DOI:
10.1039/D2RA06637H
(Paper)
RSC Adv., 2022,
12, 36126-36137
Room temperature dilute magnetic semiconductor response in (Gd, Co) co-doped ZnO for efficient spintronics applications
Received
20th October 2022
, Accepted 5th December 2022
First published on 19th December 2022
Abstract
The co-precipitation approach was utilized to experimentally synthesize ZnO, Zn0.96Gd0.04O and Zn0.96−xGd0.04CoxO (Co = 0, 0.01, 0.03, 0.04) diluted magnetic semiconductor nanotubes. The influence of gadolinium and cobalt doping on the microstructure, morphology, and optical characteristics of ZnO was investigated, and the Gd doping and Co co-doping of the host ZnO was verified by XRD and EDX. The structural investigation revealed that the addition of gadolinium and cobalt to ZnO reduced crystallinity while maintaining the preferred orientation. The SEM study uncovered that the gadolinium and cobalt dopants did not affect the morphology of the produced nanotubes, which is further confirmed through TEM. In the UV-vis spectra, no defect-related absorption peaks were found. By raising the co-doping content, the crystalline phase of the doped samples was enhanced. It was discovered that the dielectric response and the a.c. electrical conductivity display a significant dependent relationship. With the decreasing frequency and increasing Co co-dopant concentration, the εr and ε′′ values decreased. It was also discovered that the εr, ε′′, and a.c. electrical conductivity increased when doping was present. Above room temperature, co-doped ZnO nanotubes exhibited ferromagnetic properties. The ferromagnetic behaviour increased as Gd (0.03) doping increased. Increasing the Co content decreased the ferromagnetic behaviour. It was observed that Zn0.96−xGd0.04CoxO (x = 0.03) nanotubes exhibit superior electrical conductivity, magnetic and dielectric characteristics compared to pure ZnO. This high ferromagnetism is typically a result of a magnetic semiconductor that has been diluted. In addition, these nanoparticles are utilized to design spintronic-based applications in the form of thin-films.
1. Introduction
The research work on oxide semiconductors doped with transition metals (TMs)1–8 has attracted many researchers, focusing on diluted magnetic semiconductor (DMS)-based compounds for spintronics-related applications. The discovery of ferromagnetism (FM) in Ga1−xMnxAs allowed much progress in DMS for electronic devices related to spin (spintronics)9 including the quantum Hall effect, single electronic charging, resistive switching devices, and semiconductor lasers. Moreover, a large number of technological applications such as photonics, biosensors, nanoelectronics, light-emitting devices (LED), optoelectronics, and spin-based devices have been commercialized.10–15 These devices are interesting in that there is the possibility of realizing a DMS Curie temperature (TC) above room temperature ferromagnetism (RTFM). Many previous studies13,16–18 used TMs doped with II–VI and III–V semiconductors to create a FM phase in the host ZnO. This behavior is welcome in many real-life applications and has caused some controversy about the origin of FM. Many materials with non-magnetic ions have exhibited unexpected FM behaviour11,18,19 provoking interest to further research the RTFM of these structures. This is a new study for spin-based electronics. Spin-based electronics can be used for devices specially RRAM and hard drives.
DMS in II–VI semiconductor materials emanates from the introduction of TMs with 3d electrons in the lattice.13 Among these, Co-doped ZnO has interesting properties.7,20 Because cobalt (Co) is one of the most soluble transition metal ions in ZnO. In addition, it has the same ionic radius as the zinc (Zn) ion, which is favorable for doping at the Zn2+ site. ZnO can grow into many different shapes, such as spheres and dumbbells, rods, nanonails, hollow nanospheres, rods that look like pyramids or laths, flowers, and nanowires. For DMS to work well in industrial settings, its size and shape are very important. Nanostructures can change shape from top to bottom by doping, but it's hard to control the shape. The shape, size, structure, and optical properties are all affected by the amount of doping, how long it is heated, and how hot it grows. The level of TM ions substitution determines the ferromagnetic behaviour. The first RTFM in Mn-doped ZnO nanoparticles was recently reported by Hao et al.21 Oshio et al.22 later studied leakage current in the epitaxial film using this material. Recently, the behaviour of semiconductor quantum dots and Co–ZnO nanoparticles RTFM has been studied.23 It was demonstrated that Co doping caused defects and oxygen vacancies. This was demonstrated by an increase in luminescence intensity in the visible region of the PL spectra, indicating that the defects and vacancies were caused by Co doping. Controlling defects, in addition to shape and size, is critical for the application of ZnO because imperfections can alter the material's properties. The RTFM properties in Fe-doped ZnO nanoparticles were also reported by Xu et al.24 The TMs-ZnO dielectric and electrical properties are also required in high-frequency electronic devices.25–27 Despite many experimental and theoretical reports on ZnO, the work on doped TMs is still controversial.
In this paper, we report the synthesis of ZnO, Zn0.96Gd0.04O and Zn0.96−xGd0.04CoxO (Co = 0, 0.01, 0.03, 0.04) nanotubes (NTs) by co-precipitation method with varying concentrations of Co while keeping the Gd content at a fixed concentration. The changing in the concentration of Co altered the crystallinity and indicated a hindrance in the recombination rate of photoinduced electron–hole pairs in ZnO. This enhanced dielectric and magnetic response of zinc NTs.
2. Materials and methods
2.1 Chemicals
The reagents, zinc chloride [ZnCl2·6H2O], gadolinium chloride [GdCl3·6H2O], and cobalt chloride [CoCl2·4H2O] were purchased from Alfa-Aesar and used as received.
2.2 Sample preparation
The (Gd, Co) co-doped ZnO specimens were produced in fewer mounts by using the co-precipitation method. The procedure was to dissolve zinc chloride [ZnCl2·6H2O], in 40 ml pure water before the dropwise addition of 24 ml of aqueous ammonia solution (1.7 M) and urea with strong stirring whilst pH is controlled between 1.0 and 10.2.28,29 Finally, brownish-type powder was accumulated by washing and centrifugation after the reaction. They were then dried at 70 °C for 28 h before annealing at 500 °C for 3 h in a furnace. For the Gd and Co co-doped specimens, zinc, gadolinium, and cobalt acetate were slowly dripped whilst stirring before separating the precipitates.
2.3 Instruments
The Cu Kα radiation (λ = 1.5406 Å) from XRD was employed for structural characterization. The High Score Plus software was used for the Rietveld refinements to determine the specimen's lattice parameters and volumes. A field emission scanning electron microscope was utilized to examine the particle shapes (FE-SEM). Transmission electron microscopy was utilized to determine the nature of the particles. The UV-vis spectrometer was utilized to examine the optical absorption spectrum. In the frequency range of 40 Hz to 5 MHz, dielectric properties and electrical conductivity were measured using the impedance analyzer from the frequency range of 40 Hz to 1 MHz. A Quantum Design superconducting quantum interface device (SQUID) was used to measure diluted magnetic semiconductor properties.
3. Results and discussions
3.1 Structural properties
The XRD result of ZnO, Zn0.96Gd0.04O and Zn0.96−xGd0.04CoxO (Co = 0, 0.01, 0.03, 0.04) NTs, are displayed in Fig. 1. In all the specimens, no impurities phase was detected and crystallized with the hexagonal structure (wurtzite-type P63mc space group). All the lattice constants of a = 3.2497 Å, c = 5.1974 Å and a = 3.2457 Å, c = 5.1914 Å were acquired by Rietveld refinement software along with weighted profile factor (RWP = 9.38%, 9.37 and the goodness-of-fit χ2 = 2.571, 2.581) for the pure ZnO and Zn0.96Gd0.04O as shown in Fig. 1(a) and (b). The unit cell volume for pure ZnO is V = 47.45 Å3 and it is decreased to V = 47.40 Å3 for Zn0.96Gd0.04O specimen (see Fig. 1(b)). For Zn0.93Gd0.04Co0.03O, the unit cell volume increased to V = 47.28 Å3, which is higher when compared to pure and Zn0.96Gd0.04O. Also, the internal parameter u, which is the length of the bond along the c-axis, is not the same as the value for the ideal wurtzite structure (0.375), as expected for real crystals. Also, the internal parameter goes up as x goes up (as mentioned in Table 1) without keeping an inverse correlation with the c/a ratio, as commonly reported for ZnO.30 It is anticipated since the ionic radius of Gd3+ (94 Å), and Co2+ (0.65 Å) are larger than that of Zn2+ (0.60 Å). This behaviour can be explained by the fact that Gd3+ and Co2+ ions replace Zn2+ ions in the structure of wurtzite. This is because gadolinium and cobalt ions have larger ionic radii and less electronegativity than zinc ions.1,23,26 Also, the XRD results support the idea that Gd3+ and Co2+ ions were put into the wurtzite structure, likely replacing Zn ions. This caused the wurtzite unit cell to change shape. Also, the XRD patterns reveal that the Gd (fixed) and Co co-dopants in ZnO increase their concentrations, the peaks shifted at a lower angle, as displayed in Fig. 1(b). It is recommended that specimens are transferred to a disordered form as a result of Gd and Co doping.
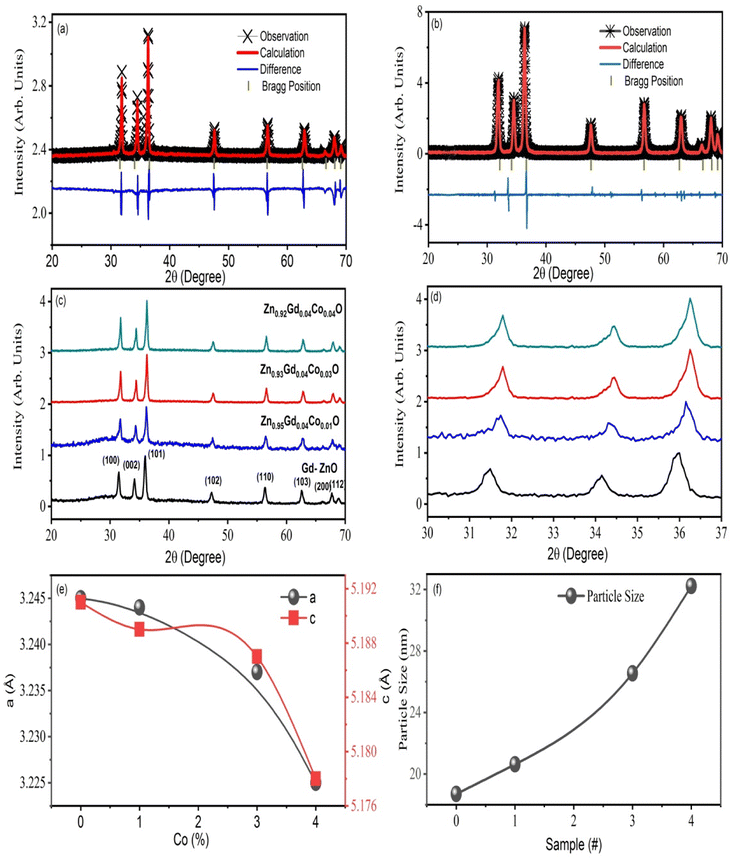 |
| Fig. 1 (a) and (b) The Rietveld refinement of ZnO and Zn0.96Gd0.04O (c) XRD for all the samples. (d) The peak shifting with different doping (e) lattice parameters calculated from XRDs, and (f) the particle size of single doped and Zn0.96−xGd0.04CoxO (Co = 0, 0.01, 0.03, 0.04) samples. | |
Table 1 The variation of structure parameters was calculated from ZnO and Zn0.96−xGd0.04CoxO (Co = 0, 0.01, 0.03, 0.04) samples
Sample |
hkl |
d-Spacing (Å) |
2θ (°) |
Grain size (nm) |
Lattice constant |
c/a |
u |
Unite cell volume (Å3) |
a (Å) |
c (Å) |
ZnO |
100 |
2.7973 |
31.832 |
14.34 |
3.2497 |
5.1874 |
1.596 |
0.388 |
47.45 |
002 |
2.5972 |
34.423 |
13.11 |
— |
— |
|
— |
101 |
2.4704 |
36.344 |
11.45 |
— |
— |
|
— |
Zn0.96Gd0.04O |
100 |
2.8079 |
31.847 |
13.54 |
3.2457 |
5.1914 |
1.599 |
0.392 |
47.40 |
002 |
2.5973 |
34.645 |
13.61 |
— |
— |
|
— |
101 |
2.4715 |
36.465 |
12.34 |
— |
— |
|
— |
Zn0.95Gd0.04Co0.01O |
100 |
2.8082 |
31.902 |
12.32 |
3.244 |
5.189 |
1.599 |
0.395 |
47.37 |
002 |
2.5976 |
34.670 |
13.35 |
— |
— |
|
— |
101 |
2.4719 |
36.490 |
14.01 |
— |
— |
|
— |
Zn0.93Gd0.04Co0.03O |
100 |
2.8085 |
36.498 |
14.75 |
3.237 |
5.187 |
1.602 |
0.399 |
47.28 |
002 |
2.5980 |
34.689 |
15.54 |
— |
— |
|
— |
101 |
2.4721 |
36.512 |
16.4 |
— |
— |
|
— |
Zn0.92Gd0.04Co0.04O |
100 |
2.8088 |
36.459 |
|
3.225 |
5.178 |
1.605 |
0.401 |
47.21 |
002 |
2.5984 |
34.692 |
|
|
|
|
|
101 |
2.4727 |
36.534 |
|
|
|
|
|
The XRD peaks broadening were used for the Scherrer formula to determine the crystallite size of specimens,27,31 and it was found that size decreased with an increase in Co content from 0 to 4%. Such a decrease can be attributed to grain growth inhibition caused by the presence of Gd and Co in ZnO. This shows that the presence Co in high amounts does not only provide O2 vacancies to smooth densification to support and inhibits grain development. However, grain boundary segregation in highly doped specimens causes a reduction in particle size. The decrease in lattice parameter with a higher in Co content is because of the lower ionic radius of Co (70 pm) when compared to Zn (74 pm) ions. Table 1 summarizes the calculated X-ray density, grains size, lattice parameters, rain size, and V.
The compositions (wt%) of Zn, O, Gd and Co obtained are given by the EDX spectra in Fig. 2(a)–(c). As anticipated, Zn and O are the predominant chemical components in pure ZnO, but Gd and Co co-doped ZnO specimens exhibit Gd and Co peaks. It was discovered that the weight percent of doped TMs was close to the prescribed quantity used for sample preparation as displayed in the inset of Fig. 2(a)–(c). Fig. 2(a)–(c) displays the morphology and elemental analysis of pure, 3 and 4 wt% Gd and Co co-doped ZnO examined by SEM analysis. These results indicate that the NTs have same morphology with hexagonal wurtzite structure. The form of the samples tube-like structure with increasing aggregation as Gd and Co content increases.
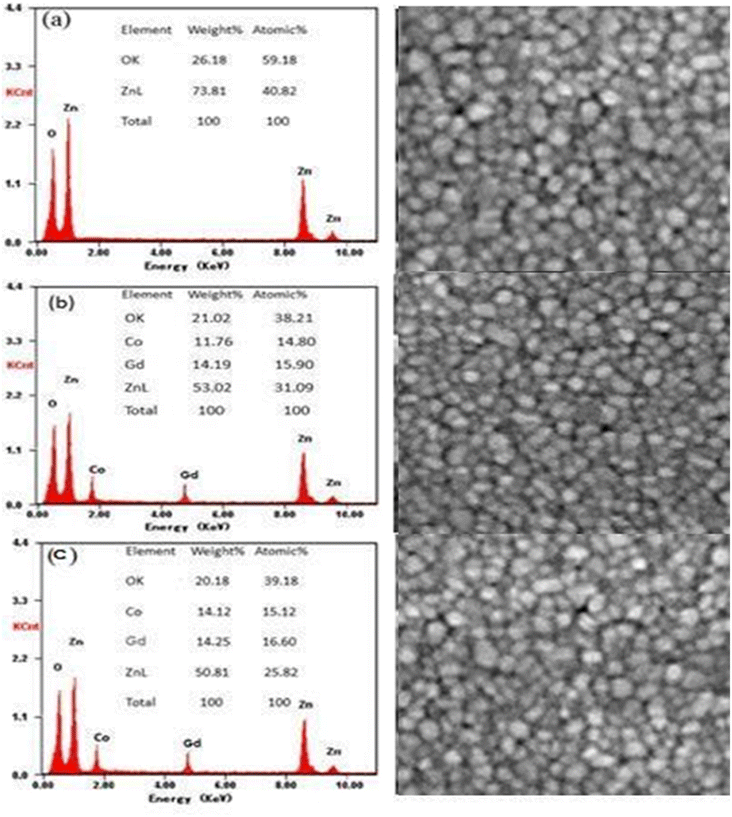 |
| Fig. 2 EDX images of (a) ZnO, (b) Zn0.93Gd0.04Co0.03O, (c) Zn0.92Gd0.04Co0.04O and their corresponding SEM images. | |
The transmission electron microscopy (TEM) images of ZnO, Zn0.93Gd0.04Co0.03O, and Zn0.92Gd0.04Co0.04O NTs are displayed in Fig. 3(a)–(c). The TEM images for ZnO, Zn0.93Gd0.04Co0.03O and Zn0.92Gd0.04Co0.04O NTs displayed almost tube-like shaped with a uniform distribution. The TEM study indicates that the mean tube size for pure ZnO is approximately 15 nm, 17 for Zn0.93Gd0.04Co0.03O and 19 nm for Zn0.92Gd0.04Co0.04O. The co-doping with Gd and Co enhances the tube size, which is consistent with the XRD findings. Fig. 3(a)–(c) middle panel displays selected area electron diffraction (SEAD) images of the synthesized NTs. The fringes achieved in the SAED image for the ZnO sample indicate the formation of the polycrystalline tetragonal structure of ZnO. It is also essential to note that the broad XRD peak of Gd and Co-doped ZnO suggests small crystallite size and, consequently, a significant surface area. It is evident from the TEM micrograph that the size of Gd–Co co-doped ZnO is greater than that of pure ZnO, implying that the surface area of Gd–Co co-doped ZnO is greater than that of pure ZnO. A larger surface area of the sample is required for enhanced photodegradation efficiency.
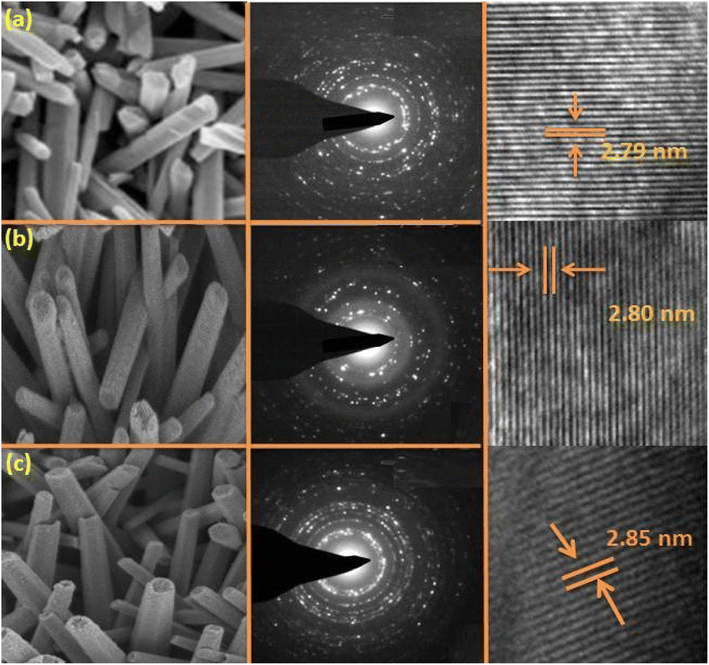 |
| Fig. 3 TEM images of (a) ZnO, (b) Zn0.93Gd0.04Co0.03O, (c) Zn0.92Gd0.04Co0.04O, their corresponding SEAD images (med panels) and HRTEM micrographs. | |
Fig. 4 depicts the pure FT-IR, the pure, 0 wt%, 1 wt%, 3 wt%, and 4 wt% Co-doped ZnO NTs. All samples had predominant absorption bands at 3507, 1650, 1115, 995, 788, and 614 cm−1. The absorption spectra at 614 cm−1 corresponds to the Zn–O range. The absorbent band at 3507 cm−1 corresponds to the OH vibration of water. The band around 1650 cm−1 is caused by C
O stretching. Band of absorption detected at 2350 cm−1 resembles CO2 molecules in the air. In contrast, the absorption band at around 995 cm−1 indicates the shoulder of resonance interaction between modes of pulsation of oxide ions in nano-crystals with asymmetric stretching. The bands that occur at around 788 cm−1 and 1115 cm−1 are due to the stretching of Zn–O–Zn and C–O. The band at 3507 cm−1 in the FTIR spectrum of the Co-doped ZnO sample corresponds to the –OH mode in H2O molecules. The presence of these manufactured NPs can be attributed to the atmospheric absorption of water molecules. The 995 cm−1 band consists of a shoulder with unequal stretching resonance contact between the vibrational modes of oxide ions in nano-crystals. The ZnO surface quickly absorbs the acetate and hydroxyl assemblies by drying them at temperatures exceeding 600 °C, consistent with the XRD output result, the FTIR result demonstrates that Co occupies the Zn site in the ZnO grid, as the zero peak in the spectrum is identical to that of Co.
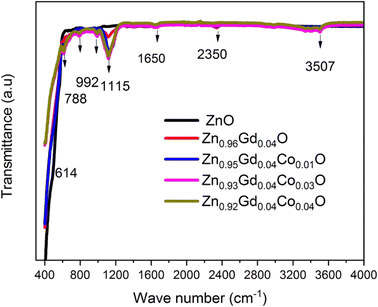 |
| Fig. 4 The FT-IR pattern of pure, 0 wt%, 1 wt%, 3 wt%, and 4 wt% Co-doped ZnO NTs. | |
All samples were investigated for their optical properties using UV-vis spectroscopy, as shown in Fig. 5. Fig. 5(a) depicts the UV-vis absorption spectra of ZnO and Zn0.96−xGd0.04CoxO (Co = 0.01, 0.03, and 0.04) NTs at room temperature and within the wavelength range of 300–900 nm. Compared to ZnO NTs, Zn0.96−xGd0.04CoxO (Co = 0.01, 0.03, 0.04) NTs exhibit prominent visible absorption peaks. As the concentration of dopant increases, the absorption peaks of ZnO and Zn0.96−xGd0.04CoxO (Co = 0.01, 0.03, and 0.04) shift to wavelengths 370, 380–410 nm. The redshift in the absorption peaks suggests that the sizes of NTs enhance as the concentration of dopant rises. The mean crystallinity rises as a result of disorder and impurities introduced into the ZnO crystal structure by the dopant.32–35 Using ellipsometry and optical analysis, the electronic transitions of dopant ions were analysed over a broad spectral range (380–410 nm).31,36 In samples, the electronic transition occurred due to the presence of dopant ions (Gd3+ and Co2+) in a tetrahedral.37–40 Through the wurtzite structure (P63/mmc space group) of ZnO, tetrahedrally coordinated O2− and Zn2+ ions are formed. Dopant ions (Gd3+ and Co2+) replace host ions (Zn2+) in the absorption spectrum of the visible spectrum. Tauc relation was used to calculate the optical band gap (Eg) of ZnO and Zn0.96−xGd0.04CoxO (Co = 0.01, 0.03, 0.04).41
where
α is the coefficient of optical absorption,
B is a constant and
hν is the energy of the incident photon. For ZnO,
n is 1/2 because it has direct bandgap.
Fig. 5(b)–(e) depicts the extrapolated bandgap curves for ZnO and Zn
0.96−xGd
0.04Co
xO (Co = 0.01, 0.03, 0.04) NTs. In
Fig. 4(b) shows that the ZnO NTs have 3.43 eV bandgap that is bigger than the bulk. The bandgap of continuously decreases from 3.45 eV to 3.15 eV with increase in doping concentration (Gd = 4% and Co = 1, 3 and 4%) which are shown in
Fig. 5(b)–(e).
42,43 Doping decreased the bandgap of ZnO NTs because an exchange transition between the s–d and p–d electronic states occurred. Bylsma
et al., provide an explanation for the narrowing of
Eg due to the sp–d exchange interaction effect.
44 The decrease in energy band gap is a result of an increase in the surface-to-volume ratio and an increase in the number of defects.
45,46 Redshift enhancement and bandgap reduction indicate the presence of Gd/Co dopant in the ZnO lattice.
47
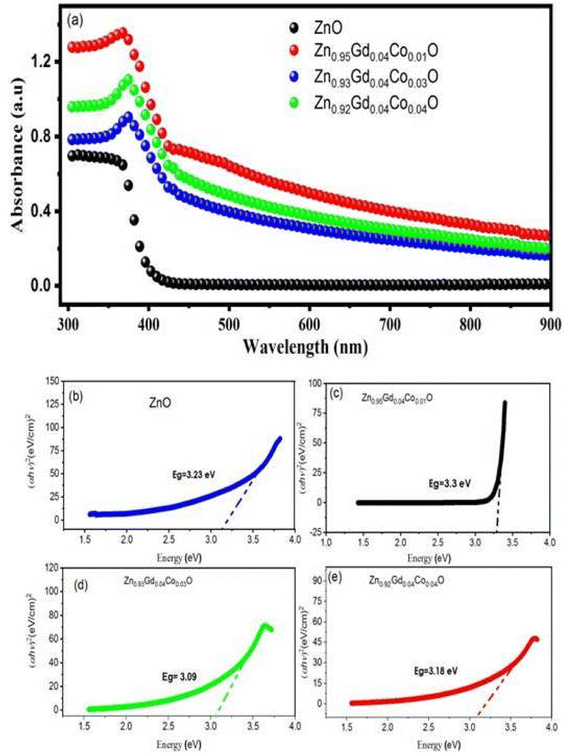 |
| Fig. 5 (a) UV-vis spectroscopy ZnO, and Zn0.96−xGd0.04CoxO (Co = 0.01, 0.03, 0.04) NTs. (b)–(e) Calculation of energy band gap using Tauc's plot method for all samples of ZnO, and Zn0.96−xGd0.04CoxO (Co = 0.01, 0.03, 0.04). | |
3.2 Dielectric properties
3.2.1 Dielectric constant. Fig. 6(a) shows the frequency (f) dependence of dielectric constant (εr) for all the specimens annealed at 500 °C. The specimens were prepared by pressing them into circular pellets of 8 mm diameter using a presser before measuring their capacitance, dielectric loss, and a.c. conductivity. The capacitance was measured using gold glue electrodes following the approach used in ref. 3 and 33. The below relation was used to determine the εrwhere C represents capacitance, d represents the height of the cylinder, A is the pellet cross-sectional area.
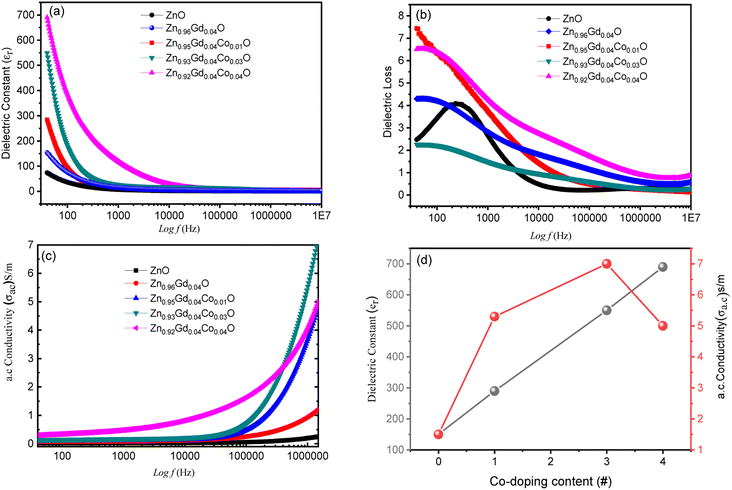 |
| Fig. 6 (a) Variation of εr versus (f) (b) the f dependence of ε′′ curves, (c) the variation of αa.c.with (f) curves and (d) phase diagram of dielectric constant and conductivity of pure and Gd doped ZnO and (Gd (fixed), Co)–ZnO co-doped with 4% Gd and Co = 1, 3 and 4%. | |
The εr values for all samples decrease uniformly with increasing f from 1.5 × 104 Hz and remain constant at higher frequencies. Usually, there are two types of polarizations i.e. space charge polarization (SCP) and rotation dielectric polarization (RDP) are responsible for large values of dielectric constants observed. The interfacial region contains many defects like dangling bonds, oxygen vacancies (OVS) and vacancy clusters, etc., where oxygen vacancies can be ionized into single or double ionized vacancies.29,31
There will be a large amount of dipole moment (random in direction) generated due to the presence of positive and negative vacancies and the presence of an external magnetic field can only polarize them to induce RDP. Interfacial defects in these nanocrystalline materials will also trap space charge migrating opposite to the electric fields38,48 thereby inducing SCP and enhanced dielectric constant. This causes grain boundaries to be electrically active. The observed decrease in εr with increasing (f) is due to the fact that any species contributing to polarizability is bound to lag behind the applied field. The interfacial area also increases when particles become small and this increases the SCP and RDP whilst Co co-doping enhances the OVS to enhance SCP and RDP. Thus, both crystallize size and doping concentration affect dielectric properties. The εr increases with an increase in Co co-doping concentration as shown in Fig. 5(a) and (d) and this emanates from the lattice distortion when small-sized Co2+ ions substitute Zn2+ ions as previously reported in ref. 26.
3.2.2 Dielectric loss. Fig. 6(b) displays the changes in dielectric loss (ε′′) with the (f) of the doped and co-doped ZnO nanoparticles. Here, it is observed that enhancing the frequency, ε′′ decreased. Similar behaviour was observed in all the specimens i.e. dispersion at low (f) and (f) independence at high frequency. The decrease in ε′′ observed at high frequencies is due to ionic migration. The (ε′′) found at low and intermediate frequencies (fs) is also attributable to ionic hopping and conduction losses induced by charge transport. Ionic polarisation losses can contribute to this phenomenon. However, at high (fs), ion vibrations might be the only reason.
3.2.3 Electrical conductivity. Fig. 6(c) illustrates the (f) AC conductivity (αa.c.), AC, of (Gd, Co) co-doped ZnO samples. The σa.c. rises with (f) at each voltage and these results are consistent with those reported before.49 Thus, initially, σa.c. rises slowly with an increase in f, whilst at higher frequencies, conductivity sharply increases. Such behaviour is caused by the hopping concept; at low frequency, the σa.c. is constant even though charge carriers travel along infinite pathways, whereas transport is through hopping of charge carriers for f-dependent σa.c.. For all specimens, σa.c. follows the below relationshipwhere αa.c. is the a.c. electrical conductivity, ε′′ is the imaginary, εr is the dielectric constant of free space, part of the dielectric constant, and ω = 2πf, f is the frequency. Eqn (2) displays that αa.c. only depends on the ε′′. Thus, the ε′′ decreases with an increase in f, whilst αa.c. enhances. This result corroborates well with previous results, where it was shown that αa.c. increases with an enhancement in (f) due to the series resistance effect.50 There are two possible mechanisms responsible for this behaviour and these are (1) promotion of jumping of charge carriers by electric energy related to the high (f) and (2) enhanced dielectric relaxation of ZnO NTs polarization in the high (f) region. Thus, co-doped ZnO nanopowder is an excellent material for high-energy storage devices. Moreover, enhanced σa.c. observed may be useful for industrial gas sensing applications due to enhanced electron transmission.51,52 Fig. 6(d) showed the phase diagram of the dielectric constant and conductivity versus doping. It showed that the dielectric behaviour and conductivity increases with doping (as shown in Fig. 5(c) and (d)) because the number of free electrons increases with the addition of impurity ions, which will further help in conduction.
3.3 Magnetic properties
Fig. 7(a) depicts magnetic hysteresis (M–H) loops of (Gd, Co) co-doped ZnO NTs recorded at ambient temperature. For pure ZnO, the M–H loop shows diamagnetism at 300 K [its data is not shown here] whilst Zn0.96Gd0.04O and Zn0.96−xGd0.04CoxO (Co = 0, 0.01, 0.03, 0.04) samples demonstrate an obvious ferromagnetic (FM) response. Fig. 7(a) and (b) shows that for Zn0.96−xGd0.04CoxO (Co = 0, 0.01, 0.03, 0.04) specimens, the remanent magnetization (Mr) values are ∼0.3367 emu g−1, 0.4413 emu g−1, 0.5788 emu g−1, 0.4538 emu g−1 along with coercive field are 47 Oe, 65 Oe, 76 Oe, 49 Oe, respectively. The situation magnetization values 0.52, 1.97, 2.67 and 1.52 (×10−2 emu g−1) values are listed in Table 2. The table shows that the Mr value of Zn0.92Gd0.04Co0.04O specimen is higher than the literature.23 The transition from paramagnetic to FM states is evident in Fig. 7. Mr values of Zn0.96−xGd0.04CoxO (Co = 0.03) specimens are larger than that of pure ZnO. This is due to the fact that O2 annealing can increase the doping level of Gd and Co ions within the host lattice, hence increasing the number of defects. The RTFM in the 1% Co co-doped specimen may be emanating from intrinsic and extrinsic magnetism sources. Extrinsic sources involve the formation of clusters of transition elements or secondary phases while intrinsic sources involve exchange interactions.
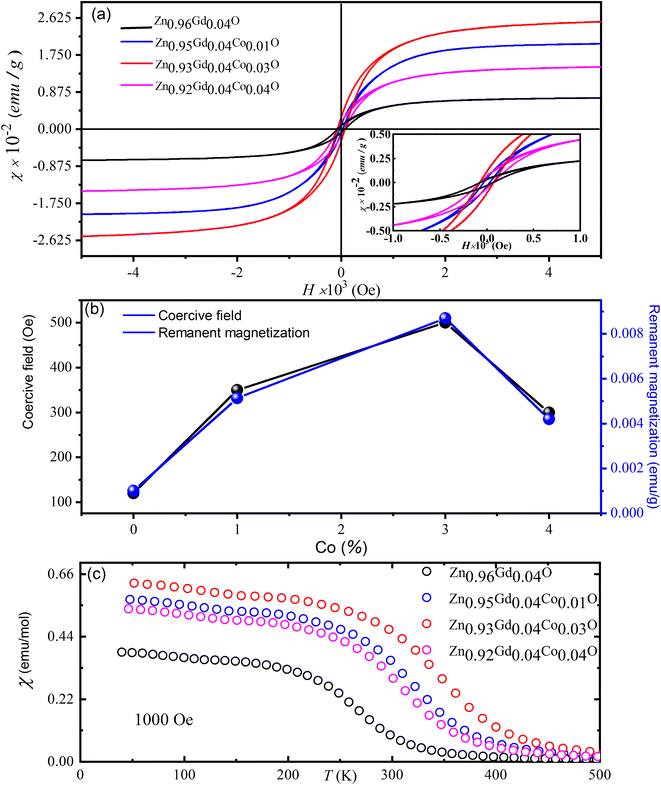 |
| Fig. 7 (a) The magnetic hysteresis (M–H) loops of the (Gd, Co) co-doped ZnO NTs and (b) their corresponding temperature-dependent magnetization and (c) the magnetization versus temperature curves for all the doped ZnO NTS. | |
Table 2 The magnetic parameters of (Gd, Co) co-doped ZnO magnetic NTs
Sample |
Remanent magnetization (Mr) (×10−2 emu g−1) |
Coercive field (Oe) |
Saturation magnetization (Ms) (×10−2 emu g−1) |
Zn0.96Gd0.04O |
0.3367 |
50 |
0.52 |
Zn0.96Gd0.04Co0.01O |
0.4413 |
68 |
1.97 |
Zn0.93Gd0.04Co0.03O |
0.5788 |
83 |
2.67 |
Zn0.92Gd0.04Co0.04O |
0.4538 |
53 |
1.52 |
To further explain the magnetic behavior, plots of magnetization with temperature under a magnetic field of 103 Oe are displayed in the Fig. 7(c). These data show that Zn0.96−xGd0.04CoxO (Co = 0.03) samples have higher FM behavior than either pure Gd or higher Co co-doped specimens. The origins of FM in TM-ZnO51,52 were shown to be due to coupling between TM ions and bound polarons to form bound magnetic polarons. According to the research of defect-bound transporters for point defect hybridization, TM-doped produces RTFM in doped ZnO. In this study, we show that O2 annealing can enhance the substitution of Gd and Co into the ZnO lattice. RTFM in Zn0.96−xGd0.04CoxO NTs are caused by doping of Gd3+ and Co2+ for Zn2+ and O2 during annealing to produce O2 vacancies for maintaining charge balance. This is an interesting novel phenomenon introduced during the specimen preparation process.
As displayed in Fig. 7(c), the lower around 350 K TC is visible in the χ(T) Co = 0.03. The displayed decrease in the magnetic moment for Co with higher depends may be related to Co co-doped ZnO specimens' structural property. The changes in cell parameters indicate that lattice constants, and consequently unit cell volume, raise as cobalt concentration rises. The expansion of the unit cell volume increases the distance between the nearest cobalt ions in the ZnO matrix, and this results in antiferromagnetic super-exchange interactions between adjacent Co ions, thereby an increase in magnetic moment. The effect of temperature on the nanoparticles' inverse magnetic susceptibility between 2 and 300 K. It is seen that the inverse susceptibility goes down in a straight line until 380 K, when it drops off the Curie–Weiss line. The modified Curie–Weiss expression fits well with the susceptibility of the nanoparticles we used in our experiments as mentioned below,
χ(T) = χo + (1/8)μeff2x/(T − θC) |
where
χo represents the temperature-independent susceptibility,
C the Curie constant and
θC is the Curie–Weiss temperature,
x is the concentration of Co ions and
μeff is the effective moment. The calculated Curie–Weiss law parameters
i.e., effective moment
μeff = 2.45 μ
B and
θ = −25 K. In contrast, values for replaced Zn
0.96−xGd
0.04Co
xO (
x = 0.0, 0.1, 0.3, and 0.4) samples are found to decrease with increasing Co co-doped concentration
x, with effective moment values ranging between 2.46 and 2.49 μ
B, provide information's related with the exchange integral
j, which is a measure of the interaction between the magnetic ions. The negative values of suggest that the magnetic dopants have a relatively weak antiferromagnetic interaction.
53,54 Most other oxide compounds have a value between 24 and 27 K, which is virtually as low as the value for Co-doped ZnO. Most other oxide compounds have a value between 24 and 27 K, which is virtually as low as the value for Co-doped ZnO. The magnetic properties found at low temperatures may result from flaws introduced during manufacturing or the existence of impurity phases. Clarification of this aspect requires further research.
4. Conclusions
ZnO and ferromagnetic (Gd, Co) co-doped ZnO NTs were successfully fabricated in this work. XRD measurements show that all the (Gd, Co) co-doped specimens have a tetragonal structure. With an increase in either (f) or Co dopant concentration, both dielectric constant and dielectric loss and electrical conductivity increases. The electrical conductivity of annealed Gd and Co-doped ZnO specimens was found to be enhanced. This occurred as a result of the substitution of Zn ions with Gd and Co ions, which increased the number of available charge carriers. A significant change in the hysteresis loop was observed, characterized by the change from the diamagnetic behavior of ZnO to ferromagnetic when co-doped with (Gd, Co). The enhanced dielectric and magnetic responses of the specimens are strongly correlated to an increase in O2 vacancies and zinc interstitials. Our results indicate that ferromagnetism in the ZnO NTs can be introduced through Gd fixed and limited Co doping and annealing at 500 °C due to the production of extra charge carriers and O2 vacancies.
Data availability
The datasets generated during and/or analyzed during the current study are available from the corresponding author upon reasonable request. Also, the manuscript's experimental work and wording is original. No part of the was manuscript found plagiarized. If the reviewer further needs the proof then we will send the plagiarized proof.
Author contributions
Rajwali Khan, Ihab Shigidi, Sattam Al Otaibi, Khaled Althubeiti, Nasir Rahman, Mohammad Sohail, Alamzeb Khan, Shahid Iqbal, Tommaso Del Rosso, Quaid Zaman, Aurangzeb Khan write this paper with their mutual discussion. Aurangzeb Khan and Rajwali Khan, created the idea and submitted the paper.
Funding
The authors extend their appreciation to the Deanship of Scientific Research at King Khalid University for funding this work through Small Groups Project under grant number RGP.1/25/43.
Conflicts of interest
The authors declare that they have no known competing financial interests or personal relationships that could have influenced the work reported in this paper.
Acknowledgements
The authors acknowledge the financial support from King Khalid University for sponsorship and support. We also acknowledge the support and guidance provided by King Khalid University.
References
- Y. Hao, et al., Novel magnetic behavior of Mn-doped ZnO hierarchical hollow spheres, J. Nanopart. Res., 2012, 14(1), 1–9 CrossRef.
- G. A. Prinz, Magnetoelectronics, Science, 1998, 282(5394), 1660–1663 CrossRef CAS PubMed.
- R. Khan, et al., Structure and magnetic properties of (Co, Ce) co-doped ZnO-based diluted magnetic semiconductor nanoparticles, J. Mater. Sci.: Mater. Electron., 2021, 32(19), 24394–24400 CrossRef CAS.
- P. Li, et al., Structural and optical properties of Co-doped ZnO nanocrystallites prepared by a one-step solution route, J. Lumin., 2012, 132(1), 220–225 CrossRef CAS.
- R. Khan, et al., Effect of Sn-doping on the structural, optical, dielectric and magnetic properties of ZnO nanoparticles for spintronics applications, J. Mater. Sci.: Mater. Electron., 2021, 32(16), 21631–21642 CrossRef CAS.
- X. Wang, et al., Properties of Ni doped and Ni–Ga co-doped ZnO thin films prepared by pulsed laser deposition, J. Alloys Compd., 2011, 509(7), 3282–3285 CrossRef CAS.
- Y.-M. Hao, et al., Structural, optical, and magnetic studies of manganese-doped zinc oxide hierarchical microspheres by self-assembly of nanoparticles, Nanoscale Res. Lett., 2012, 7(1), 1–9 CrossRef.
- A. Stroppa, X. Duan and M. Peressi, Structural and magnetic properties of Mn-doped GaAs(1 1 0) surface, Mater. Sci. Eng. B, 2006, 126(2–3), 217–221 CrossRef CAS.
- Y. Chang, et al., Synthesis, optical, and magnetic properties of diluted magnetic semiconductor Zn1−xMnxO nanowires via vapor phase growth, Appl. Phys. Lett., 2003, 83(19), 4020–4022 CrossRef CAS.
- Y. Ohno, et al., Electrical spin injection in a ferromagnetic semiconductor heterostructure, Nature, 1999, 402(6763), 790–792 CrossRef CAS.
- L. Sun, et al., Room-temperature ferromagnetism and in-plane magnetic anisotropy characteristics of nonpolar GaN:Mn films, Appl. Surf. Sci., 2009, 255(16), 7451–7454 CrossRef CAS.
- T. Dietl, A ten-year perspective on dilute magnetic semiconductors and oxides, Nat. Mater., 2010, 9(12), 965–974 CrossRef CAS.
- X. Xu and C. Cao, Structure and ferromagnetic properties of Co-doped ZnO powders, J. Magn. Magn. Mater., 2009, 321(14), 2216–2219 CrossRef CAS.
- C. F. Klingshirn, Optical properties of bound and localized excitons and of defect states, in Semiconductor Optics, 2012, Springer, pp. 363–382 Search PubMed.
- Q. Wang, Q. Sun and P. Jena, Ab initio study of electronic and magnetic properties of the C-codoped Ga1−xMnxN (10
0) surface, Phys. Rev. B: Condens. Matter Mater. Phys., 2007, 75(3), 035322 CrossRef. - J. Fu, et al., Synthesis and structural characterization of ZnO doped with Co, J. Alloys Compd., 2013, 558, 212–221 CrossRef CAS.
- N. G. Szwacki, J. Majewski and T. Dietl, Aggregation and magnetism of Cr, Mn, and Fe cations in GaN, Phys. Rev. B: Condens. Matter Mater. Phys., 2011, 83(18), 184417 CrossRef.
- Z. Lu, et al., Carrier-mediated ferromagnetism in single crystalline (Co, Ga)-codoped ZnO films, Appl. Phys. Lett., 2009, 94(15), 152507 CrossRef.
- G. Husnain, F. Tao and S.-D. Yao, Structural and magnetic properties of Co+ implanted n-GaN dilute magnetic semiconductors, Phys. B, 2010, 405(9), 2340–2343 CrossRef CAS.
- V. Gandhi, et al., Effect of cobalt doping on structural, optical, and magnetic properties of ZnO nanoparticles synthesized by coprecipitation method, J. Phys. Chem. C, 2014, 118(18), 9715–9725 CrossRef CAS.
- H. Hao, M. Qin and P. Li, Structural, optical, and magnetic properties of Co-doped ZnO nanorods fabricated by a facile solution route, J. Alloys Compd., 2012, 515, 143–148 CrossRef CAS.
- T. Oshio, et al., Effect of Mn doping on the electric and dielectric properties of ZnO epitaxial films, J. Appl. Phys., 2008, 103(9), 093717 CrossRef.
- R. Khan, M.-U. Rahman and S. Fashu, Effect of annealing temperature on the dielectric and magnetic response of (Co, Zn) co-doped SnO2 nanoparticles, J. Mater. Sci.: Mater. Electron., 2017, 28(3), 2673–2679 CrossRef CAS.
- A. Yu, et al., Micro-lotus constructed by Fe-doped ZnO hierarchically porous nanosheets: preparation, characterization and gas sensing property, Sens. Actuators, B, 2011, 158(1), 9–16 CrossRef CAS.
- R. Khan and Y. Zaman, Effect of annealing on structural, dielectric, transport and magnetic properties of (Zn, Co) co-doped SnO2 nanoparticles, J. Mater. Sci.: Mater. Electron., 2016, 27(4), 4003–4010 CrossRef CAS.
- R. Khan, S. Fashu and M.-U. Rahman, Effects of Ni co-doping concentrations on dielectric and magnetic properties of (Co, Ni) co-doped SnO2 nanoparticles, J. Mater. Sci.: Mater. Electron., 2016, 27(8), 7725–7730 CrossRef CAS.
- R. Khan and S. Fashu, Effect of annealing on Ni-doped ZnO nanoparticles synthesized by the co-precipitation method, J. Mater. Sci.: Mater. Electron., 2017, 28(14), 10122–10130 CrossRef CAS.
- C. Cong, et al., Effects of temperature on the ferromagnetism of Mn-doped ZnO nanoparticles and Mn-related Raman vibration, Nanotechnology, 2006, 17(5), 1520 CrossRef CAS.
- R. Khan, S. Fashu and Y. Zaman, Magnetic and dielectric properties of (Co, Zn) co-doped SnO2 diluted magnetic semiconducting nanoparticles, J. Mater. Sci.: Mater. Electron., 2016, 27(6), 5960–5966 CrossRef CAS.
- T. Castro, et al., Size controlling and tailoring the properties of GdxZn1-xO nanoparticles, Ceram. Int., 2022, 48(3), 4324–4331 CrossRef CAS.
- R. Khan, et al., Structure and magnetic properties of (Co, Mn) co-doped ZnO diluted magnetic semiconductor nanoparticles, J. Mater. Sci.: Mater. Electron., 2018, 29(1), 32–37 CrossRef CAS.
- K. Rajwali and M.-H. Fang, Dielectric and magnetic properties of (Zn, Co) co-doped SnO2 nanoparticles, Chin. Phys. B, 2015, 24(12), 127803 CrossRef.
- R. Khan, et al., Effect of air annealing on the structure, dielectric and magnetic properties of (Co, Ni) co-doped SnO2 nanoparticles, J. Mater. Sci.: Mater. Electron., 2016, 27(10), 10532–10540 CrossRef CAS.
- P. Jongnavakit, et al., Preparation and photocatalytic activity of Cu-doped ZnO thin films prepared by the sol–gel method, Appl. Surf. Sci., 2012, 258(20), 8192–8198 CrossRef CAS.
- W.-J. Qin, et al., Control of Cu-doping and optical properties of ZnO quantum dots by laser ablation of composite targets, Mater. Chem. Phys., 2011, 130(1–2), 425–430 CrossRef CAS.
- K. J. Kim and Y. R. Park, Optical absorption and electronic structure of Zn1−xMnxO alloys studied by spectroscopic ellipsometry, J. Appl. Phys., 2003, 94(2), 867–869 CrossRef CAS.
- J. Han, et al., Optical and dielectric properties of ZnO tetrapod structures at terahertz frequencies, Appl. Phys. Lett., 2006, 89(3), 031107 CrossRef.
- F. Gu, et al., Photoluminescence properties of SnO2 nanoparticles synthesized by sol–gel method, J. Phys. Chem. B, 2004, 108(24), 8119–8123 CrossRef CAS.
- S. Deka and P. Joy, Synthesis and magnetic properties of Mn doped ZnO nanowires, Solid State Commun., 2007, 142(4), 190–194 CrossRef CAS.
- M. Patra, et al., Studies on structural and magnetic properties of Co-doped pyramidal ZnO nanorods synthesized by solution growth technique, J. Phys. Chem. Solids, 2009, 70(3–4), 659–664 CrossRef CAS.
- K. Safeen, et al., Synthesis of Nb-doped TiO2 films on rigid and flexible substrates at low temperature, Mod. Phys. Lett. B, 2019, 33(26), 1950313 CrossRef CAS.
- M. Ahmad, et al., Preparation of highly efficient Al-doped ZnO photocatalyst by combustion synthesis, Curr. Appl. Phys., 2013, 13(4), 697–704 CrossRef.
- C. Belkhaoui, et al., Enhancing the structural, optical and electrical properties of ZnO nanopowders through (Al+Mn) doping, Results Phys., 2019, 12, 1686–1696 CrossRef.
- R. Bylsma, et al., Dependence of energy gap on x and T in Zn1−xMnxSe: the role of exchange interaction, Phys. Rev. B: Condens. Matter Mater. Phys., 1986, 33(12), 8207 CrossRef CAS PubMed.
- A. Layek, B. Manna and A. Chowdhury, Carrier recombination dynamics through defect states of ZnO nanocrystals: from nanoparticles to nanorods, Chem. Phys. Lett., 2012, 539, 133–138 CrossRef.
- C. Mrabet, et al., Some physical investigations on hexagonal-shaped nanorods of lanthanum-doped ZnO, J. Alloys Compd., 2015, 648, 826–837 CrossRef CAS.
- E. Pragna, et al., Nano Synthesis and Characterization of Co and Mn Co-Doped ZnO by Solution Combustion Technique, J. Supercond. Novel Magn., 2021, 1–10 Search PubMed.
- S.-P. Szu and C.-Y. Lin, AC impedance studies of copper doped silica glass, Mater. Chem. Phys., 2003, 82(2), 295–300 CrossRef CAS.
- O. Pakma, et al., Influence of frequency and bias voltage on dielectric properties and electrical conductivity of Al/TiO2/p-Si/p+(MOS) structures, J. Phys. D: Appl. Phys., 2008, 41(21), 215103 CrossRef.
- R. Elilarassi and G. Chandrasekaran, Synthesis and characterization of ball milled Fe-doped ZnO diluted magnetic semiconductor, Optoelectronics Letters, 2012, 8(2), 109–112 CrossRef.
- Y. Lin, et al., Fe-doped ZnO magnetic semiconductor by mechanical alloying, J. Alloys Compd., 2007, 436(1–2), 30–33 CrossRef CAS.
- S. Yin, et al., Absence of ferromagnetism in bulk polycrystalline Zn0.9Co0.1O, Phys. Rev. B: Condens. Matter Mater. Phys., 2006, 73(22), 224408 CrossRef.
- T. Fukumura, et al., Magnetic properties of Mn-doped ZnO, Appl. Phys. Lett., 2001, 78(7), 958–960 CrossRef CAS.
- L. Yang, et al., In situ synthesis of Mn-doped ZnO multileg nanostructures and Mn-related Raman vibration, J. Appl. Phys., 2005, 97(1), 014308 CrossRef.
Footnote |
† CEO of the Company of Editory, Tashkent, Uzbekistan. |
|
This journal is © The Royal Society of Chemistry 2022 |