catalytic cracking of dodecane at 625 °C by using GC-MS. Fig. 8 shows the converted hydrocarbon products over the catalysts and thermal cracking. The results showed that Zr–Ti oxide promoted cyclization reactions and produced aromatics from dodecane conversion. Higher aromatics and BTX yields were obtained over the 50% Zr–Ti oxide catalyst, which were 15.7 and 4%, respectively, indicating that the Lewis acid sites26,48 of the catalyst promoted the cyclization reaction of intermediates and underwent carbenium ion mechanisms over the Zr–Ti framework as described by Liu et al.,48 Fung et al.,29 and Sanchez-Castillo et al.30 Due to the lower acidity of the 10% Zr–Ti oxide, fewer cyclization reactions occurred in the catalyst pores resulting in low yield of aromatics (6.5%) and BTX (1.4%). Thermal cracking experiments showed only a trace of aromatics of around 0.1% from the cyclization reaction.
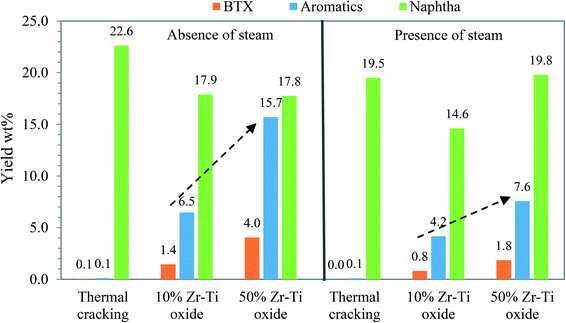 |
| Fig. 8 Steam vs. no steam for BTX and aromatic yields by using Zr–Ti oxide catalysts as compared with thermal cracking (catalytic cracking temperature 625 °C, LHSV 0.5 h−1). | |
3.3.2 Comparison of the steam and no steam catalytic cracking of dodecane.
The cracking of dodecane was further investigated in the presence of steam. The obtained products over Zr–Ti oxide were compared with thermal steam cracking at 625 °C. Fig. 9 shows that steam had almost no effect on thermal cracking and a similar conversion to that without steam was achieved. However, in the presence of steam, the olefins yield was slightly increased from 26% to 28% (Fig. 10) and there was a small increase in the propylene and ethylene selectivity (Fig. S3†). In the case of catalytic cracking, the 10% Zr–Ti oxide catalyst showed a small improvement in conversion under steam and increased from 77% to 80%. Also, the olefins yield increased from 46% to 53% (Fig. 10), similar to the olefin range of the steam cracker unit without catalyst (850 °C) for renewable naphtha reported by Pyl et al. (2011).45 The small increase in olefin due to the 10% Zr–Ti oxide catalyst promoted more carbenium ions and β-scission mechanisms over the catalyst and was less favorable for the cyclization reaction (Fig. 8). However, the addition of steam had adverse effects during dodecane cracking over the 50% Zr–Ti oxide catalyst and the conversion was decreased from 95% to 85%. This could be attributed to the lowered catalyst acidity of 50% Zr–Ti oxide in the presence of steam; the Lewis acidic sites were probably affected and several studies suggested that steam would contribute a negative effect by blocking some active acidic sites of catalysts.49–51 In addition, the cyclization reaction to formulate aromatics was decreased from 15% to 7% as presented in Fig. 8. Fig. 10 shows the olefin component produced from steam catalytic cracking and the yields of ethylene, propylene, and butene were slightly decreased but had an insignificant effect on the P/E ratio.
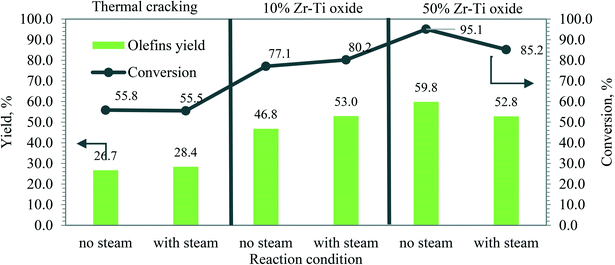 |
| Fig. 9 Steam vs. no steam catalytic cracking of dodecane, and olefin yield (cracking temperature 625 °C, LHSV 0.5 h−1). | |
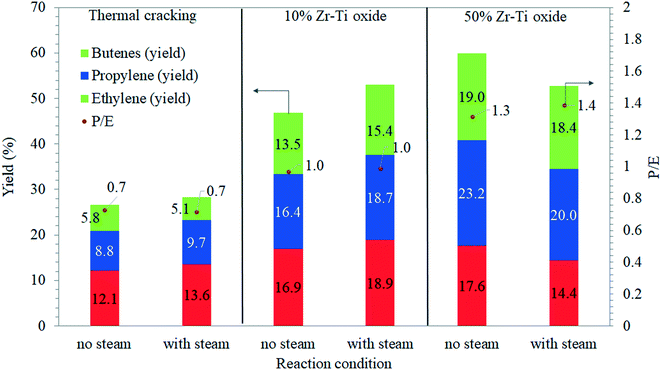 |
| Fig. 10 Light olefin yield and P/E ratio of steam vs. no steam catalytic cracking (cracking temperature 625 °C, LHSV 0.5 h−1). | |
3.3.3 Catalytic cracking stability.
The study investigated the catalytic cracking stability over time-on-stream (TOS) to determine the catalysts' framework steadiness during high-temperature cracking and conversion to olefins. The 10% and 50% Zr–Ti oxide catalysts were demonstrated to be robust catalytic materials and the catalysts had been regenerated under air flow at 600–625 °C for 2 hours and used for TOS experiment. The catalysts were then utilized to investigate catalytic cracking stability. Fig. 11 shows dodecane conversion over the 50% Zr–Ti oxide catalyst for 7 hours of time-on-stream. The olefin yield started at around 62–66% and then declined to 52–55%. This is similar to the olefin yield produced from Fischer–Tropsch synthetic oil reported by Dement'ev et al. (2020),47 however, that Fischer–Tropsch synthetic oil conversion was conducted under severe catalytic cracking at 675 °C over a catalyst mixture of Y-zeolite with ZSM-5. On the other hand, the olefins yield over the 10% Zr–Ti oxide catalyst was almost stable, starting at 44–45%, and only dropped by 2% to 42–43% after 7 hours on stream. Furthermore, a decline in the yield of propylene from 28% to 20% was observed over the 50% Zr–Ti oxide catalyst. Ethylene and butene yields were maintained as demonstrated in Fig. 11. Moreover, it can be observed from Fig. 11 that the yield of olefins was almost stable over the 10% Zr–Ti oxide for 7 hours with only a slight decrease in the ethylene yield from ∼20% to ∼18%. The time-on-stream studies showed that Zr–Ti oxide catalysts are robust catalysts for naphtha catalytic cracking.
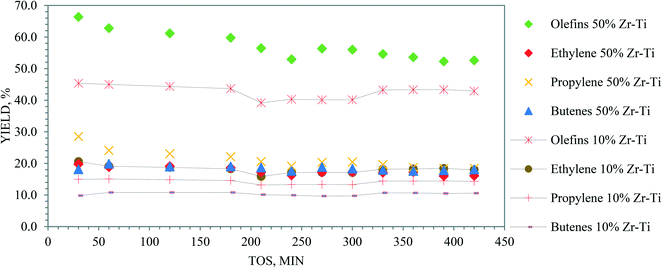 |
| Fig. 11 Time-on-stream and yields of olefin, ethylene, propylene, and butene for 50% Zr–Ti oxide and 10% Zr–Ti oxide (catalytic cracking temperature 625 °C, LHSV 0.5 h−1). | |
In summary, low conversions in the absence of catalyst were obtained during the thermal and steam cracking of dodecane, while the catalytic cracking of dodecane over the synthesized catalyst by co-participation improved the conversion and olefin yield. It was revealed that the Zr–Ti oxide catalyst has a stable framework from hydrogen reduction39,40 and its catalytic acidity was sufficient to promote the cracking of naphtha through the β-scission mechanism43 and achieved more light olefins.44Fig. 6 and S2† show that the higher gas yield could potentially mean high catalytic activity of both 10% Zr–Ti and 50% Zr–Ti oxide catalysts, and involves the ion abstraction of hydrocarbon via carbenium ions and undertakes C–C cracking through the β-scission mechanism43 and lead to more light olefins being obtained.44 Fig. S6† shows the schematic diagram of dodecane cracking and the carbenium ion contribution via the catalytic cracking mechanism. The high yield of light olefins (ethylene, propylene, and butene) and other gas products indicated an active β-scission mechanism and is a more favored reaction as compared to the free radical mechanism of the thermal cracking of dodecane at a similar conversion temperature. Fig. 8 and S3† show the aromatic selectivity produced from Zr–Ti oxide and the cyclization reaction progressed over the catalyst and increased as the Lewis acid sites increased in the catalysts.26,29,30 The P/E ratio was altered by increasing the catalytic acidity and reaction temperature. However, the ratio was not much affected by the addition of steam to the reaction. The catalytic stability is an important factor in selecting the catalyst material to achieve higher olefin yields. The catalysts synthesized by adding ZrO2 to the TiO2 framework were demonstrated to be highly stable surface oxides catalysts39 under harsh reaction conditions.
4. Conclusions
Zr–Ti oxide nanocatalysts showed a unique catalyst cluster for assisting in hydrocarbon cracking to produce olefins and promote cyclization reactions to synthesize BTX. Increasing the incorporated amount of ZrO2 in the TiO2 farmworker led to an increase in the catalyst surface acidity and catalyst stability observed from hydrogen reduction. It was found during this study that the catalyst reactivity and P/E ratio were affected by the loaded amount of ZrO2 to the TiO2 framework and reaction temperature. Increasing the reaction temperature resulted in an increase in the ethylene yield due to the further cracking of primary products and by-products, especially over 50% Zr–Ti oxide. Performing the catalytic cracking in the presence of steam led to only a slight increase in dodecane conversion over 10% Zr–Ti oxide and did not affect the P/E ratio. Zr–Ti oxide was shown to be a promising catalyst for naphtha conversion to olefins and the catalysts showed high stability with time-on-stream.
Conflicts of interest
There are no conflicts to declare.
Acknowledgements
The authors would like to acknowledge the effort of Dr. H. Sitepu from R&DC for XRD analysis on various catalyst samples.
References
- M. Umar, X. Ji, D. Kirikkaleli and A. A. Alola, The imperativeness of environmental quality in the United States transportation sector amidst biomass-fossil energy consumption and growth, J. Cleaner Prod., 2021, 285, 124863 CrossRef CAS , Available from: https://www.sciencedirect.com/science/article/pii/S0959652620349076.
- S. Standl, F. M. Kirchberger, T. Kühlewind, M. Tonigold, M. Sanchez-Sanchez and J. A. Lercher,
et al. Single-event kinetic model for methanol-to-olefins (MTO) over ZSM-5: Fundamental kinetics for the olefin co-feed reactivity, Chem. Eng. J., 2020, 402, 126023 CrossRef CAS.
- I. Amghizar, L. A. Vandewalle, K. M. Van Geem and G. B. Marin, New Trends in Olefin Production, Engineering, 2017, 3(2), 171–178 CrossRef CAS , Available from: https://www.sciencedirect.com/science/article/pii/S2095809917302965.
- R. Palos, A. Gutiérrez, M. L. Fernández, M. J. Azkoiti, J. Bilbao and J. M. Arandes, Converting the Surplus of Low-Quality Naphtha into More Valuable Products by Feeding It to a Fluid Catalytic Cracking Unit, Ind. Eng. Chem. Res., 2020, 59(38), 16868–16875 CrossRef CAS.
- Y. Yoshimura, N. Kijima, T. Hayakawa, K. Murata, K. Suzuki and F. Mizukami,
et al. Catalytic Cracking of Naphtha to Light Olefins, Catal. Surv. Jpn., 2001, 4(2), 157–167 CrossRef , Available from: https://doi.org/10.1023/A:1011463606189.
- G. A. Nasser, M. H. M. Ahmed, M. A. Firdaus, M. A. Sanhoob, I. A. Bakare and E. N. Al-Shafei,
et al., Nano BEA zeolite catalysts for the selective catalytic cracking of n-dodecane to light olefins, RSC Adv., 2021, 11(14), 7904–7912 RSC.
- P. Wattanapaphawong, P. Reubroycharoen, N. Mimura, O. Sato and A. Yamaguchi, Effect of carbon number on the production of propylene and ethylene by catalytic cracking of straight-chain alkanes over phosphorus-modified ZSM-5, Fuel Process. Technol., 2020, 202, 106367 CrossRef CAS , Available from: https://www.sciencedirect.com/science/article/pii/S0378382019321630.
- A. K. Jamil, O. Muraza, K. Miyake, M. H. M. Ahmed, Z. H. Yamani and Y. Hirota,
et al. Stable production of gasoline-ranged hydrocarbons from dimethyl ether over iron-modified ZSM-22 zeolite, Energy Fuels, 2018, 32(11), 11796–11801 CrossRef CAS.
- M. A. Sanhoob, O. Muraza, M. Yoshioka, M. Qamaruddin and T. Yokoi, Lanthanum, cerium, and boron incorporated ZSM-12 zeolites for catalytic cracking of n-hexane, J. Anal. Appl. Pyrolysis, 2018, 129, 231–240 CrossRef CAS.
- I. A. Bakare, O. Muraza, T. Kurniawan, Z. H. Yamani, E. N. Shafei and A. K. Punetha,
et al. Hydrothermal stability of MTT zeolite in hot water: The role of La and Ce, Microporous Mesoporous Mater., 2016, 233 Search PubMed.
- S. A. Lateef, I. A. Bakare, A. Mayoral, V. Sebastian and O. Muraza, Selective catalytic cracking of n-hexane to olefins over SSZ-54 fabricated by facile and novel dual templating method, Fuel, 2018, 227, 48–58 CrossRef CAS.
- M. Albahar, C. Li, V. L. Zholobenko and A. A. Garforth, The effect of ZSM-5 zeolite crystal size on p-xylene selectivity in toluene disproportionation, Microporous Mesoporous Mater., 2020, 302, 110221 CrossRef CAS.
- H.-J. Freund and M. W. Roberts, Surface chemistry of carbon dioxide, Surf. Sci. Rep., 1996, 25(8), 225–273 CrossRef.
- A. Corma, J. Mengual and P. J. Miguel, Steam catalytic cracking of naphtha over ZSM-5 zeolite for production of propene and ethene: Micro and macroscopic implications of the presence of steam, Appl. Catal., A, 2012, 417, 220–235 CrossRef.
- A. Yamaguchi, D. Jin, T. Ikeda, K. Sato, N. Hiyoshi and T. Hanaoka,
et al. P-ZSM-5 pretreated by high-temperature calcination as durable catalysts for steam cracking of n-hexane, Catal. Lett., 2014, 144(1), 44–49 CrossRef CAS.
- A. Yamaguchi, D. Jin, T. Ikeda, K. Sato, N. Hiyoshi and T. Hanaoka,
et al. Effect of steam during catalytic cracking of n-hexane using P-ZSM-5 catalyst, Catal. Commun., 2015, 69, 20–24 CrossRef CAS.
- H. K. Amusa, S. Adamu, I. A. Bakare, A. S. Arjah, S. A. Al-Bogami and S. Al-Ghamdi,
et al. High-performance VOx on SrO-$γ$Al2O3 catalyst for oxidative cracking of n-hexane to light olefins under anaerobic environment, J. Ind. Eng. Chem., 2020, 89, 339–350 CrossRef CAS.
- C. Boyadjian, B. van der Veer, I. V. Babich, L. Lefferts and K. Seshan, Catalytic oxidative cracking as a route to olefins: Oxidative conversion of hexane over MoO3-Li/MgO, Catal. Today, 2010, 157(1–4), 345–350 CrossRef CAS.
- C. Boyadjian and L. Lefferts, Promoting Li/MgO catalyst with molybdenum oxide for oxidative conversion of n-hexane, Catalysts, 2020, 10(3), 354 CrossRef CAS.
- R. B. Dudek, X. Tian, M. Blivin, L. M. Neal, H. Zhao and F. Li, Perovskite oxides for redox oxidative cracking of n-hexane under a cyclic redox scheme, Appl. Catal., B, 2019, 246, 30–40 CrossRef CAS.
- X. Tian, R. B. Dudek, Y. Gao, H. Zhao and F. Li, Redox oxidative cracking of n-hexane with Fe-substituted barium hexaaluminates as redox catalysts, Catal. Sci. Technol., 2019, 9(9), 2211–2220 RSC.
- Y. Jiao, H. Zhang, S. Li, C. Guo, P. Yao and J. Wang, Impact of acidity in ZrO2-TiO2-Al2O3 composite oxides on the catalytic activity and coking behaviors during n-decane cracking, Fuel, 2018, 233, 724–731 CrossRef CAS.
- L. O. Alemán-Vázquez, J. L. Cano-Domínguez, E. Torres-García and J. R. Villagómez-Ibarra, Industrial aplication of catalytic systems for n-heptane isomerization, Molecules, 2011, 16(7), 5916–5927 CrossRef.
- C.-C. Hwang and C.-Y. Mou, Comparison of the promotion effects on sulfated mesoporous zirconia catalysts achieved by alumina and gallium, Appl. Catal., A, 2009, 365(2), 173–179 CrossRef CAS.
- I. Wang, W.-F. Chang, R.-J. Shiau, J.-C. Wu and C.-S. Chung, Nonoxidative dehydrogenation of ethylbenzene over TiO2 ZrO2 catalysts: I. Effect of composition on surface properties and catalytic activities, J. Catal., 1983, 83(2), 428–436 CrossRef CAS.
- M. E. Manríquez, T. López, R. Gómez and J. Navarrete, Preparation of TiO2-ZrO2 mixed oxides with controlled acid-basic properties, J. Mol. Catal. A: Chem., 2004, 220(2), 229–237 CrossRef.
- H.-Y. T. Chen, S. Tosoni and G. Pacchioni, A DFT study of the acid--base properties of anatase TiO2 and tetragonal ZrO2 by adsorption of CO and CO2 probe molecules, Surf. Sci., 2016, 652, 163–171 CrossRef CAS.
- V. Vishwanathan, H.-S. Roh, J.-W. Kim and K.-W. Jun, Surface properties and catalytic activity of TiO 2--ZrO 2 mixed oxides in dehydration of methanol to dimethyl ether, Catal. Lett., 2004, 96(1–2), 23–28 CrossRef CAS.
- J. Fung and I. Wang, The Reaction Mechanism of C6Hydrocarbons over Acid–Base Bifunctional Catalysts, TiO2–ZrO2, J. Catal., 1996, 164(1), 166–172 CrossRef CAS , Available from: https://www.sciencedirect.com/science/article/pii/S0021951796903723.
- M. A. Sanchez-Castillo, R. J. Madon and J. A. Dumesic, Role of Rare Earth Cations in Y Zeolite for Hydrocarbon Cracking, J. Phys. Chem. B, 2005, 109(6), 2164–2175, DOI:10.1021/jp0489875.
- D. Mao, G. Lu and Q. Chen, Influence of calcination temperature and preparation method of TiO2--ZrO2 on conversion of cyclohexanone oxime to $\varepsilon$-caprolactam over B2O3/TiO2--ZrO2 catalyst, Appl. Catal., A, 2004, 263(1), 83–89 CrossRef CAS.
- M. H. M. Ahmed, O. Muraza, A. Galadima, A. K. Jamil, E. N. Shafei and Z. H. Yamani,
et al. Hydrothermal Stabilization of Rich Al-BEA Zeolite by Post-Synthesis
Addition of Zr for Steam Catalytic Cracking of n -Dodecane, Energy Fuels, 2018, 32(4), 5501–5508 CrossRef CAS.
- M. A. Sanhoob, U. Khalil, E. N. Shafei, K.-H. Choi, T. Yokoi and O. Muraza, Steam cracking of green diesel (C12) to BTX and olefins over silane-treated hierarchical BEA, Fuel, 2020, 263, 116624 CrossRef CAS , Available from: https://www.sciencedirect.com/science/article/pii/S0016236119319787.
- A. Kostyniuk, D. Bajec and B. Likozar, Catalytic hydrocracking reactions of tetralin as aromatic biomass tar model compound to benzene/toluene/xylenes (BTX) over zeolites under ambient pressure conditions, J. Ind. Eng. Chem., 2021, 96, 130–143 CrossRef CAS.
-
S. A. Stout, G. S. Douglas and A. D. Uhler, Chemical fingerprinting of gasoline and distillate fuels, in Standard Handbook Oil Spill Environmental Forensics, Elsevier, 2016, pp. 509–564 Search PubMed.
- W. Xiong, R. Bernesky, R. Bechard, G. Michaud and J. Lang, A tiered approach to distinguish sources of gasoline and diesel spills, Sci. Total Environ., 2014, 487, 452–462 CrossRef CAS PubMed.
- E. N. Al-Shafei, D. Robert Brown, S. P. Katikaneni, H. Aljama and H. A.-H. Badairy, Direct conversion of CO2 with methane into chemicals over ZrO2/TiO2 catalysts, Chem. Eng. J., 2021, 129416 CrossRef CAS , Available from: https://www.sciencedirect.com/science/article/pii/S1385894721010044.
- B. Neppolian, Q. Wang, H. Yamashita, H. Choi, V. Vishwanathan and H.-S. Roh,
et al. Synthesis and characterization of ZrO2--TiO2 binary oxide semiconductor nanoparticles: application and interparticle electron transfer process, Appl. Catal., A, 2006, 96(1), 23–28 Search PubMed , Available from: https://www.sciencedirect.com/science/article/pii/S0926860X03001285.
- K. N. Rao, B. M. Reddy and S.-E. Park, Novel CeO2 promoted TiO2--ZrO2 nano-oxide catalysts for oxidative dehydrogenation of p-diethylbenzene utilizing CO2 as soft oxidant, Appl. Catal., B, 2010, 100(3–4), 472–480 CrossRef CAS.
- G. Rajua, B. M. Reddy and S. E. Parkb, Utilization of carbon dioxide in oxidative dehydrogenation reactions, Indian J. Chem., Sect. A: Inorg., Bio-inorg., Phys., Theor. Anal. Chem., 2012, 51(9–10), 1315–1324 Search PubMed.
- R. Pérez-Hernández, D. Mendoza-Anaya, M. E. Fernández and A. Gómez-Cortés, Synthesis of mixed ZrO2--TiO2 oxides by sol--gel: microstructural characterization and infrared spectroscopy studies of NOx, J. Mol. Catal. A: Chem., 2008, 281(1–2), 200–206 CrossRef.
- K. Nishiwaki, N. Kakuta, A. Ueno and H. Nakabayashi, Generation of acid sites on finely divided TiO2, J. Catal., 1989, 118(2), 498–501 CrossRef CAS , Available from: https://www.sciencedirect.com/science/article/pii/0021951789903370.
- G. Fogassy, N. Thegarid, Y. Schuurman and C. Mirodatos, From biomass to bio-gasoline by FCC co-processing: effect of feed composition and catalyst structure on product quality, Energy Environ. Sci., 2011, 4(12), 5068–5076 RSC.
- K. H. Song, S. K. Jeong, B. H. Jeong, K.-Y. Lee and H. J. Kim, Acid/base-treated activated carbon catalysts for the low-temperature endothermic cracking of n-dodecane with applications in hypersonic vehicle heat management systems, Catalysts, 2020, 10(10), 1149 CrossRef CAS.
- S. P. Pyl, C. M. Schietekat, M.-F. Reyniers, R. Abhari, G. B. Marin and K. M. Van Geem, Biomass to olefins: Cracking of renewable naphtha, Chem. Eng. J., 2011, 176, 178–187 CrossRef.
- T. Ren, M. Patel and K. Blok, Olefins from conventional and heavy feedstocks: Energy use in steam cracking and alternative processes, Energy, 2006, 31(4), 425–451 CrossRef CAS.
- K. I. Dement'ev, A. D. Sagaradze, P. S. Kuznetsov, T. A. Palankoev and A. L. Maximov, Selective Production of Light Olefins from Fischer--Tropsch Synthetic Oil by Catalytic Cracking, Ind. Eng. Chem. Res., 2020, 59(36), 15875–15883 CrossRef.
- J. Liu, N. He, W. Zhou, L. Lin, G. Liu and C. Liu,
et al. Isobutane aromatization over a complete Lewis acid Zn/HZSM-5 zeolite catalyst: performance and mechanism, Catal. Sci. Technol., 2018, 8(16), 4018–4029 RSC.
- F. Raoof, M. Taghizadeh, A. Eliassi and F. Yaripour, Effects of temperature and feed composition on catalytic dehydration of methanol to dimethyl ether over $γ$-alumina, Fuel, 2008, 87(13–14), 2967–2971 CrossRef CAS.
- K.-W. Jun, H.-S. Lee, H.-S. Roh and S.-E. Park, Highly water-enhanced H-ZSM-5 catalysts for dehydration of methanol to dimethyl ether, Bull. Korean Chem. Soc., 2003, 24(1), 106–108 CrossRef CAS.
- B. Sabour, M. H. Peyrovi, T. Hamoule and M. Rashidzadeh, Catalytic dehydration of methanol to dimethyl ether (DME) over Al-HMS catalysts, J. Ind. Eng. Chem., 2014, 20(1), 222–227 CrossRef CAS.
Footnote |
† Electronic supplementary information (ESI) available. See DOI: 10.1039/d1re00290b |
|
This journal is © The Royal Society of Chemistry 2022 |