DOI:
10.1039/D2RA07568G
(Paper)
RSC Adv., 2022,
12, 35270-35278
Solvent-polarity reconfigurable fluorescent 4-piperazino-N-aryl-1,8-naphthalimide crown ether logic gates†
Received
28th November 2022
, Accepted 30th November 2022
First published on 9th December 2022
Abstract
Four compounds 1–4 were designed and synthesised, comprising a 4-amino-N-aryl-1,8-naphthalimide fluorophore, a piperazine receptor, and an aryl group, as fluorescent logic gates. At the imide position, the substituent is phenyl (1), 1,2-dimethoxyphenyl (2), benzo-15-crown-5 (3), or benzo-18-crown-6 (4). Molecules 1 and 2 are constructed according to a fluorophore–spacer–receptor format, while 3 and 4 are engineered according to a receptor1–spacer1–fluorophore–spacer2–receptor2 format based on photoinduced electron transfer and internal charge transfer mechanisms. The compounds were studied in water, water/methanol mixtures of different ratios, and methanol by UV-visible absorption and steady-state fluorescence spectroscopy, as a function of pH, metal ions and solvent polarity. The excited state
of 1–4 is 8.4 ± 0.2 in water, 7.6 ± 0.1 in 1
:
1 (v/v) water/methanol, and 7.1 ± 0.3 in methanol. The
of 3 in water is 0.92 and the
and
of 4 in water are 2.3 and 2.9. 1H NMR data in D2O and CD3OD confirm H+ interaction at the piperazine moiety, and Na+ and Ba2+ binding at the benzo-15-crown-5 and benzo-18-crown-6 moieties of 3 and 4. By altering the solvent polarity, the fluorescent logic gates can be reconfigured between TRANSFER logic and AND logic. Molecules with polarity reconfigurable logic could be useful tools for probing the microenvironment of cellular membranes and protein interfaces.
Introduction
The field of molecular logic-based computation1 has attracted a considerable amount of attention since the inception of the first molecular AND logic gate in 1993 (ref. 2) based on photoinduced electron transfer (PET).3 From these humble beginnings, the field has steadily moved forward from proof-of-concept demonstrations to potential societal applications.4 Examples of fluorescent logic gates along these lines of exploration include multi-analyte AND logic gates (lab-on-a-molecules),5 corrosion (Pourbaix) sensors,6 medical diagnostics,7 phototherapeutic releasing molecules,8 tagging of micrometer objects,9 cellular/enzyme monitoring10 and edge detection.11
The first molecular AND logic gates by de Silva were studied in alcohol solutions of methanol and propanol.2,12 Methanol is the de facto solvent substitute for water when solubility is an issue, while ethanol is a less toxic alternative for cellular studies.13 In many studies, a compromise is to use water/alcohol solvent compositions.14 Given this tendency, it is often assumed that the alcohol/water ratio readily correlates to the fluorescence quantum yield (ΦF). However, to the best of our knowledge, there are no systematic studies that examine the effect of solvent polarity, specifically in water/methanol, on the photophysical parameters of crown ether sensors (i.e. ΦF).15 In biological systems, notably at the cellular level, polarity determines interactions with proteins and enzymes, and the permeability of membrane compartments.16 Evidence suggests that mitochondrial16 and lysosomal17 polarity in cancer cells is lower than in normal cells. And so a better understanding of polarity effects is medicinally useful.
Crown ether-based chemosensors, although dating over three decades, still attract attention.18–20 Recently, there is considerable interest in PET chemosensors for Ba2+.21 Physicists are searching for evidence that neutrinos are their own antiparticles by investigating neutrinoless double beta decay of 136Xe to 136Ba2+.22 Using single molecule fluorescent imaging (SMFI), experimentalists are searching for Ba2+ in situ and in real time from the decay of 136Xe in high pressure xenon gas under ultra-vacuum conditions. Thapa and co-workers have reported diazacrown 4-amino-1,8-naphthalimide chemosensors for Ba2+ in acetonitrile and in dry phase.23 In this study, as with many azacrown chemosensor studies, acetonitrile is used as the solvent. There is a tendency for trace water and/or hydrated metal ions to act as unsuspecting proton sources.24 Hence, the emission enhancement may not always be due to metal ion complexation, but rather to protonation of a nitrogen atom. A remedy to this issue is to use oxacrown ethers.25
In our previous study, we investigated the properties of 4-methylpiperazino-N-aryl-1,8-naphthalimides.26 We discovered that a carbonyl moiety of the 4-piperazino-1,8-naphthalimide fluorophore assists in metal ion binding (i.e. Na+, K+) within the benzo-15-crown-5 and benzo-18-crown-6 cavities in the excited state. Building upon this finding, we have investigated 4-piperazino-N-aryl-1,8-naphthalimides with benzo-15-crown-5 and benzo-18-crown-6 moieties as solvent-polarity reconfigurable logic gates. We selected piperazine as the proton receptor, which is readily protonated in neutral water, to facilitate 1H NMR titration experiments in D2O. An objective was to examine whether there is any ground state interaction between a carbonyl of the naphthalimide and the metal ion within the crown cavity.26 We have also systematically and thoroughly examined the effect of water/methanol solvent compositions in 10% (v/v) increments on the photophysical properties of four 4-amino-N-aryl-1,8-naphthalimide logic gates. Molecules 3 and 4 are designed with a receptor1–spacer1–fluorophore–spacer2–receptor2 format12 based on PET3 and internal charge transfer (ICT) with a 1,8-naphthalimide fluorophore, a piperazine moiety at the 4-position and a benzocrown ether at the imide position. Spacer1 is the N-imide node, and spacer2 is the diethylene bridges within the piperazine moiety consistent with C0-type and C2-type spacers.27 Molecules 1 and 2 with phenyl and 1,2-dimethoxyphenyl moieties serve as fluorophore–spacer–receptor models (Scheme 1).
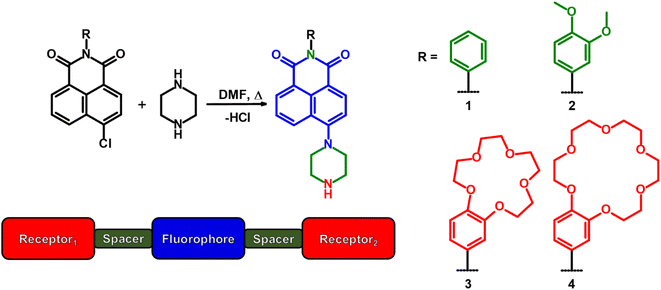 |
| Scheme 1 The synthesis of 4-piperazino-N-aryl-1,8-naphthalimide molecular logic gates 1–4 and the design format of 3 and 4. | |
Results and discussion
The syntheses of 1–4 are shown in Scheme 1. 4-Chloro-1,8-naphthalic anhydride was reacted with aniline, 3,4-dimethoxyaniline, 4′-aminobenzo-15-crown-5 or 4′-aminobenzo-18-crown-6.16 The 4-chloro-N-aryl-1,8-naphthalimides were then reacted with excess piperazine in N,N-dimethylformamide (DMF) at 130 °C. The products were recovered as orange powders in good yields ranging from 77–94% after precipitation from a suitable solvent. The compounds were characterised by standard spectroscopic techniques including 1H, 13C, and 13C DEPT-135 NMR, FTIR, and HRMS. Full details are provided in the Experimental section. The corresponding spectra are available in the ESI (Fig. S1–S16†).
The thermodynamic driving forces for PET, based on the Weller equation (eqn (1)) from the piperazine secondary amine and the 3,4-dimethoxyphenyl to the excited state amino-1,8-naphthalimide fluorophore, are 0.07 eV and 0.37 eV (6.7 kJ mol−1 and 35 kJ mol−1).28
|
ΔGPET = Eox + Ered − ES − e2/εr
| (1) |
These estimates are based on known electrochemical data.12,28 Although the values are mildly endothermic, they are influenced by the solvent polarity according to the coulombic term e2/εr, where ε is the solvent dielectric constant. Intramolecular PET in neutral molecules generates a charged radical ion pair, which is stabilised by solvents with dipole moments. Generally, the greater the solvent polarity, the more stabilised the radical ion pair resulting from PET, and so the ΦF falls concurrently.25,26 As part of this study, we have systematically examined the effect of the ε (methanol: 33, water: 80) on the competition between PET and fluorescence as a continuum between water to methanol in 10% (v/v) methanol increments.
The UV-visible absorption spectra of 1–4 were first examined as a function of acidity in water, 1
:
1 (v/v) water/methanol, and methanol (Fig. S17–S20).† A summary of data for 1–4 is available in Table S1† and specifically for 4 in Table 1. Compounds 1 and 2 have λmax at 405 nm and 403 nm in water. In 10−4 M H+, slight hypsochromic shifts of 8 nm and 2 nm are observed. The log
ε of 1 and 2 are 3.18 ± 0.05 and 3.54 ± 0.04 and are indicative of π → π* transitions. In methanol, the λmax is 393 nm for 1 and 2. Overall, there is little change in the UV-vis spectra of 1 and 2, with the exception of 1 in water with an isosbestic point at 345 nm. In 1
:
1 (v/v) water/methanol and methanol, the log
ε for 1–4 are relatively constant at 3.98 ± 0.11. The spectra of 3 are generally consistent with H+-induced peak shifts of 14 nm, 10 nm and 6 nm in water, 1
:
1 (v/v) water/methanol and methanol, respectively, with isosbestic points at 416 nm, 409 nm and 402 nm. In contrast, the spectra of 4 are notable. Titrations of 4 from 10−11 M to 10−4 M H+ reveals bathochromic shifts of 14 nm, 24 nm and 21 nm accompanied by hyperchromic shifts with a λmax at 392 nm, 389 nm and 380 nm in water, 1
:
1 (v/v) water/methanol and methanol, respectively. Isosbestic points appear at 416 nm, 409 nm and 402 nm. Isosbestic points are distinctly observed in all three composition solvents for 3 and 4 (and for 1 in water).
Table 1 Spectroscopic parameters of 4 in water, 1
:
1 (v/v) water/methanol and methanol.a See Table S1 in the ESI for 1–3
Parameter |
H2O (ϕH2O = 1.0) |
H2O/MeOH (ϕH2O = 0.5) |
MeOH (ϕH2O = 0.0) |
10−4 M. ε in L mol−1 cm−1. λex = 400 nm. Δλ = λem − λabs. Δv = 1/λabs − 1/λem. Measured with reference to quinine hemisulfate in 0.1 M H2SO4. Proton-induced fluorescence enhancement, . Determined by eqn (2). Determined by eqn (3). |
λabs, 10−4 M H+/nm |
392 |
389 |
380 |
Log ε10−4 M H+b |
3.49 |
4.03 |
4.05 |
λabs, 10−11 M H+/nm |
406 |
413 |
401 |
Log ε10−11 M H+b |
3.38 |
3.94 |
3.97 |
λisos/nm |
336, 409 |
321, 405 |
315, 394 |
λem, 10−4 M H+/nmc |
540 |
536 |
520 |
Δλ10−4 M H+/nmd |
148 |
147 |
140 |
Δv10−4 M H+/cm−1e |
67 568 |
68 027 |
71 429 |
ΦF, 10−4 M H+f |
0.066 |
0.050 |
0.036 |
ΦF, 10−11 M H+f |
0.002 |
0.002 |
0.002 |
FEg |
72 |
22 |
13 |
pKah |
8.6 |
7.5 |
7.1 |
i |
8.3 |
7.5 |
7.0 |
An analysis of the absorbance–pH plots provided ground state proton binding constants (pKa, pβH+) (Fig. S21–S24†). For 1 in water, and 3 and 4 in all solvent compositions (Fig. S17a, S19 and S20†), significant changes occur in the absorbance maxima upon protonation. In the three solvents, the spectra of 4 (and 1 in water) exhibits simultaneous changes to the absorbance and wavelength. These observations are consistent with a twisted ICT (TICT) state due to charge repulsion and a geometry change on protonation of the piperazine.29 The spectra of 2 shows no such changes, while with 3 only a slight change in the wavelength is observed.
The emission properties of 1–4 (Fig. S25–S28†) as a function of acidity are tabulated (Tables 1 and S1†). The λmax in water at 10−4 M H+ of 1–4 appear between 540 nm and 549 nm. In methanol, the λmax is red-shifted by 8 nm to 20 nm. H+-induced Stokes shifts of ca. 140 nm (71
429 cm−1) occur. The ΦF in acid ranges between 2% and 7%, and in base from 0.2% to 3%. Clear digital off–on switching across the solvent polarity continuum is identified with 4 between 10−11 M H+ (vial B) and 10−4 M H+ (vial A). Irradiated with a 365 nm UV lamp, acidic solutions of 4 emit yellow light in water and green light in methanol (Fig. 1).
 |
| Fig. 1 Molecule 4 irradiated with 365 nm light in water, 1 : 1 (v/v) water/methanol and methanol with 10−4 M H+ (vials A) and 10−11 M H+ (vials B). | |
Fig. 2 shows the fluorescence spectra of 4 in water as a function of (a) H+, (b) Ba2+ and (c) water/methanol solvent composition. Titration with acid from 10−11 M H+ to 10−4 M H+ results in a fluorescence enhancement (FE) of 72 with a peak maximum at 540 nm. The emission increases due to protonation of the piperazine nitrogen atom, which inhibits the TICT from the lone pairs of the aliphatic nitrogen atom to the fluorophore.5,29 Titration with Ba2+ in 10−4 M H+ results in a further emission increase due to complexation of Ba2+ within the crown cavity (Fig. 2b), which raises the oxidation potential Eox of the benzocrown and consequently raises ΔGPET so that PET from the benzocrown to the 4-aminonaphthalimide is prevented. We also observe in Fig. 2c, counterintuitively and unlike 1–3 (Table S1†), an increase in the emission (and ΦF, Table 1) of 4 on changing the solvent from methanol to water. Being a π → π* transition, we naturally expect the carbonyl oxygen atoms to be more basic in the excited ICT state than in the ground state.14 This observation suggests that 4 is less strongly hydrogen bonded at a carbonyl oxygen in the excited state than in the ground state, perhaps due to a conformational change associated with the more flexible and bulky ethoxy linkages within the benzo-18-crown-6, which alters the solvent shell.
 |
| Fig. 2 Emission spectra in water of 14 μM 4 as a function of (a) H+, (b) Ba2+ at 10−4 M H+, and (c) methanol/water solvent compositions at 10−4 M H+, and the intensity–concentration profiles. λex = 400 nm. | |
From the fluorescence titrations of 1–4, the excited state proton binding constants
and cation binding constants
were evaluated. The binding constant is defined as the concentration at which half of a receptor is occupied by the specific analyte. Proton binding constants were determined from absorbance (eqn (2)) and
|
 | (2) |
|
 | (3) |
fluorescence (
eqn (3)) intensity plots using Henderson–Hasselbalch equations, which produce a sigmoidal titration curve.
30 The analyte-intensity data was fitted as a non-linear equation, and the binding constants were obtained from the point of inflection (Fig. S29–S32
†) where
IF,
IF,max and
IF,min are the observed, maximum and minimum fluorescence intensities. A similar equation was used to calculate the metal cation binding constants

with the benzocrown ethers.
25 The

of
1–4 are 8.4 ± 0.2 in water, 7.6 ± 0.1 in 1
![[thin space (1/6-em)]](https://www.rsc.org/images/entities/char_2009.gif)
:
![[thin space (1/6-em)]](https://www.rsc.org/images/entities/char_2009.gif)
1 (v/v) water/methanol and 7.1 ± 0.3 in methanol. The

of
3 is 0.92 in water and 1.4 in 1
![[thin space (1/6-em)]](https://www.rsc.org/images/entities/char_2009.gif)
:
![[thin space (1/6-em)]](https://www.rsc.org/images/entities/char_2009.gif)
1 (v/v) water/methanol (Fig. S33–S35
†). These values are greater those of anthracene-based logic gates.
2,12 The

of
4 in water is 2.9 (Fig. S36–S38
†) and 3.7 in 1
![[thin space (1/6-em)]](https://www.rsc.org/images/entities/char_2009.gif)
:
![[thin space (1/6-em)]](https://www.rsc.org/images/entities/char_2009.gif)
1 (v/v) water/methanol (Fig. S39–S41
†). No evidence for metal ion binding was observed with
2 bearing the 3,4-dimethoxyphenyl moiety.
Molecules 3 and 4 displays logic characteristics dependent on the solvent conditions (Fig. S42† and 3). The threshold limit is set at half of the maximum fluorescence of the most intense emission spectrum (1, 1). In water, 3 and 4 behave as single-input H+-driven YES gates. When Na+ and Ba2+, respectively, are included as inputs in water, 3 and 4 function as a two-input H+, M+-driven TRANSFER logic gates (Fig. S42a† and 3a). A third cation, such as K+ or Hg2+ (pβK+ = 2.3, pβHg2+ = 3.4 in water, Fig. S36–S38†) can also bind within the benzo-18-crown-6 cavity to yield an integrated OR–AND gate algebraically expressed as (A + B)·C.1a
 |
| Fig. 3 Fluorescence emission spectra of 14 μM 4 in (a) water and (b) methanol. The red line denotes the digital threshold, which was set at ΦF,max/2. a Low input: 10−11 M H+, high input: 10−4 M H+; b low input: 0 mM Ba2+, high input: 14 mM Ba2+; c measured with reference to quinine hemisulfate in 0.1 M H2SO4. | |
In methanol, 4 functions as a two-input H+, Ba2+-driven AND logic gate (Fig. 3b) and 3 as a two-input H+, Na+-driven AND gate (Fig. S42b†). At high H+ and Ba2+ levels, the emission of 4 is high with a ΦF of 0.153 and a switching ratio of 51. For comparison, 3 has a high ΦF of 0.163 and turn-on ratio of 9 at high H+ and Na+ levels. This tale of two solvents is an example of logic function tuning by solvent polarity.15,31 Rather interestingly, the optimal ΦF of 3 is observed in methanol while for 4 it is observed in 1
:
4 (v/v) water/methanol (Fig. 4), the latter with a 5-fold emission enhancement. The addition of water to 3 in the presence of H+ and Na+ in pure methanol causes a clear decreasing trend in the ΦF output (Fig. 4,
symbol). However, in the case of 4 in the presence of H+ and Ba2+, an increase of up to 20% water in methanol enhances the ΦF output, which on further addition of water, then causes a decrease in the ΦF output (Fig. 4,
symbol). Labelled with respect to the volume fraction of water, the x-axis of Fig. 4 is also indicative of the dielectric constant ε ranging from 33 to 80 for methanol and water, respectively. The general trend is that the ΦF decreases with increasing ε.
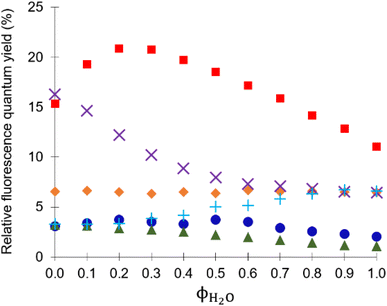 |
| Fig. 4 Fluorescence quantum yields (ΦF) in different water/methanol solvent compositions as a water volume fraction at 10−4 M H+ for ( ) 9 μM 1-H+, ( ) 10 μM 2-H+, ( ) 9 μM 3-H+, ( ) 9 μM 3-H+ and 10−2 M Na+, ( ) 14 μM 4-H+, and ( ) 14 μM 4-H+ and 10−2 M Ba2+. | |
1H NMR study
1H NMR spectra were examined as a function of pD and either pNa or pBa for 3 and 4 in CD3OD (Fig. S47† and 5) and D2O (Fig. S43 and S45†).32 Fig. 5 and S47† show the 1H NMR spectra of 3 and 4 in CD3OD as ‘truth-table’ studies under four input conditions. In D2O 3 was not fully soluble without acid so only two states were examined (Fig. S45†), while 4 was soluble for all four conditions. We alert the reader to the fact that the NMR measurements are ground state measurements, so any effect of D+, OD−, Na+ and Ba2+ is not directly comparable to the emission results. Generally, the 1H NMR spectra can be qualitatively divided into three regions. As illustrated in Fig. 5a, between 6.9 ppm and 8.7 ppm, there are sharp signals attributed to the five naphthalimide protons (H1–H5) at 7.4–8.7 ppm, and the three phenyl protons (H10–H12) at 6.9–7.4 ppm. The multiplets at 3.7–4.5 ppm are due to the benzocrown (H13–H22) and those at 3.4–3.5 ppm are due to the piperazine (H6–H9).
 |
| Fig. 5 1H NMR spectra of 8 mM 4 in CD3OD: (a) 50 mM NaOD added, (b) 50 mM DCl added, (c) 50 mM NaOD and 85 mM Ba2+ added, and (d) 50 mM DCl and 85 mM Ba2+ added. | |
The uppermost 1H NMR spectrum of 4 in CD3OD (Fig. 5a) was recorded in the presence of NaOD to ensure that the logic gate was unprotonated. The five naphthalimide protons appear at 7.4 ppm and 7.8 ppm as a doublet and apparent triplet, and as a superimposed cluster of three resonances at 8.5–8.7 ppm. The three phenyl protons are resolved as three multiplets at 6.8–7.2 ppm. On protonation of 4 to 4-D+ (Fig. 5b) in CD3OD, there is a large ΔδH = 0.42 ppm for the resonances at 3.2 ppm, attributed to the piperazine protons (H6–H9). Likewise with 3 (Fig. S47†), a ΔδH = 0.34 ppm is observed on protonation. In D2O, the ΔδH is less subtle at 0.20 ppm. Otherwise, the crown ether protons (H13–H22) are negligibly shifted, and there is only a slight upfield ΔδH for H10–H12, a slight downfield shift of the naphthalimide protons H2 and H4, and no change for naphthalimide protons H1, H3 and H5. These results confirm protonation occurs at the aliphatic piperazine nitrogen atom.
Addition of Ba2+ to 4 in CD3OD results in a downfield shift of 0.17–0.28 ppm for the crown ether protons H13–H22 and broadening with loss of fine structure for the phenyl protons H10–H12 (Fig. 5c). The crown ether proton signals are distorted, while the piperazine and naphthalimide proton signals are negligibly affected. Hence, the data indicates that binding of Ba2+ is restricted to the benzo-18-crown-6 ether. Addition of both D+ and Ba2+ results in downfield shifts for all protons with the exception of naphthalimide protons H1, H3 and H5, which become better resolved (Fig. 5d). The lack of any significant chemical shift for H1, H3 and H5 suggests the carbonyl oxygen atoms do not assist with metal ion binding in the ground state.
A similar 1H NMR analysis of 4 was performed in D2O (Fig. S43†). The same three regions are clearly visible, although the aromatic region is more complex (Fig. S43a†). On addition of DCl, the resulting spectrum of 4-D+ is simplified and parallels the observations in CD3OD (Fig. S43b†). The piperazine resonances for protons H6–H9 shift downfield ΔδH = 0.20 ppm and the crown ether protons H13–H22 shift upfield by ΔδH 0.28 ppm. In contrast, addition of Ba2+ results in a downfield shift of the crown ether protons by 0.1–0.2 ppm (Fig. S43c†). This result clearly demonstrates that 4 is perturbed by Ba2+ exclusively about the benzocrown in basic conditions. In the presence of both D+ and Ba2+ (Fig. S43d†) the piperazine protons are downfield by ΔδH = 0.4 ppm, the crown ether protons are better resolved, and the benzocrown protons move upfield 0.20 ppm.
A 1H NMR titration in D2O was performed on 4 as a function of increasing aliquots of Ba2+ (Fig. S44†). All eight of the aromatic proton signals shift downfield by up to ΔδH = 0.3 ppm on addition of 10 equivalents of Ba2+. The apparent triple at 7.0 ppm, assigned to overlapping H2 and H11, is resolved into two doublets on addition of 50 equivalents of Ba2+. Similarly, 1H NMR titration on 3 as a function of increasing aliquots of Na+ (Fig. S46†) also shows a general downfield shift trend, which is most prominent at 100 equivalents (1200 mM). The piperazine protons H6–9 and the benzocrown protons H13–22 in conjunction with the phenyl protons H10–12 are electronically decoupled via the spacer units within the receptor1–spacer1–fluorophore–spacer2–receptor2 design and thus do not contribute to synergistic 1H NMR chemical shifts.
Conclusions
A set of four 4-piperazino-N-aryl-1,8-naphthalimide molecular logic gates incorporating virtual C0-type and ethylene (C2-type) spacers, and benzocrown ether and piperazine moieties, were synthesised. Metal ion and proton binding constants were evaluated. We highlight that the benzo-18-crown-6 ether attached to the naphthalimide can capture Ba2+. Furthermore, the crown-containing molecules were demonstrated as solvent-polarity fluorescent logic gates, specifically as two-input H+, Na+-driven or H+, Ba2+-driven TRANSFER gates in water, and two-input H+, Na+-driven and H+, Ba2+-driven AND gates in methanol, for the respective crown ethers. An interesting finding is that the on state of the benzo-15-crown-5 gate is brightest in methanol, while the benzo-18-crown-6 gate is brightest in 1
:
4 (v/v) water/methanol.
1H NMR titration experiments were performed in D2O and CD3OD in order to examine the four logic (ground) states for the benzo-18-crown-6 logic gate. For the benzo-15-crown-5 logic gate, quality NMR spectra could only be obtain for the two states in acidic media due to limited solubility of the neutral compound at millimolar concentrations. These experiments nicely confirmed that the spacer units electronically decouple the fluorophore from the two receptors within the receptor1–spacer1–fluorophore–spacer2–receptor2 design. The NMR experiments also provide strong evidence for metal ion binding within the benzocrown cavity. However, there was no conclusive evidence for ground state interactions between a carbonyl of the naphthalimide and the metal ion within the benzocrown cavity. Therefore, the carbonyl oxygen atoms within the excited ICT fluorophore must be more basic in the excited state than in the ground state to account for the stronger metal ion binding interactions.26
These results should provide insight into the development of solvent-polarity reconfigurable fluorescent logic gates for probing the microenvironments at cellular membranes and protein interfaces.10
Experimental
A list of chemicals and instrumentation are provided in the ESI.† The syntheses of 1–4 are shown in Scheme 1.
General method for synthesis of 4-piperazino-N-aryl-1,8-naphthalimides
The 4-chloro-N-aryl-1,8-naphthalimide and excess piperazine (10–15 equivalents) were dissolved in 10 mL of DMF. The contents within the 50 mL round-bottom flask were heated and stirred at 130 °C until completion. The DMF was removed under vacuum by rotatory evaporator as an azeotropic mixture with heptane. The products were isolated by precipitation from a suitable solvent.
4-Piperazino-N-phenyl-1,8-naphthalimide (1)
4-Chloro-N-phenyl-1,8-naphthalimide (1.00 g, 3.25 mmol) and piperazine (3.46 g, 40.0 mmol) were reacted (general method). Addition of distilled cold water to the mixture gave an orange precipitate. The solid was collected by filtration and washed with cold distilled water and dried (1.07 g, 92% yield). Rf (9
:
1 (v/v) CH2Cl2/MeOH) = 0.88; m.p. 230 °C (dec.); 1H-NMR (500 MHz, CDCl3, ppm): δ 8.68 (dd, 1H, J = 7.3 Hz, 1.0 Hz, Naph-H), 8.60 (d, 1H, J = 8.0 Hz, Naph-H), 8.51 (dd, 1H, J = 8.5 Hz, 1.0 Hz, Naph-H), 7.81 (dd, 1H, J = 8.5 Hz, 7.3 Hz, Naph-H), 7.57 (m, 2H, Ph-H), 7.50 (m, 1H, Ph-H), 7.34 (m, 2H, Ph-H), 7.30 (d, 1H, J = 8.0 Hz, Naph-H), 3.93 (br, s, 2H, Pip-H), 3.76 (m, 2H, Pip-H), 3.33 (m, 2H, Pip-H), 3.28 (m, 2H, Pip-H); 13C-NMR (125 MHz, CDCl3, ppm): δ 40.21 (CH2), 45.77 (CH2), 52.72 (CH2), 53.53 (CH2), 115.73 (CH), 117.95 (C), 123.60 (C), 126.35 (C), 126.50 (CH), 128.70 (2CH), 129.36 (2CH), 129.97 (CH), 130.24 (C), 131.72 (CH), 132.75 (CH), 135.49 (C), 155.27 (C), 164.00 (CO), 164.48 (CO); IR νmax (NaCl, cm−1): 3064, 2906, 2831, 1699, 1653, 1560, 1507, 1369, 1232, 1004, 787, 740; HRMS (ES-TOF, m/z): calculated C22H20N3O2 358.1556, found 358.1550.
4-Piperazino-N-(3,4-dimethoxyphenyl)-1,8-naphthalimide (2)
4-Chloro-N-(3,4-dimethoxyphenyl)-1,8-naphthalimide (500 mg, 1.36 mmol) and piperazine (1.53 g, 17.8 mmol) were reacted (general method). The orange solid was collected by filtration, washed with cold distilled water and dried (440 mg, 77% yield). Rf (9
:
1 (v/v) CH2Cl2/MeOH) = 0.90; m.p. 190 °C (dec.); 1H-NMR (500 MHz, CDCl3, ppm): δ 8.67 (dd, 1H, J = 7.2 Hz, 0.8 Hz, Naph-H), 8.58 (d, 1H, J = 8.0 Hz, Naph-H), 8.48 (dd, 1H, J = 8.5 Hz, 0.8 Hz, Naph-H), 7.79 (dd, 1H, J = 8.4 Hz, 7.4 Hz, Naph-H), 7.27 (d, 1H, J = 8.2 Hz, Naph-H), 7.02 (d, 1H, J = 8.5 Hz, Ph-H), 6.87 (dd, 1H, J = 8.4 Hz, 2.3 Hz, Ph-H), 6.80 (d, 1H, J = 2.3 Hz, Ph-H), 3.94 (s, 3H, –OCH3), 3.91 (br, s, 2H, Pip-H), 3.88 (s, 3H, –OCH3), 3.74 (m, 2H, Pip-H), 3.28 (m, 4H, Pip-H); 13C-NMR (125 MHz, CDCl3, ppm): δ 40.21 (CH2), 45.77 (CH2), 52.73 (CH2), 53.52 (CH2), 55.94 (–OCH3), 56.05 (–OCH3), 111.42 (CH), 111.78 (CH), 115.72 (CH), 117.95 (C), 120.76 (CH), 123.61 (C), 126.35 (CH), 128.16 (C), 129.98 (CH), 130.20 (C), 131.75 (CH), 132.78 (CH), 149.17 (C), 149.61 (C), 155.26 (C), 164.23 (CO), 164.71 (CO); IR νmax (NaCl, cm−1): 3008, 2929, 2835, 1705, 1662, 1585, 1512, 1436, 1369, 1238, 1002, 754; HRMS (ES-TOF, m/z): calculated C24H24N3O4 418.1767, found 418.1752.
4-Piperazino-N-(benzo-15-crown-5)-1,8-naphthalimide (3)
4-Chloro-N-(benzo-15-crown-5)-1,8-naphthalimide (500 mg, 1.00 mmol) and piperazine (1.17 g, 13.5 mmol) were reacted (general method). The mixture was extracted using dichloromethane and water/triethylamine. The organic layer was collected and reduced under vacuum by rotary evaporator. Addition of cold acetone gave an orange precipitate. The solid was collected by filtration and washed with cold acetone and diethyl ether and dried (520 mg, 94% yield). Rf (9
:
1 (v/v) CH2Cl2/MeOH) = 0.60; m.p. 246 °C (dec.); 1H-NMR (500 MHz, CDCl3, ppm): δ 8.66 (dd, 1H, J = 7.3 Hz, 1.1 Hz, Naph-H), 8.57 (d, 1H, J = 8.0 Hz, Naph-H), 8.47 (dd, 1H, J = 8.5 Hz, 1.1 Hz, Naph-H), 7.78 (dd, 1H, J = 8.4 Hz, 7.3 Hz, Naph-H), 7.27 (d, 1H, Naph-H overlapped by CHCl3 residual speak), 7.00 (d, 1H, J = 8.5 Hz, Ph-H), 6.85 (dd, 1H, J = 8.5 Hz, 2.4 Hz, Ph-H), 6.80 (d, 1H, J = 2.4 Hz, Ph-H), 4.21 (m, 2H, Crown-H), 4.14 (m, 2H, Crown-H), 3.94 (m, 2H, Crown-H), 3.92 (br, s, 2H, Pip-H), 3.89 (m, 2H, Crown-H), 3.72–3.80 (m, 10H, Crown-H), 3.30 (m, 2H, Pip-H), 3.25 (m, 2H, Pip-H); 13C-NMR (125 MHz, CDCl3, ppm): δ 40.22 (Pip-CH2), 45.76 (Pip-CH2), 52.72 (Pip-CH2), 53.53 (Pip-CH2), 69.22 (Crown-CH2), 69.36 (Crown-CH2), 69.59 (Crown-CH2), 69.68 (Crown-CH2), 70.68 (Crown-CH2), 70.70 (Crown-CH2), 71.23 (Crown-CH2), 71.27 (Crown-CH2), 114.19 (CH), 114.59 (CH), 115.72 (CH), 118.05 (C), 121.34 (CH), 123.67 (C), 126.32 (CH), 126.50 (C), 128.56 (C), 129.88 (CH), 130.20 (C), 131.69 (CH), 132.71 (CH), 149.33 (C), 149.71 (C), 155.19 (C), 164.09 (CO), 164.57 (CO); IR νmax (NaCl, cm−1): 3064, 2920, 2864, 1683, 1654, 1506, 1456, 1375, 1238, 1122, 1002, 771; HRMS (ES-TOF, m/z): calculated C30H34N3O7 548.2397, found 548.2379.
4-Piperazino-N-(benzo-18-crown-6)-1,8-naphthalimide (4)
4-Chloro-N-(benzo-18-crown-6)-1,8-naphthalimide (203 mg, 3.70 mmol) and piperazine (408 mg, 4.86 mmol) were reacted (general method). Acetonitrile and diethyl ether were added to the solution mixture resulting in an orange precipitate. The solid was collected by filtration, and washed with cold diethyl ether and dried (175 mg, 81% yield). Rf (9
:
1 (v/v) CH2Cl2/MeOH) = 0.50; m.p. 240 °C (dec.); 1H-NMR (500 MHz, CDCl3, ppm): δ 8.61 (d, 1H, J = 7.2 Hz, Naph-H), 8.55 (d, 1H, J = 8.1 Hz, Naph-H), 8.47 (d, 1H, J = 8.5 Hz, Naph-H), 7.72 (t, 1H, J = 7.9 Hz, Naph-H), 7.24 (d, 1H, J = 8.1 Hz, Naph-H), 7.00 (d, 1H, J = 8.4 Hz, Ph-H), 6.85 (dd, 1H, J = 8.4 Hz, 2.1 Hz, Ph-H), 6.81 (d, 1H, J = 2.1 Hz, Ph-H), 4.22 (m, 2H, Crown-H), 4.16 (m, 2H, Crown-H), 3.95 (m, 2H, Crown-H), 3.90 (m, 2H, Crown-H), 3.71–3.79 (m, 12H, Crown-H), 3.27 (m, 4H, Pip-H), 3.22 (m, 4H, Pip-H), 2.95 (s, 2H, Pip-NH); 13C-NMR (125 MHz, CDCl3, ppm): δ 46.26 (2× Pip-CH2), 54.40 (2× Pip-CH2), 69.02 (Crown-CH2), 69.18 (Crown-CH2), 69.48 (Crown-CH2), 69.57 (Crown-CH2), 70.68 (Crown-CH2), 70.72 (Crown-CH2), 70.84 (2× Crown-CH2), 70.90 (Crown-CH2), 70.92 (Crown-CH2), 113.87 (CH), 114.24 (CH), 115.02 (CH), 116.75 (C), 121.24 (CH), 123.44 (C), 125.70 (CH), 126.32 (C), 128.66 (C), 130.31 (C), 130.65 (CH), 131.51 (CH), 133.00 (CH), 148.93 (C), 149.71 (C), 156.65 (C), 164.31 (CO), 164.81 (CO); IR νmax (NaCl, cm−1): 3064, 2914, 1695, 1652, 1585, 1506, 1456, 1375, 1247, 1122, 945, 756; HRMS (ES-TOF, m/z): calculated C32H38N3O8 592.2653, found 592.2657.
Author contributions
Conceptualization (DCM), investigation (GG), formal analysis (GG, DCM), supervision (DCM), writing – original draft (GG, DCM) and writing – review & editing (GG, DCM).
Conflicts of interest
There are no conflicts of interest to declare.
Acknowledgements
Financial support is acknowledged from the University of Malta. Prof. Robert M. Borg and Dr Duncan Micallef are thanked for NMR support.
References
-
(a) A. P. de Silva, Molecular Logic-based Computation, The Royal Society of Chemistry, Cambridge, UK, 2013 Search PubMed;
(b) K. Szaciłowski, Infochemistry, Wiley-VCH, Chichester, UK, 2012 CrossRef;
(c) Molecular and Supramolecular Information Processing: From Molecular Switches to Logic Systems, ed. E. Katz, Wiley-VCH Verlag, Weinheim, Germany, 2012 Search PubMed;
(d) Biomolecular Information Processing: From Logic Systems to Smart Sensors and Actuators, ed. E. Katz, Wiley-VCH Verlag, Weinheim, Germany, 2012 Search PubMed.
- A. P. de Silva, H. Q. N. Gunaratne and C. P. McCoy, Nature, 1993, 364, 42 CrossRef.
-
(a) B. Daly, J. Ling and A. P. de Silva, Chem. Soc. Rev., 2015, 44, 4203 RSC;
(b) J. Ling, B. Daly, V. A. D. Silverson and A. P. de Silva, Chem. Commun., 2015, 51, 8403 RSC;
(c) A. P. de Silva, T. S. Moody and G. D. Wright, Analyst, 2009, 134, 2385 RSC;
(d) D. C. Magri, Analyst, 2015, 140, 7487 RSC.
-
(a) C.-Y. Yao, H.-Y. Lin, H. S. N. Cory and A. P. de Silva, Mol. Syst. Des. Eng., 2020, 5, 1325 RSC;
(b) D. C. Magri, Coord. Chem. Rev., 2021, 426, 213598 CrossRef CAS;
(c) S. Erbas-Cakmak, T. Gunnlaugsson, S. Kolemen, T. D. James, A. C. Sedgwick, J. Yoon and E. U. Akkaya, Chem. Soc. Rev., 2018, 47, 2266 RSC;
(d) J. Andréasson and U. Pischel, Chem. Soc. Rev., 2018, 47, 2228 RSC.
-
(a) G. J. Scerri, J. C. Spiteri, C. J. Mallia and D. C. Magri, Chem. Commun., 2019, 55, 4961 RSC;
(b) J. M. A. Spiteri, C. J. Mallia, G. J. Scerri and D. C. Magri, Org. Biomol. Chem., 2017, 15, 10116 RSC;
(c) D. C. Magri, M. Camilleri Fava and C. J. Mallia, Chem. Commun., 2014, 50, 1009 RSC;
(d) D. C. Magri, G. J. Brown, G. D. McClean and A. P. de Silva, J. Am. Chem. Soc., 2006, 128, 4950 CrossRef CAS.
-
(a) G. J. Scerri, J. C. Spiteri and D. C. Magri, Mater. Adv., 2021, 2, 434 RSC;
(b) J. C. Spiteri, A. D. Johnson, S. A. Denisov, G. Jonusauskas, N. D. McClenaghan and D. C. Magri, Dyes Pigm., 2018, 157, 278 CrossRef CAS;
(c) J. C. Spiteri, S. A. Denisov, G. Jonusauskas, S. Klejna, K. Szaciłowski, N. D. McClenaghan and D. C. Magri, Org. Biomol. Chem., 2018, 16, 6195 RSC;
(d) A. D. Johnson, K. A. Paterson, J. C. Spiteri, S. A. Denisov, G. Jonusauskas, A. Tron, N. D. McClenaghan and D. C. Magri, New J. Chem., 2016, 40, 9917 RSC;
(e) J. C. Spiteri, J. S. Schembri and D. C. Magri, New J. Chem., 2015, 39, 3349 RSC;
(f) T. J. Farrugia and D. C. Magri, New J. Chem., 2013, 37, 148 RSC.
-
(a) J. K. Tusa and H. He, J. Mater. Chem., 2005, 15, 2640 RSC;
(b) H. He, M. A. Mortellaro, M. J. P. Leiner, S. T. Young, R. J. Fraatz and J. K. Tusa, Anal. Chem., 2003, 75, 549 CrossRef CAS;
(c) T. Gunnlaugsson, M. Nieuwenhuyzen, L. Richard and V. Thoss, J. Chem. Soc., Perkin Trans. 2, 2002, 141 CAS;
(d) T. Gunnlaugsson, M. Nieuwenhuyzen, L. Richard and V. Thoss, Tetrahedron Lett., 2001, 42, 4725 CrossRef CAS;
(e) T. Gunnlaugsson and J. P. Leonard, Chem. Commun., 2003, 2424 RSC;
(f) T. Konry and D. R. Walt, J. Am. Chem. Soc., 2009, 131, 13232 CrossRef CAS PubMed;
(g) S. Erbas-Cakmak, O. A. Bozdemir, Y. Cakmak and E. U. Akkaya, Chem. Sci., 2013, 4, 858 RSC.
-
(a) U. Reddy G, J. Axthelm, P. Hoffmann, N. Taye, S. Gläser, H. Görls, S. L. Hopkins, W. Plass, U. Neugebauer, S. Bonnet and A. Schiller, J. Am. Chem. Soc., 2017, 139, 4991 CrossRef;
(b) V. Ramu, G. Upendar Reddy, J. Liu, P. Hoffmann, R. Sollapur, R. Wyrwa, S. Kupfer, C. Spielmann, S. Bonnet, U. Neugebauer and A. Schiller, Chem.–Eur. J., 2019, 25, 8453 CrossRef CAS.
-
(a) N. Zerafa, M. Cini and D. C. Magri, Mol. Syst. Des. Eng., 2021, 6, 93 RSC;
(b) M. E. S. West, C.-Y. Yao, G. Melaugh, K. Kawamoto, S. Uchiyama and A. P. de Silva, Chem.–Eur. J., 2021, 27, 13268 CrossRef CAS PubMed;
(c) M. Vella Refalo, N. V. Farrugia, A. D. Johnson, S. Klejna, K. Szacilowski and D. C. Magri, J. Mater. Chem. C, 2019, 7, 15225 RSC;
(d) C.-Y. Yao, J. Ling, L.-Y.-H. Chen and A. P. de Silva, Chem. Sci., 2019, 10, 2272 RSC;
(e) A. P. de Silva, M. R. James, B. O. F. McKinney, D. A. Pears and S. M. Weir, Nat. Mater., 2006, 5, 787 CrossRef PubMed.
-
(a) C. Geraghty, C. Wynne and R. B. P. Elmes, Coord. Chem. Rev., 2021, 437, 213713 CrossRef CAS;
(b) W. Wang, J. Chen, H. Ma, W. Xing, N. Lv, B. Zhang, H. Xu and W. Wang, Chem. Commun., 2021, 57, 8166 RSC;
(c) A. D. Johnson, J. A. Buhagiar and D. C. Magri, RSC Med. Chem., 2021, 12, 2060 RSC;
(d) G. D. Wright, Y. Chao-Yi, T. S. Moody and A. P. de Silva, Chem. Commun., 2020, 56, 6838 RSC;
(e) Y. Zhang, W. W. Chen, Y. Y. Fang, X. B. Zhang, Y. Liu and H. X. Ju, J. Am. Chem. Soc., 2021, 143, 15233 CrossRef CAS PubMed;
(f) B. McLaughlin, E. M. Surender, G. D. Wright, B. Daly and A. P. de Silva, Chem. Commun., 2018, 54, 1319 RSC.
-
(a) J. Ling, G. Naren, J. Kelly, T. S. Moody and A. P. de Silva, J. Am. Chem. Soc., 2015, 137, 3763 CrossRef CAS;
(b) J. Ling, G. Naren, J. Kelly, D. B. Fox and A. P. de Silva, Chem. Sci., 2015, 6, 4472 RSC.
- A. P. de Silva, H. Q. N. Gunaratne and C. P. McCoy, J. Am. Chem. Soc., 1997, 119, 7891 CrossRef CAS.
- I. Johnson and M. T. Z. Spence, The molecular probes handbook: a guide to fluorescent probes and labeling technologies, 11th edn, 2010, Life Technologies Corporations, USA Search PubMed.
- For example: A. P. de Silva, H. Q. N. Gunaratne, J. L. Habib-Jiwan, C. P. McCoy, T. E. Rice and J. P. Soumillion, Angew. Chem., Int. Ed., 1995, 107, 1728 CrossRef.
-
(a) S. Uchiyama, E. Fukatsu, G. D. McClean and A. P. de Silva, Angew. Chem., Int. Ed., 2016, 55, 768 CrossRef CAS PubMed;
(b) A. P. de Silva and K. R. A. S. Sandanayake, Tetrahedron Lett., 1991, 32, 421 CrossRef;
(c) A. P. de Silva and K. R. A. S. Sandanayake, J. Chem. Soc., Chem. Commun., 1989, 1183 RSC.
- N. Jiang, J. Fan, F. Xu, X. Peng, H. Mu, J. Wang and X. Xiong, Angew. Chem., Int. Ed., 2015, 54, 1 CrossRef.
- K. Pal, P. Kumar and A. Lal Koner, J. Photochem. Photobiol., B, 2020, 206, 111848 CrossRef CAS PubMed.
- Reviews:
(a) J. Li, D. Yim, W. D. Jang and J. Yoon, Chem. Soc. Rev., 2017, 46, 2437 RSC;
(b) P. A. Panchenko, O. A. Fedorova and Y. V. Fedorov, Russ. Chem. Rev., 2014, 83, 155 CrossRef;
(c) G. W. Gokel, W. M. Leevy and M. E. Weber, Chem. Rev., 2004, 104, 2723 CrossRef CAS PubMed;
(d) G. W. Gokel, L. J. Barbour, R. Ferdani and J. Hu, Acc. Chem. Res., 2002, 35, 878 CrossRef CAS PubMed;
(e) G. W. Gokel, Chem. Soc. Rev., 1992, 21, 39 RSC.
- Examples:
(a) P. A. Panchenko, Y. V. Fedorov, O. A. Fedorova and G. Jonusauskas, Dyes Pigm., 2013, 98, 347 CrossRef CAS;
(b) P. A. Panchenko, Y. V. Fedorov, O. A. Fedorova and G. Jonusauskas, Phys. Chem. Chem. Phys., 2015, 17, 22749 RSC;
(c) P. A. Panchenko, Y. V. Fedorov, V. P. Perevalov, G. Jonusauskas and O. A. Fedorova, J. Phys. Chem. A, 2010, 114, 4118 CrossRef CAS PubMed;
(d) P. A. Panchenko, Y. V. Fedorov and O. A. Fedorova, J. Photochem. Photobiol., A, 2018, 364, 124 CrossRef CAS;
(e) P. A. Panchenko, A. S. Polyakova, Y. V. Fedorov and O. A. Fedorova, Mendeleev Commun., 2019, 29, 155 CrossRef CAS;
(f) C. Hou, A. M. Urbanec and H. Cao, Tetrahedron Lett., 2011, 52, 4903 CrossRef CAS;
(g) P. Nandhikonda, M. P. Begaye and M. D. Heagy, Tetrahedron Lett., 2009, 50, 2459 CrossRef CAS;
(h) G. W. Gokel, S. L. De Wall and E. S. Meadows, Eur. J. Org. Chem., 2000, 17, 2967 CrossRef.
- Recent examples:
(a) F. Nicoli, M. Baroncini, S. Silvi, J. Groppi and A. Credi, Org. Chem. Front., 2021, 8, 5531 RSC;
(b) Z. Liu, H. Zhang and J. Han, Org. Biomol. Chem., 2021, 19, 3287 RSC;
(c) S. An, Q. Xu, Z. Ni, J. Hu, C. Peng, L. Zhai, Y. Guo and H. Liu, Angew. Chem., Int. Ed., 2021, 60, 9959 CrossRef CAS PubMed.
-
(a) P. Thapa, I. Arnquist, N. Byrnes, A. A. Denisenko, F. W. Foss Jr, B. J. P. Jones, A. D. McDonald, D. R. Nygren and K. Woodruff, Sci. Rep., 2019, 9, 15097 CrossRef CAS PubMed;
(b) A. D. McDonald, et al., Phys. Chem. Lett., 2018, 120, 132504 CAS;
(c) N. Byrnes, F. W. Foss Jr, B. J. P. Jones, A. D. McDonald, D. R. Nygren, P. Thapa and A. Trinidad, J. Phys.: Conf. Ser., 2019, 1312, 012001 CrossRef CAS.
- I. Rivell, et al., Nature, 2020, 583, 48 CrossRef PubMed.
- P. Thapa, N. K. Byrnes, A. A. Denisenko, J. X. Mao, A. D. McDonald, C. A. Newhouse, T. T. Vuong, K. Woodruff, K. Nam, D. R. Nygren, B. J. P. Jones and F. W. Foss Jr, ACS Sens., 2021, 6, 192 CrossRef CAS PubMed.
-
(a) J. F. Callan, A. P. de Silva, J. Ferguson, A. J. M. Huxley and A. M. O'Brien, Tetrahedron, 2004, 60, 11125 CrossRef CAS;
(b) D. P. Kennedy, C. D. Incarvito and S. C. Burdette, Inorg. Chem., 2010, 49, 916 CrossRef CAS PubMed.
- D. C. Magri, J. F. Callan, N. D. McClenaghan, A. P. de Silva, D. B. Fox and K. R. A. S. Sandanayake, J. Fluoresc., 2005, 15, 769 CrossRef CAS PubMed.
- A. Diacono, M. C. Aquilina, A. Calleja, G. Agius, G. Gauci, K. Szaciłowski and D. C. Magri, Org. Biomol. Chem., 2020, 18, 4773 RSC.
-
(a) R. K. Meka and M. D. Heagy, J. Org. Chem., 2017, 82, 12153 CrossRef CAS PubMed;
(b) P. Nandhikonda, M. P. Begaye, Z. Cao and M. D. Heagy, Org. Biomol. Chem., 2010, 8, 3195 RSC.
- A. Weller, Pure Appl. Chem., 1968, 16, 115 CrossRef CAS . EOX is the oxidation potentials of 1,2-dimethoxybenzene (1.45 eV) or piperazine amine (1.15 eV), ERED is the reduction potential of naphthalimide (−1.57 eV), ES is the excited state singlet energy of naphthalimide (2.55 eV) and e2/εr is the coulombic term (0.10 eV) where e is the electric charge of an electron, ε is the solvent dielectric constant and r the separation distance. Potentials are versus SCE in acetonitrile..
- W. Chi, J. Chen, Q. Qiao, Y. Gao, Z. Xub and X. Liu, Phys. Chem. Chem. Phys., 2019, 21, 16798 RSC.
- L. N. Neupane, C. Raj Lohani, J. Kim and K.-H. Lee, Tetrahedron, 2013, 69, 11057 CrossRef CAS.
- J. Hatai, S. Pal, G. P. Jose, T. Sengupta and S. Bandyopadhyay, RSC Adv., 2012, 2, 7033 RSC.
- M. A. Cardona, M. Kveder, U. Baisch, M. R. Probert and D. C. Magri, RSC Adv., 2016, 6, 84712 RSC.
Footnote |
† Electronic supplementary information (ESI) available: Experimental details, UV-vis absorption, emission, NMR, IR and mass spectra. See DOI: https://doi.org/10.1039/d2ra07568g |
|
This journal is © The Royal Society of Chemistry 2022 |
Click here to see how this site uses Cookies. View our privacy policy here.