DOI:
10.1039/D2RA06679C
(Paper)
RSC Adv., 2022,
12, 32790-32795
Metal-free visible-light-induced hydroxy-perfluoroalkylation of conjugated olefins using enamine catalyst†
Received
22nd October 2022
, Accepted 5th November 2022
First published on 15th November 2022
Abstract
We developed a simple and sustainable method for the hydroxy-perfluoroalkylation of electron-deficient conjugated olefins and styrenes. In this protcol, in situ generated enamine forms electron-donor–accepter (EDA) complexes with perfluoroalkyl iodide, and reaction proceed with visible-light irradiation. Tertiary amine also interacts with perfluoroalkyl iodide via halogen-bonding, promoting the perfluoroalkyl radical generation. This reaction does not require any transition-metal or photoredox catalyst, and gaseous oxygen is used as the green hydroxy source. Moreover, various commercially available substrates and perfluoroalkyl iodides were tolerated, affording the desired hydroxy-perfluoroalkylated products in good to moderate yields (>50 examples, up to 90%).
Introduction
Fluorine atoms have unique properties, such as the third smallest van der Waals radius, the highest electronegativity, and a strong carbon–fluorine bond.1 Therefore, the introduction of fluorine into organic compounds has attracted attention from various fields, such as pharmaceuticals,2 agrochemicals,3 and functional materials,4 owing to the substantial property changes induced by the presence of fluorine. In addition to mono-fluorination reactions,5 a number of synthetic methods for fluoroalkylation have been established.6 In particular, radical protocols7 using commonly available perfluoroalkyl iodides as radical precursors have been widely explored.8 Furthermore, the reaction of simple terminated olefins is well established because of the high electrophilicity of perfluoroalkyl radicals.9 However, the handing of electron-deficient conjugated olefins remains challenging because they can easily self-polymerize and have low reactivity toward perfluoroalkyl radicals.10 Therefore, perfluoroalkylation reactions for electron-deficient olefins are in high demand.
In recent years, reactions using photoredox catalysts under mild visible-light conditions have received significant attention owing to their sustainability.11 Thus far, various types of perfluoroalkylation reactions using valuable Ir or Ru catalysts have been reported.12 More recently, from the perspective of eco-friendliness and cost reduction, significant efforts have been devoted to develop metal-free photo-organocatalysed reactions. Research on the reaction using organic dye have been widely reported.13 Furthermore, simple molecules, such as enamine, amine, or phosphine could be used as an organocatalyst, which can form electron-donor–accepter (EDA) complexes with perfluoroalkyl iodide.14 Our group has also reported that in situ generated enamine can function as a photo-organocatalyst for the iodo-perfluoroalkylation of electron-rich unconjugated olefins (Scheme 1A).14f
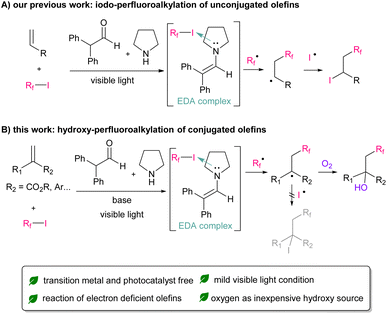 |
| Scheme 1 Perfluoroalkylation of olefins. | |
Focusing on the significant potential of enamine as an organocatalyst, we applied our perfluoroalkylation reactions on electron-deficient conjugated olefins. As a result, iodo-perfluoroalkylation reaction did not proceed owing to the instability of iodide adduct product.15 Instead, we found that hydroxy-perfluoroalkylation proceeded in the presence of molecular oxygen and tertiary amine as organic base (Scheme 1B). Herein, we present the first example of the metal-free visible-light-induced hydroxy-perfluoroalkylation of electron-deficient conjugated olefins. This reaction could be applied to various perfluoroalkyl iodides, electron-deficient olefins, and styrenes.
Results and discussion
We selected the reaction of ethyl methacrylate (1a) and 3.0 eq. of C6F13I (2a) to optimize the reaction conditions (Table 1). In our initial attempt, 10 mol% of diphenylacetaldehyde (3),14f 40 mol% of pyrrolidine (4), and 0.8 eq. of oxygen in 1,2-dichoro ethane (DCE) with white light-emitting-diode (LED) irradiation at 25 °C external temperature for 3 h afforded the desired hydroxy-perfluoroalkylated product 5aa with only 3% yield (entry 1). We hypothesized that the iodine ions generated in the system would inactivate and stop the catalytic cycle of enamine. Therefore, we increased the equivalent of 4 in hopes that 4 would capture iodine,14f but the yield did not improve (entry 2). In this case, an amide type by-product was observed in which 4 reacted with 2 and oxygen (Scheme S2†).16 Therefore, we considered that the use of excess amount of secondary amine is unsuitable because it was consumed as a by-product before it captured iodine. Afterwards, we added 1.0 eq. of N,N-diisopropylethylamine (DIPEA), which is tertiary amine and have no possibility to produce amide type by-product, instead of 4 and found that the yield improved to 37% (entry 3). Moreover, using 2.0 eq. of DIPEA further increased the yield to 53% (entry 4). From these results, we considered the possibility of generating perfluoroalkyl radicals via halogen-bond interaction between DIPEA and 2a same as previously reported.17 Therefore, experiments without 3, 4, or both 3 and 4 were conducted to confirm the necessity of the enamine catalyst (entries 5–7). In all cases, 5aa was obtained in 34–44% yield, suggesting that DIPEA also promote this reaction. However, the yields of entries 3–5 decreased compared to that of entry 2, and it indicated that both enamine and DIPEA were necessary for satisfactory yields. Additionally, replacing the enamine catalyst with 10 mol% of EY–2Na, which is known to be an effective photoredox organocatalyst for radical perfluoroalkylations,13d,g decreased the yield to 31% (entry 8). This indicates that the photoredox catalyst is incompatible with the reaction in the presence of oxygen, which emphasizes the efficacy of the enamine catalyst in this system. Subsequently, we screened several tertiary amines without enamine (entries 9–11). As a result, using of triethylamine (TEA) or 1,8-diazabicyclo[5.4.0]undec-7-ene (DBU) decreased the yield to 9–14%, and reaction did not proceed in the presence of 1,4-diazabicyclo[2.2.2]octane (DABCO). Next, we optimized the reaction time using enamine and DIPEA, and it was found that 24 h of irradiation generated product 5aa with 75% yield (entries 12–13). In addition, the oxygen equivalent was investigated. Increasing the oxygen equivalent to 1.0–2.0 eq., considerable amounts of oxygen-derived amide-type by-products were obtained,16 and the product yields decreased to 30–50% yields (entries 14 and 15, Table S1†). However, reducing the amount of oxygen to 0.5 eq. led to the oligomerization of 1a, and the yield decreased to 58% (entry 16). Therefore, 0.8 eq. of oxygen is the optimum amount for this reaction. Moreover, the reaction without oxygen yielded trace amounts of the desired product, and oligomerization of 1a proceeded instead of iodo-perfluoroalkyation (entry 17). Also, the reaction in air produced a low yield of 38% (entry 18). Finally, the reaction under the dark at 80 °C was conducted and found that thermal radical generation is not a main route (entry 19). Furthermore, we confirmed that the reaction also proceeds by using pre-synthesized enamine (Table S3†).
Table 1 Optimization of the reaction conditionsa

|
Entry |
3 (mol%) |
4 (mol%) |
Base (eq.) |
O2 (eq.) |
Time (h) |
Yield (%)b |
Reaction conditions: 1a (0.25 mmol), 2a (0.75 mmol, 3.0 eq.), 3 (0.025 mmol, 10 mol%), 4 (0.01 mmol, 40 mol%), DIPEA (0.5 mmol, 2.0 eq.), O2 (0.2 mmol, 0.8 eq.), DCE (2.5 mL), at 25 °C, in argon atmosphere, for 24 h, and under white LED irradiation. Yields based on 19F NMR spectroscopy using benzotrifluoride as an internal standard; isolated yields are given in parentheses. 10 mol% Eosin Y–2Na was used instead of 3 and 4. Under normal atmosphere. In the dark at 80 °C. |
1 |
10 |
40 |
— |
0.8 |
3 |
3 |
2 |
10 |
140 |
— |
0.8 |
3 |
4 |
3 |
10 |
40 |
DIPEA (1.0) |
0.8 |
3 |
37 |
4 |
10 |
40 |
DIPEA (2.0) |
0.8 |
3 |
53 |
5 |
— |
— |
DIPEA (2.0) |
0.8 |
3 |
34 |
6 |
10 |
— |
DIPEA (2.0) |
0.8 |
3 |
35 |
7 |
— |
40 |
DIPEA (2.0) |
0.8 |
3 |
44 |
8c |
— |
— |
DIPEA (2.0) |
0.8 |
3 |
31 |
9 |
— |
— |
TEA (2.0) |
0.8 |
3 |
14 |
10 |
— |
— |
DBU (2.0) |
0.8 |
3 |
9 |
11 |
— |
— |
DABCO (2.0) |
0.8 |
3 |
n.r |
12 |
10 |
40 |
DIPEA (2.0) |
0.8 |
6 |
61 |
13 |
10 |
40 |
DIPEA (2.0) |
0.8 |
24 |
75 (80) |
14 |
10 |
40 |
DIPEA (2.0) |
1.0 |
24 |
53 |
15 |
10 |
40 |
DIPEA (2.0) |
2.0 |
24 |
30 |
16 |
10 |
40 |
DIPEA (2.0) |
0.5 |
24 |
58 |
17 |
10 |
40 |
DIPEA (2.0) |
— |
24 |
Trace |
18d |
10 |
40 |
DIPEA (2.0) |
— |
24 |
38 |
19e |
10 |
40 |
DIPEA (2.0) |
0.8 |
24 |
10 |
After determining the optimized conditions, i.e., entry 13, we investigated the substrate scope of the electron-deficient conjugated olefins (Table 2). Significantly, the use of various methacrylates with different ester groups (ethyl, methyl, tertiary butyl, benzyl, phenyl, and cyclohexyl) afforded the corresponding hydroxy-perfluoroalkylated products 5aa–5fa in 69–81% yields. The reaction using benzyl methacrylate (1d) can be scaled up to 6.25 mmol, producing 1.22 g of 5da (Scheme S4†). In addition, the use of methacrylate, which has glycidyl, trifluoromethyl, isobornyl, menthyl, and 8-phenylmenthyl groups, resulted in good yields of the desired products 5ga–5ka, respectively. The reaction tolerance to amides was low and produced the corresponding products 5la–5na in 18–24% yields. Notably, the reaction of the substrate with camphorsultum (1l) produced 5la with a 95
:
5 diastereoselectivity. This is due to the high bulkiness of the camphorsultum group, which also gave high selectivity in our previous perfluoroalkylation reactions.10c,f Thereafter, the reactions of more electron-deficient several acrylates (1o–1r) were examined and the corresponding products 5oa–5ra were obtained in 17–32% yields. When the yield was low (5la–5ra), considerable amounts of oxygen-derived by-product (Scheme S2†) was obtained. Furthermore, ethyl 2-phenethyl-propenoate (1s) and itaconic acid diesters (1t and 1u) produced the corresponding products 5sa–5ua in 36–64% yields.
Table 2 Substrate scope of the electron-deficient olefinsa,b
Reaction conditions: 1 (0.25 mmol), 2a (0.75 mmol, 3.0 eq.), 3 (0.025 mmol, 10 mol%), 4 (0.01 mmol, 40 mol%), DIPEA (0.5 mmol, 2.0 eq.), O2 (0.2 mmol, 0.8 eq.), DCE (2.5 mL), at 25 °C, in argon atmosphere, for 24 h, and under white LED irradiation. Isolated yields. |
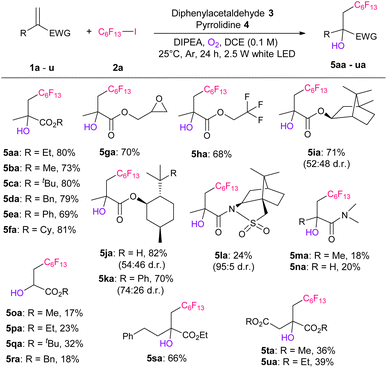 |
Next, we investigated the scope of perfluoroalkyl iodides using 1a (Table 3). Initially, the corresponding hydroxy-perfluoroalkylated products 5aa–5ad were obtained in 76–80% yields. Perfluorobenzyl iodide (2e) can also be used for this reaction. Subsequently, we performed the same reactions using short-chain length perfluoroalkyl iodides (n < 5) and successfully obtained the desired products in good to moderate yields, which were measured using crude 19F NMR (Table S7†). However, because of their high volatility, these products are difficult to isolate via silica gel column chromatography. Therefore, we selected 1d as the substrate, which has a higher boiling point than 1a, and examined the scope of shorter chain length perfluoroalkyl iodides. The desired products 5df–5dj with short liner perfluoroalkylated groups (n = 1–5) were obtained in 71–76% yields. Likewise, bulkier perfluoroisopropyl iodide (2k) and perfluorocyclohexyl iodide (2l) were also tolerated, producing the corresponding products 5dk and 5dl in 64% and 27% yields, respectively. In addition, less nucleophiloc fluorine sources (2m and 2n) with a methylene group next to the iodine atom resulted in products 5dm and 5dn, respectively, in 20–27% yields.
Table 3 Screening of perfluoroalkyl iodidesa,b
Reaction conditions: 1 (0.25 mmol), 2 (0.75 mmol, 3.0 eq.), 3 (0.025 mmol, 10 mol%), 4 (0.01 mmol, 40 mol%), DIPEA (0.5 mmol, 2.0 eq.), O2 (0.2 mmol, 0.8 eq.), DCE (2.5 mL), at 25 °C, in argon atmosphere, for 24 h, and under white LED irradiation. Isolated yields. |
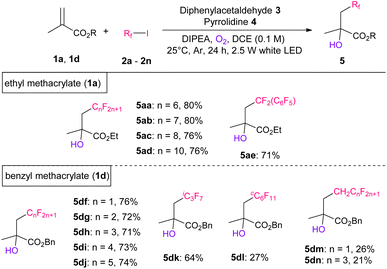 |
We also applied our reactions to various styrenes (Table 4). First, a series of styrenes with electron-donating or electron-withdrawing groups at the p-position (6a–6h) were examined, affording the corresponding hydroxy-perfluoroalkylated products 7aa–7ha in excellent yields (74–89%). In the case of p-nitrostyrene, 6i afforded the desired product 7ia in a 39% yield. Furthermore, o- or m-substituted chlorostyrene (6j or 6k) and 2,3,4,5,6-pentafluorostyrene (6l) gave good yields of products 7ja–7la (63–80%). α-Methyl- or phenyl-substituted styrene (6m–6o) also gave hydroxy-perfluoroalkylated products 7ma–7oa in 69–90% yields. The reaction was then applied to naphthalene substrate 6p, and the desired product 7pa was obtained in 56% yield. In addition, 1,2-dihydronaphthalene (6q) and β-methylstyrene (6r) afforded the corresponding products 7qa and 7ra, respectively, in moderate yields and diastereo-selectivities.
Table 4 Substrate scope of styrenesa,b
Reaction conditions: 6 (0.25 mmol), 2a (0.75 mmol, 3.0 eq.), 3 (0.025 mmol, 10 mol%), 4 (0.01 mmol, 40 mol%), DIPEA (0.5 mmol, 2.0 eq.), O2 (0.2 mmol, 0.8 eq.), DCE (2.5 mL), at 25 °C, in argon atmosphere, for 24 h, and under white LED irradiation. Isolated yields. |
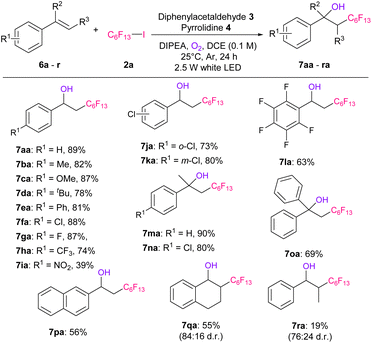 |
To confirm the reaction mechanism, we carried our several control experiments. First, radical trapping experiment for 6a using (2,2,6,6-tetramethylpiperidin-1-yl)oxyl (TEMPO) as radical scavenger was performed (Scheme 2A). As a result, only TEMPO-perfluoroalkylated product 9 was observed, which indicates radical pathway is involved in this reaction. In addition, we conducted labeled experiments using H218O or 18O2 (Scheme 2B). The results suggested that the hydroxy source of this reaction is molecular oxygen. Subsequently, 19F NMR titration experiment, determination of binding stoichiometry, and calculation of association constant (Ka) between 2 and enamine or DIPEA were performed, respectively, to confirm the radical generation mechanism (Fig. S4–S9†).17 As a result, we found that in situ generated enamine would preferentially interact with 2 over DIPEA, and worked effectively as catalyst.
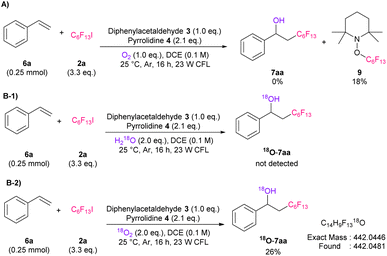 |
| Scheme 2 Control experiments. Yields based on 19F NMR spectroscopy using benzotrifluoride as an internal standard, CFL: compact fluorescent lamps. | |
Based on our previous report14f and other literature,18 we proposed a plausible reaction mechanism (Scheme 3). From the results of entries 4–7 in Table 1 and mechanistic studies, we proposed that both enamine and DIPEA are involved in the perfluoroalkyl radical formation. In a catalytic cycle, enamine was produced by condensation of 3 and 4; then, it generated an EDA complex with 2.14f The results of UV-vis absorption spectra shows the EDA complexation between enamine and 2 (Fig. S10†). After the visible-light irradiation of the EDA complex, perfluoroalkyl radicals, iodide ions, and enamine radical cations were produced. The enamine catalyst was then regenerated via single-electron transfer (SET) from iodine ions,19 and iodine radicals were then reconverted to iodine ions by amine (DIPEA˙+/DIPEA = +0.68 V vs. SCE,20 I2/2I− = +0.54 V (ref. 21)). Finally, HI derived from the iodine ions, formed salt with amine (Fig. S12†).15b Simultaneously, DIPEA was responsible of a halogen bonding interaction with 2 and then perfluoroalkyl radicals were generated by visible-light irradiation.17 Next, the produced perfluoroalkyl radicals attacked the substrate 1 or 6, and the subsequent radical intermediate A was promptly trapped by gaseous oxygen to produce peroxyl intermediate B. Based on the finding that less than 1.0 eq. of oxygen is sufficient for the reaction (Table 1, entry 13), it is assumed that the reaction involves the formation of dimer C, which was formed via the reaction of B and A.18 Finally, the desired product 5 or 7 was produced via hydrogen atom transfer from DIPEA radical cations, as previously reported.18
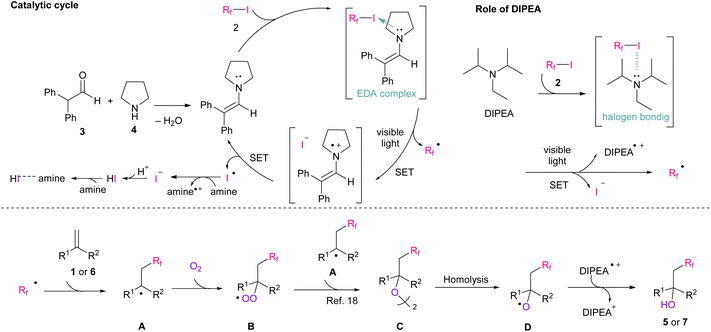 |
| Scheme 3 Proposed mechanism of hydroxy-perfluoroalkylation. | |
Conclusions
In conclusion, we demonstrated the transition metal-free visible-light-induced hydroxy-perfuoroalkylation of electron-deficient conjugated olefins and styrenes using enamine and DIPEA as photo-organocatalysts. This green protocol could be applied to various commercially available substrates and perfluoroalkyl iodides. Further investigations on the reaction mechanism and substrate scope are currently underway in our laboratory.
Conflicts of interest
There are no conflicts to declare.
Acknowledgements
This work was supported by a Grant-in-Aid for Transformative Research Areas (A) Digitalization-Driven Transformative Organic Synthesis (Digi-TOS) (MEXT KAKENHI Grants JP21H05207, and JP21H05219) from MEXT, Grants-in-Aid for Scientific Research (C) (JSPS KAKENHI Grants JP19K05472). We are grateful to Tosoh Finechem Co. for their generous donation of the trifluoromethyl iodide.
Notes and references
-
(a) A. Bondi, J. Phys. Chem., 1964, 68, 441–451 Search PubMed;
(b) B. E. Smart, J. Fluorine Chem., 2001, 109, 3–11 Search PubMed;
(c) D. O'Hagan, Chem. Soc. Rev., 2008, 37, 308–319 Search PubMed;
(d) R. Berger, G. Resnati, P. Metrangolo, E. Weber and J. Hulliger, Chem. Soc. Rev., 2011, 40, 3496–3508 Search PubMed;
(e) N. A. Meanwell, J. Med. Chem., 2018, 61, 5822–5880 Search PubMed.
- For selected reviews, see:
(a) C. Isanbor and D. O'Hagan, J. Fluorine Chem., 2006, 127, 303–319 Search PubMed;
(b) K. L. Kirk, J. Fluorine Chem., 2006, 127, 1013–1029 Search PubMed;
(c) S. Purser, P. R. Moore, S. Swallow and V. Gouverneur, Chem. Soc. Rev., 2008, 37, 320–330 Search PubMed;
(d) W. K. Hagmann, J. Med. Chem., 2008, 51, 4359–4369 Search PubMed;
(e) J. Wang, M. Sánchez-Roselló, J. L. Aceña, C. del Pozo, A. E. Sorochinsky, S. Fustero, V. A. Soloshonok and H. Liu, Chem. Rev., 2014, 114, 2432–2506 Search PubMed;
(f) Y. Zhou, J. Wang, Z. Gu, S. Wang, W. Zhu, J. L. Aceña, V. A. Soloshonok, K. Izawa and H. Liu, Chem. Rev., 2016, 116, 422–518 Search PubMed;
(g) M. Inoue, Y. Sumii and N. Shibata, ACS Omega, 2020, 5, 10633–10640 Search PubMed.
- For selected reviews, see:
(a) P. Jeschke, ChemBioChem, 2004, 5, 570–589 Search PubMed;
(b) T. Fujiwara and D. O'Hagan, J. Fluorine Chem., 2014, 167, 16–29 Search PubMed;
(c) Y. Ogawa, E. Tokunaga, O. Kobayashi, K. Hirai and N. Shibata, iScience, 2020, 23, 101467–101520 Search PubMed.
- For selected example and review, see:
(a) D. M. Walba, H. A. Razavi, N. A. Clark and D. S. Parmar, J. Am. Chem. Soc., 1988, 110, 8686–8691 Search PubMed;
(b) M. Hird, Chem. Soc. Rev., 2007, 36, 2070–2095 Search PubMed.
- For recent reviews of mono-fluorinations, see:
(a) P. A. Champagne, J. Desroches, J.-D. Hamel, M. Vandamme and J.-F. Paquin, Chem. Rev., 2015, 115, 9073–9174 Search PubMed;
(b) B. Lantaño and A. Postigo, Org. Biomol. Chem., 2017, 15, 9954–9973 Search PubMed;
(c) Y. Zhu, J. Han, J. Wang, N. Shibata, M. Sodeoka, V. A. Soloshonok, J. A. S. Coelho and F. D. Toste, Chem. Rev., 2018, 118, 3887–3964 Search PubMed;
(d) R. Szpera, D. F. J. Moseley, L. B. Smith, A. J. Sterling and V. Gouverneur, Angew. Chem., Int. Ed., 2019, 58, 14824–14848 Search PubMed.
- For recent reviews of fluoroalkylations, see:
(a) J. A. Ma and D. Cahard, J. Fluorine Chem., 2007, 128, 975–996 Search PubMed;
(b) J. Hu, W. Zhang and F. Wang, Chem. Commun., 2009, 7465–7478 Search PubMed;
(c) G. Landelle, M. Bergeron, M.-O. Turcotte-Savard and J.-F. Paquin, Chem. Soc. Rev., 2011, 40, 2867–2908 Search PubMed;
(d) S. Barata-Vallejo, M. R. Torviso, B. Lantaño, S. M. Bonesi and A. Postigo, J. Fluorine Chem., 2014, 161, 134–141 Search PubMed;
(e) S. Barata-Vallejo, B. Lantaño and A. Postigo, Chem.–Eur. J., 2014, 20, 16806–16829 Search PubMed;
(f) S. Barata-Vallejo, S. M. Bonesi and A. Postigo, RSC Adv., 2015, 5, 62498–62518 Search PubMed;
(g) C. Ni and J. Hu, Chem. Soc. Rev., 2016, 45, 5441–5454 Search PubMed;
(h) H.-X. Song, Q.-Y. Han, C.-L. Zhao and C.-P. Zhang, Green Chem., 2018, 20, 1662–1731 Search PubMed;
(i) Z. Feng, Y.-L. Xiao and X. Zhang, Acc. Chem. Res., 2018, 51, 2264–2278 Search PubMed.
- For selected reviews of radical protocols, see:
(a) C. P. Jasperse, D. P. Curran and T. L. Fevig, Chem. Rev., 1991, 91, 1237–1286 Search PubMed;
(b) J. Iqbal, B. Bhatia and N. K. Nayyar, Chem. Rev., 1994, 94, 519–564 Search PubMed;
(c) W. R. Dolbier, Chem. Rev., 1996, 96, 1557–1584 Search PubMed;
(d) A. Studer, Angew. Chem., Int. Ed., 2012, 51, 8950–8958 Search PubMed;
(e) U. Wille, Chem. Rev., 2013, 113, 813–853 Search PubMed;
(f) S. Barata-Vallejo, M. V. Cooke and A. Postigo, ACS Catal., 2018, 8, 7287–7307 Search PubMed.
- For selected reviews of perfluoroalkylation using perfluoroalkyl iodide, see:
(a) N. O. Brace, J. Fluorine Chem., 1999, 93, 1–25 Search PubMed;
(b) N. O. Brace, J. Fluorine Chem., 1999, 96, 101–127 Search PubMed;
(c) N. O. Brace, J. Fluorine Chem., 2001, 108, 147–175 Search PubMed.
- For selected reviews of perfluoroalkylation for electron-rich olefins, see:
(a) E. Merino and C. Nevado, Chem. Soc. Rev., 2014, 43, 6598–6608 Search PubMed;
(b) H. Egami and M. Sodeoka, Angew. Chem., Int. Ed., 2014, 53, 8294–8308 Search PubMed;
(c) T. Besset, T. Poisson and X. Pannecoucke, Eur. J. Org. Chem., 2015, 2765–2789 Search PubMed;
(d) P. Gao, X.-R. Song, X.-Y. Liu and Y.-M. Liang, Chem.–Eur. J., 2015, 21, 7648–7661 Search PubMed.
- For selected examples of perfluoalkylation for electron-deficient olefins, see:
(a) Z.-M. Qiu and D. J. Burton, J. Org. Chem., 1995, 60, 3465–3472 Search PubMed;
(b) T. Yajima, C. Saito and H. Nagano, Tetrahedron, 2005, 61, 10203–10215 Search PubMed;
(c) T. Yajima and H. Nagano, Org. Lett., 2007, 9, 2513–2515 Search PubMed;
(d) E. Yoshioka, S. Kohtani, K. Sawai, Kentefu, E. Tanaka and H. Miyabe, J. Org. Chem., 2012, 77, 8588–8604 Search PubMed;
(e) T. Yajima, I. Jahan, T. Tonoi, M. Shinmen, A. Nishikawa, K. Yamaguchi, I. Sekine and H. Nagano, Tetrahedron, 2012, 68, 6856–6861 Search PubMed;
(f) T. Yajima, K. Yamaguchi, R. Hirokane and E. Nogami, J. Fluorine Chem., 2013, 150, 1–7 Search PubMed;
(g) X.-J. Tang and W. R. Dolbier Jr, Angew. Chem., Int. Ed., 2015, 54, 4246–4249 Search PubMed;
(h) E. Yoshioka, S. Kohtani, E. Tanaka, Y. Hata and H. Miyabe, Tetrahedron, 2015, 71, 773–781 Search PubMed;
(i) S.-H. Zhou, J.-H. Lin, G. Zhao, J.-C. Xiao and W.-G. Cao, RSC Adv., 2016, 6, 60080–60083 Search PubMed.
- For selected reviews of photoredox catalyst, see:
(a) T. P. Yoon, M. A. Ischay and J. Du, Nat. Chem., 2010, 2, 527–532 Search PubMed;
(b) J. M. R. Narayanam and C. R. J. Stephenson, Chem. Soc. Rev., 2011, 40, 102–113 Search PubMed;
(c) D. M. Schultz and T. P. Yoon, Science, 2014, 343, 1239176 Search PubMed;
(d) K. L. Skubi, T. R. Blum and T. P. Yoon, Chem. Rev., 2016, 116, 10035–10074 Search PubMed;
(e) N. A. Romero and D. A. Nicewicz, Chem. Rev., 2016, 116, 10075–10166 Search PubMed;
(f) L. Marzo, S. K. Pagire, O. Reiser and B. König, Angew. Chem., Int. Ed., 2018, 57, 10034–10072 Search PubMed;
(g) S. P. Pitre and L. E. Overman, Chem. Rev., 2022, 122, 1717–1751 Search PubMed.
- For selected reviews of photocatalysed perfluoroalkylation see:
(a) T. Koike and M. Akita, Top. Catal., 2014, 57, 967–974 Search PubMed;
(b) X. Pan, H. Xia and J. Wu, Org. Chem. Front., 2016, 3, 1163–1185 Search PubMed;
(c) T. Koike and M. Akita, Acc. Chem. Res., 2016, 49, 1937–1945 Search PubMed;
(d) T. Chatterjee, N. Iqbal, Y. You and E. J. Cho, Acc. Chem. Res., 2016, 49, 2284–2294 Search PubMed . For selected examples for photocatalysed perfluoroalkylation see: ;
(e) D. A. Nagib, M. E. Scott and D. W. C. MacMillan, J. Am. Chem. Soc., 2009, 131, 10875–10877 Search PubMed;
(f) P. V. Pham, D. A. Nagib and D. W. C. MacMillan, Angew. Chem., Int. Ed., 2011, 50, 6119–6122 Search PubMed;
(g) D. A. Nagib and D. W. C. MacMillan, Nature, 2011, 480, 224–228 Search PubMed;
(h) C.-J. Wallentin, J. D. Nguyen, P. Finkbeiner and C. R. J. Stephenson, J. Am. Chem. Soc., 2012, 134, 8875–8884 Search PubMed;
(i) Y. Yasu, T. Koike and M. Akita, Angew. Chem., Int. Ed., 2012, 51, 9567–9571 Search PubMed;
(j) N. Iqbal, S. Choi, E. Ko and E. J. Cho, Tetrahedron Lett., 2012, 53, 2005–2008 Search PubMed;
(k) Y. Yasu, T. Koike and M. Akita, Chem. Commun., 2013, 49, 2037–2039 Search PubMed;
(l) N. Iqbal, J. Jung, S. Park and E. J. Cho, Angew. Chem., Int. Ed., 2014, 53, 539–542 Search PubMed;
(m) M. Daniel, G. Dagousset, P. Diter, P.-A. Klein, B. Tuccio, A.-M. Goncalves, G. Masson and E. Magnier, Angew. Chem., Int. Ed., 2017, 56, 3997–4001 Search PubMed.
- For selected examples of perfluoroalkylation using organic dye, see:
(a) D. J. Wilger, N. J. Gesmundo and D. A. Nicewicz, Chem. Sci., 2013, 4, 3160–3165 Search PubMed;
(b) L. Cui, Y. Matusaki, N. Tada, T. Miura, B. Uno and A. Itoh, Adv. Synth. Catal., 2013, 355, 2203–2207 Search PubMed;
(c) S. P. Pitre, C. D. McTiernan, H. Ismaili and J. C. Scaiano, ACS Catal., 2014, 4, 2530–2535 Search PubMed;
(d) T. Yajima and M. Ikegami, Eur. J. Org. Chem., 2017, 2126–2129 Search PubMed;
(e) G.-R. Park, Y. Choi, M. G. Choi, S.-K. Chang and E. J. Cho, Asian J. Org. Chem., 2017, 6, 436–440 Search PubMed;
(f) N. Noto, T. Koike and M. Akita, Chem. Sci., 2017, 8, 6375–6379 Search PubMed;
(g) T. Yajima and S. Shigenaga, Org. Lett., 2019, 21, 138–141 Search PubMed.
- For selected examples of perfluoroalkylation via the formation of EDA complex, see:
(a) M. Nappi, G. Bergonzini and P. Melchiorre, Angew. Chem., Int. Ed., 2014, 53, 4921–4925 Search PubMed;
(b) Ł. Wozniak, J. J. Murphy and P. Melchiorre, J. Am. Chem. Soc., 2015, 137, 5678–5681 Search PubMed;
(c) Y. Cheng and S. Yu, Org. Lett., 2016, 18, 2962–2965 Search PubMed;
(d) H. Matsui, M. Murase and T. Yajima, Org. Bimol. Chem., 2018, 16, 7120–7123 Search PubMed;
(e) L. Helmecke, M. Spittler, K. Baumgarten and C. Czekelius, Org. Lett., 2019, 21, 7823–7827 Search PubMed;
(f) T. Yajima, M. Murase and Y. Ofuji, Eur. J. Org. Chem., 2020, 3808–3811 Search PubMed.
-
(a) J. Cao, G. Wang, L. Gao, H. Chen, X. Liu, X. Cheng and S. Li, Chem. Sci., 2019, 10, 2767–2772 Search PubMed;
(b) Y. Shen, N. Lei, C. Lu, D. Xi, X. Geng, P. Tao, Z. Su and K. Zheng, Chem. Sci., 2021, 12, 15399–15406 Search PubMed.
- Y. Xiao, Y.-K. Chun, S.-C. Cheng, C.-O. Ng, M.-K. Tse, N.-Y. Lei, R. Liu and C.-C. Ko, Catal. Sci. Technol., 2021, 11, 556–562 Search PubMed.
-
(a) X. Sun, W. Wang, Y. Li, J. Ma and S. Yu, Org. Lett., 2016, 18, 4638–4641 Search PubMed;
(b) Y. Wang, J. Wang, G.-X. Li, G. He and G. Chen, Org. Lett., 2017, 19, 1442–1445 Search PubMed;
(c) X. Tang and A. Studer, Chem. Sci., 2017, 8, 6888–6892 Search PubMed;
(d) T. Chen, Y. Guo, K. Sun, L.-Z. Wu, W.-Q. Liu, C. Liu, Y. Huang and Q.-Y. Chen, Org. Chem. Front., 2018, 5, 1045–1048 Search PubMed;
(e) D. Zheng and A. Studer, Org. Lett., 2019, 21, 325–329 Search PubMed;
(f) K. Jana, I. Mizota and A. Studer, Org. Lett., 2021, 23, 1280–1284 Search PubMed.
-
(a) Q. Lu, C. Liu, Z. Huang, Y. Ma, J. Zhang and A. Lei, Chem. Commun., 2014, 50, 14101–14104 Search PubMed;
(b) B. Sun, R. Zhu, X. Zhuang, X. Shi, P. Huang, Z. Yan, C. Yu and C. Jin, Org. Lett., 2021, 23, 617–622 Search PubMed;
(c) Z.-Z. Xie, Y. Zheng, K. Tang, J.-P. Guan, C.-P. Yuan, J.-A. Xiao, H.-Y. Xiang, K. Chen, X.-Q. Chen and H. Yang, Org. Lett., 2021, 23, 9474–9479 Search PubMed.
- Y. Li, D. Wang, L. Zhang and S. Luo, J. Org. Chem., 2019, 84, 12071–12090 Search PubMed.
-
(a) U. Pischel, X. Zhang, B. Hellrung, E. Haselbach, P.-A. Muller and W. A. Nau, J. Am. Chem. Soc., 2000, 122, 2027–2034 Search PubMed;
(b) E. Yoshioka, S. Kohtani, T. Jichu, T. Fukazawa, T. Nagai, A. Kawashima, Y. Takemoto and H. Miyabe, J. Org. Chem., 2016, 81, 7217–7229 Search PubMed.
- Handbook of Chemistry: Pure Chemistry, The Chemical Society of Japan, Tokyo, 3rd edn, 1984, ch. 12, p. 475 Search PubMed.
|
This journal is © The Royal Society of Chemistry 2022 |
Click here to see how this site uses Cookies. View our privacy policy here.