DOI:
10.1039/D2RA05676C
(Paper)
RSC Adv., 2022,
12, 31778-31785
Photophysics of tetracarboxy-zinc phthalocyanine photosensitizers
Received
8th September 2022
, Accepted 20th October 2022
First published on 7th November 2022
Abstract
Zinc-tetracarboxy-phthalocyanine (ZnPc(COOH)4) was synthesized by a melting method and basic hydrolysis. A ZnPc(COOH)4/Fe3O4/Ch composite was prepared by immobilization of ZnPc(COOH)4 onto Fe3O4/chitosan nanoparticles by a simple immersion method. The photophysical properties were studied using UV-vis spectrophotometry, fluorescence spectroscopy and time-correlated single photon counting (TCSPC) in different aqueous solutions. The UV-vis spectra of the ZnPc(COOH)4/Fe3O4/Ch composite displays absorption by the aromatic rings, with a Q band exhibited at λmax = 702 nm. Moreover, the ZnPc(COOH)4/Fe3O4/Ch composite exhibits long triplet-state lifetimes of 1.6 μs and 12.3 μs, crucial for application as a photosensitizer. A triplet quantum yield of 0.56 for the ZnPc(COOH)4/Fe3O4/Ch composite in DMSO/H2O was achieved. FTIR showed that the conjugation of ZnPc(COOH)4 with Fe3O4/chitosan nanoparticles was achieved by electrostatic interaction.
Introduction
Metallophthalocyanine (MPc) derivatives are popular photodynamic therapy (PDT) photosensitizers (PSs). Research on a novel PS requires extensive human effort and a high cost investment over decades before clinical application. Nevertheless, some MPc derivatives, such as: aluminium phthalocyanine (Photosens®, Russia), used against skin, breast and lung malignancies, and cancers of the gastrointestinal tract;1 silicon Pc (Pc 4, USA), for the sterilization of blood components against human colon, breast and ovarian cancers, and gliomas;2 and a liposomal zinc phthalocyanine formulation, using a controlled organic solvent dilution against squamous cell carcinomas of the upper aerodigestive tract,3 have undergone clinical trials.
Current efforts are being made in the development of new photosensitizers (PSs) with improved solubility in body fluids and injectable solvents, photostability, enhanced permeability and retention effect, elimination and cumulative systemic toxicity.4–9 In the field of organic photosensitizers, metallophthalocyanines (MPcs) play an important role due to their excellent photo- and electro-chemical stability and exclusive light-harvesting capability in the red/NIR spectral regions.10–13
The main disadvantages of MPcs in PDT are the lack of solubility and selectivity; therefore, the combination of magnetic iron oxide nanoparticles with a photosensitizer is a new and promising approach in PDT. Fe3O4 nanoparticles have been successfully applied in tumor therapy by inducing hyperthermia and oxidative stress that lead to tumor cell damage.14–16 For application in PDT, magnetic nanoparticles (NPs) are usually coated with polymers, bound to the particle through organic linkers.17 Functionalization of Fe3O4 nanoparticles may lead to enhancement of their biocompatibility, colloidal stability, and an increase in the number of groups, through which the required antitumor effect can be obtained.
The major goal of this paper is to create a new photosensitizer with adequate solubility, especially in body fluids and injectable solvents, with greater tumor selectivity, enhanced hydrophilicity, and strong absorption in the NIR spectral region. Therefore, conjugation of an MPc derivative to a magnetic NP functionalized with a polymer is the first part of our research aimed at delivering PSs to tumor cells. The magnetic iron oxide nanoparticles will be used as the carrier of the photosensitizer because of: their ability to carry and deliver therapeutic photosensitizers into deep-seated tumours; the enhanced solubility of the hydrophobic PS with an appropriate size to accumulate in the tumour tissues via enhanced permeability and retention effect; and the ability to attack cancer cells selectively without harming other healthy cells. The Fe3O4 NPs will be functionalized with chitosan, which is a biodegradable, biocompatible polysaccharide and, in comparison with many other polymers, has many free –OH and –NH2 groups that can serve as anchors for conjugation of therapeutics and targeting ligands.
Considering the above mentioned information, we focused our research on attaching functionalized ZnPc with carboxylic groups (–COOH) to an Fe3O4/chitosan system hoping to get a synergistic effect in the photodynamic parameters of the resulting composite.
Materials and methods
Materials
All materials, trimellitic anhydride, zinc acetate dehydrate (Zn(CH3COOH)2·2H2O), ammonium molybdate tetrahydrate ((NH4)6MO7O24)·4H2O, anhydrous sodium sulfate (Na2SO4), urea, 1-bromo-naphthalene, hydrochloric acid and acetic acid were purchased from Sigma-Aldrich, Inc., St. Louis, MO, USA and used as received. Dimethyl sulfoxide (DMSO), chitosan (Ch) and hydrogen peroxide (H2O2) were obtained from Alfa Aesar, Heysham, UK. Fe3O4 nanoparticles (Merck) were cleaned in a flux of hydrogen at room temperature for 20 minutes.
Equipment
The UV-vis spectra of the solutions were measured using a UV-vis spectrophotometer (Lambda 25, PerkinElmer, Inc., Shelton, CT, USA) from 200 nm to 1200 nm in 10 mm quartz cuvettes. The steady-state fluorescence spectroscopy was performed using a spectrometer (LS-55, PerkinElmer, Inc., Shelton, CT, USA) equipped with double-grating excitation and emission monochromators. Time-correlated single photon counting (TCSPC) was used to determine the fluorescence lifetime. The time-resolved fluorescence spectra were recorded on a spectrometer (FLS980, Edinburgh Instruments, Livingston EH54 7DQ, Oxford, UK). All the measurements were made at room temperature (295 ± 1 K). A Bruker D8 ADVANCE X-ray diffractometer (using Cu Kα radiation with λ = 1.5406 Å) was used for structural investigation of the magnetic nanoparticles. A Bruker FTIR spectrometer was used to provide information about the chemical composition.
Synthesis
The synthetic pathway of ZnPc(COOH)4
A mixture consisting of 4.35 g (0.022 mol) of trimellitic anhydride, 2.52 g of Zn(CH3COOH)2·2H2O, 0.3 g of ((NH4)6MO7O24)·4H2O, 0.5 g of Na2SO4, 13.51 g (0.225 mol) of urea and 5 ml of 1-bromonaphthalene was heated at 200–205 °C for 8 h with continuous stirring. After 8 hours, the reaction mixture was cooled to room temperature and treated with methanol. The obtained suspension was filtered. The solid reaction product was washed on the filter with methanol, chloroform and, finally, with acetone. After drying, the product was crumbled and then refluxed for one hour in 5% hydrochloric acid (HCl) solution. After drying, the same procedure was carried out with 5% sodium hydroxide (NaOH) solution for one hour at 90 °C. Finally, the solution was acidified with HCl until the pH was equal to 2, and the precipitated final product was filtered and dried in the open air. 0.68 g of ZnPc(COOH)4 was obtained with a yield of 70% (Fig. 1).
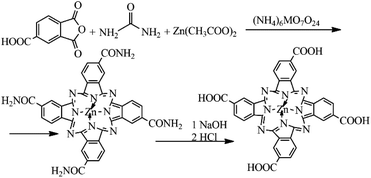 |
| Fig. 1 Schematic diagram of ZnPc(COOH)4 synthesis. | |
Preparation of chitosan-functionalized magnetic nanoparticles
Chitosan and Fe3O4 were mixed in an appropriate proportion to form the chitosan–magnetic nanoparticles composite with amine groups by the reverse-phase suspension cross-linking method.18 Aqueous acetic acid solution was used as a solvent for the chitosan polymer and H2O2 was used as the cross-linker. In this specific procedure, a chitosan solution was prepared using a mixture of 2% acetic acid and 10% H2O2 solutions. Then 0.2 g Fe3O4 was added and stirred with strong ultrasonic agitation at room temperature for 4 h. At the end of this period, some of the chitosan-Fe3O4 nanocomposite particles were collected from the reaction mixture by using a permanent magnet. The product was washed with ethanol and dried in vacuum at 60 °C for 5 hours and used for XRD analysis (Fig. 2).
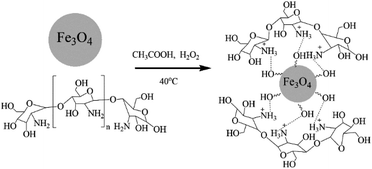 |
| Fig. 2 Schematic diagram of the preparation of chitosan-functionalized magnetic nanoparticles. | |
Chitosan is able to interact with negatively charged molecules,19 such as the hydroxyl (Fe–OH) groups on the surface of magnetite nanoparticles. The presence of –OH groups on the surface of the Fe3O4 nanoparticles was confirmed by the strong broad band with a maximum at 3431 cm−1 in the IR spectrum (Fig. 4), corresponding to ν(O–H) oscillations. We suppose that ionic interactions occur between the negatively charged CH3COO− species and the positively charged (NH3+) groups of the chitosan molecules dissolved in the aqueous acetic acid solution.
The conjugation of ZnPc(COOH)4 to chitosan, Fe3O4 and Fe3O4/Ch nanoparticles
Acetic acid is a weak acid and is a very common solvent for chitosan. A sample of 0.3 g of chitosan was dissolved in 50 ml of 2% concentrated acetic acid. Then 0.5 ml of 10% hydrogen peroxide was added to the solution for the destruction of intermacromolecular hydrogen bonds and interchain hydrogen bonds to make water-soluble chitosan. The appropriate ratio of chitosan to acetic acid in the chitosan–acetic acid solution was 1
:
0.5, and then ZnPc(COOH)4 was dissolved in a 1
:
1 DMSO/H2O solution. After that, both solutions were mixed, heated at 40 °C and stirred continuously for 40 min.
In a separate experiment, ZnPc(COOH)4 solution was mixed with a dispersion medium containing chitosan-functionalized magnetic nanoparticles at room temperature and stirred for 2 h using a mechanical stirrer.
Experiments where ZnPc(COOH)4 was dissolved in 1
:
1 DMSO/H2O solution and simply mixed with Fe3O4 were also performed.
Results and discussion
Structural analysis of the Fe3O4 and Fe3O4/chitosan magnetic nanoparticles
The X-ray diffraction patterns of the Fe3O4 and Fe3O4/chitosan nanoparticles, along with the standard pattern of Fe3O4 (JCPDS #75-0033), are shown in Fig. 3 and details of the peaks are given in Table 1. The similar XRD patterns reveal that Fe3O4 does not undergo any phase changes following functionalization with chitosan, a situation also confirmed by other reports.20,21
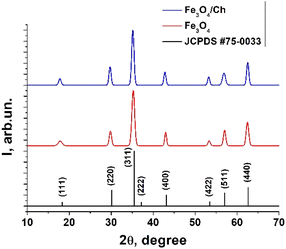 |
| Fig. 3 XRD patterns of the Fe3O4 and Fe3O4/chitosan nanoparticles, and the JCPDS #75-0033 card. | |
Table 1 Structural parameters of the Fe3O4 and Fe3O4/chitosan nanoparticles
|
2θ |
FWHM |
D, nm |
Dmean, nm |
Fe3O4 |
17.84 |
1.05 |
8.5 |
13.95 |
29.81 |
0.67 |
13.7 |
35.25 |
0.79 |
11. |
42.99 |
0.52 |
18.1 |
53.35 |
0.62 |
15.9 |
57.03 |
0.66 |
15.3 |
62.47 |
0.72 |
14.3 |
Fe3O4/Ch |
17.78 |
0.66 |
13.5 |
14.80 |
29.72 |
0.61 |
15.0 |
35.16 |
0.66 |
14.1 |
42.81 |
0.60 |
15.8 |
53.24 |
0.59 |
16.7 |
56.88 |
0.86 |
11.7 |
62.54 |
0.61 |
16.8 |
XRD diffraction analysis revealed a broad nature of the diffraction maxima, indicating that Fe3O4 has small crystallite sizes. The crystallite sizes were evaluated using the Debye–Scherrer formula:
|
D = (kλ/β cos θ)
| (1) |
where
λ is the wavelength of the X-rays (1.5406 Å),
β is the FWHM (full width at half maximum),
θ is the diffraction angle,
k = 0.94 and
D is the crystallite size. The metal oxide nanoparticles have a mean crystallite size of 13.95 nm. During the coating process with chitosan, the crystallite size slightly increases, as the size of the individual crystallite is related to the thickness of the chitosan layer. The mean crystallite size of the nanoparticles with chitosan increases up to 14.80 nm.
FTIR analysis
The FTIR spectra of chitosan, the Fe3O4 and Fe3O4/chitosan nanoparticles, ZnPc(COOH)4 and the ZnPc(COOH)4/Fe3O4/chitosan composite are presented in Fig. 4 and 5. The intense peak observed at 636 cm−1 in the FTIR spectrum of Fe3O4 is attributed to the stretching vibration mode associated with Fe–O bonds in the crystalline lattice of the Fe3O4 nanoparticles. This vibration is shifted to 641 cm−1 in the FTIR spectrum of the Fe3O4/chitosan nanoparticles. The modification and shift of the main characteristic bands (stretching C–O at 1024 cm−1, bending NH2 at 1648 cm−1, stretching O–H 3300 cm−1 and C–H 2864 cm−1) in the IR spectrum of chitosan to 1073 and 1029 cm−1 (ν(C–O)), 1615 cm−1 (δ(NH2)), 3431 cm−1 (ν(O–H)), and 2935 and 2874 cm−1 (ν(C–H)) in the IR spectrum of the Fe3O4/chitosan system) demonstrate the binding of chitosan to the Fe3O4 nanoparticles (Fig. 4). The result is consistent with similar investigations.22,23 The chemical interaction of ZnPc(COOH)4 with the Fe3O4/chitosan system is confirmed by the shift of the signal from 1702 cm−1 (ν(C
O)) of the protonated COOH groups in the IR spectrum of ZnPc(COOH)4, associated with splitting, to 1660 cm−1 (νsym(COO)), and 1436 and 1406 cm−1 (νasym(COOO)) that correspond to deprotonated carboxylic groups. This can be explained by the dissociation of carboxylic groups and formation of electrostatic interactions between NH3+ and COO− fragments (Fig. 16a).
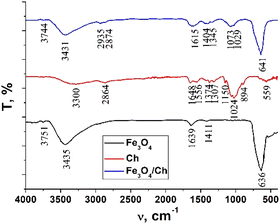 |
| Fig. 4 FTIR spectra of chitosan, the Fe3O4 nanoparticles and the Fe3O4/chitosan nanoparticles. | |
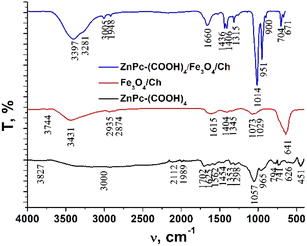 |
| Fig. 5 FTIR spectra of ZnPc(COOH)4, Fe3O4/chitosan and the ZnPc(COOH)4/Fe3O4/chitosan composite. | |
UV-vis and fluorescence analysis
Usually, MPcs give rise to electronic spectra with two strong absorption bands, one around 300 nm, called the “B” or Soret band, due to electronic transitions from the deeper π-HOMO to n*-LUMO energy levels, while the other at 600–650 nm, called the “Q” band, due to electronic transitions from the π-HOMO to π*-LUMO energy levels.24 The UV-vis spectra of ZnPc(COOH)4 and ZnPc(COOH)4/Ch in DMSO/H2O are presented in Fig. 6. The absorption spectra of the synthesized materials display absorption peaks in the visible region at around 700 nm. In the case of ZnPc(COOH)4 and ZnPc(COOH)4/Ch dissolved in DMSO/H2O, absorption maxima are situated at 640 and 697 nm, and 642 and 699 nm, respectively. The shifts of the Q band depend on the change in electron distribution in the phthalocyanine ring caused by the substituents and their position. The UV-vis spectra of ZnPc(COOH)4 immobilized on non-functionalized magnetic nanoparticles are shown in Fig. 7. The intensity of the absorption for the ZnPc(COOH)4/Fe3O4 material in a solution of 1
:
1
:
2 DMSO/Ac.ac/phys.sol. (where “Ac.ac” is acetic acid, and “phys.sol.” is a physiological NaCl solution) decreases when decreasing the molar concentration of ZnPc(COOH)4 from 0.33 mol m−3 to 0.042 mol m−3. The conjugation of ZnPc(COOH)4 with the chitosan-functionalized Fe3O4 nanoparticles gives rise to similar positions of the maxima in the Q band absorbance spectra (Fig. 8) as in the case when ZnPc(COOH)4 is mixed with the non-functionalized Fe3O4 nanoparticles. A difference appears in the width of the bands, with a narrower band being found for ZnPc(COOH)4/Fe3O4/Ch than for ZnPc(COOH)4/Fe3O4.
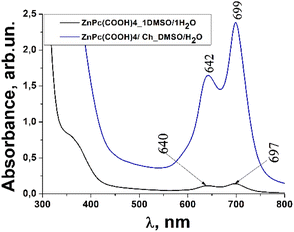 |
| Fig. 6 Absorbance spectra changes of the ZnPc(COOH)4 compound and ZnPc(COOH)4/Ch in DMSO/H2O solvent. | |
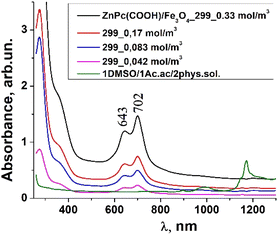 |
| Fig. 7 Absorbance spectra changes of ZnPc(COOH)4/Fe3O4 in DMSO/Ac.ac/phys.sol. solution, 1 : 1 : 2 ratio, at different molar concentrations. | |
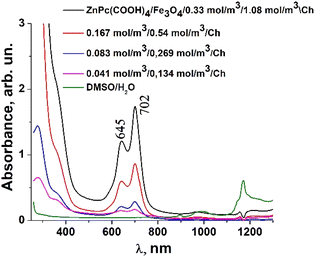 |
| Fig. 8 Absorbance of the ZnPc(COOH)4/Fe3O4/Ch composite in DMSO/H2O solution at different molar concentrations. | |
In Fig. 7, Fe3O4 was dissolved in a physiological solution of 0.9% NaCl and 2% acetic acid. For the Fe3O4/chitosan solution measurements shown in Fig. 8, 2% acid acetic and 10% hydrogen peroxide were used. The Q band extends into the 580–800 nm region and exhibited two peaks at λmax = 645 nm and 702 nm in the case of the Fe3O4 nanoparticles linked to chitosan (Fig. 8), almost the same values as when Fe3O4 is not bound to chitosan (Fig. 7). Both the ZnPc(COOH)4/Fe3O4/chitosan and ZnPc(COOH)4/Fe3O4 spectra (Fig. 9) show similar specific absorption peaks of the phthalocyanine aromatic ring. The chitosan had no obvious absorption peak in the visible region, but leads to an increased intensity of the 702 nm peak and a narrower Q band. The comparison in Fig. 9 allows us to suppose that the Q absorption band could be assigned to the π–π* transition on the ZnPc macrocycle. Introducing the peripheral –COOH substituent onto the macrocycle of ZnPc led to a significant bathochromic shift of the absorption spectra due to an increased destabilization of the HOMO electron state versus the LUMO state.
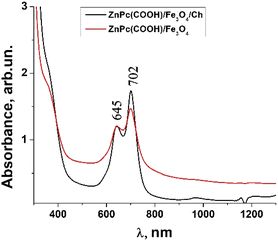 |
| Fig. 9 Comparison of the absorbance of the ZnPc(COOH)4/Fe3O4 and ZnPc(COOH)4/Fe3O4/Ch composites in DMSO/phys.sol. 0.9% and DMSO/H2O2/Ac.ac solutions. | |
The low energy peak is due to the monomer, while the high energy peak is caused by the aggregation. The aggregation species persisted more when the Fe3O4 nanoparticles were not bound to chitosan.
The fluorescence emission spectrum of ZnPc(COOH)4 in DMSO/H2O is shown in Fig. 10. The fluorescence spectrum after excitation at 615 nm shows two emission bands situated at 695 nm and 765 nm. The fluorescence spectrum of the ZnPc(COOH)4/chitosan system (Fig. 11) after excitation at 638 nm also shows two bands, as in Fig. 10, but they are both shifted 10 nm into the near-infrared region. The fluorescence spectrum of ZnPc(COOH)4 immobilized on the Fe3O4 magnetic nanoparticles shows broad and structured fluorescence at 702 nm, 764 nm, 789 nm and 826 nm, and shows an increase in intensity at 850 nm, when excited at 645 nm (Fig. 12). The limits of the measurement equipment did not allow us to record fluorescence above 850 nm. The spectrum of ZnPc(COOH)4 immobilized on the Fe3O4/chitosan magnetic nanoparticles shown in Fig. 13 displayed less structured fluorescence. Only two broad bands situated at 713 nm and 784 nm shifted to the near-infrared region are revealed. The resultant red-shift was associated with the electrostatic interaction between ZnPc(COOH)4 and the chitosan-functionalized Fe3O4 nanoparticles.
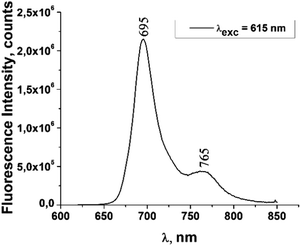 |
| Fig. 10 Emission spectrum of ZnPc(COOH)4 in DMSO/H2O solution. | |
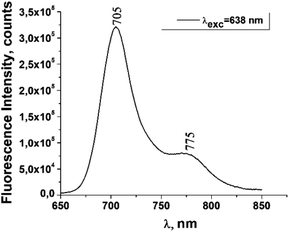 |
| Fig. 11 Emission spectrum of ZnPc(COOH)4/chitosan in DMSO/H2O solution. | |
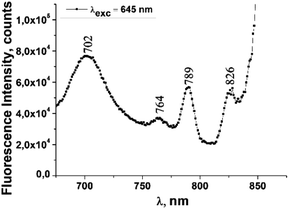 |
| Fig. 12 Emission spectrum of ZnPc(COOH)4/Fe3O4 in DMSO/phys.sol. 0.9% solution. | |
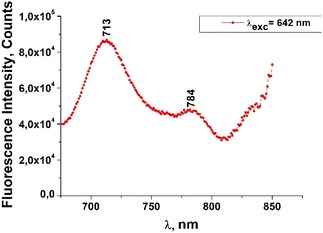 |
| Fig. 13 Emission spectrum of ZnPc(COOH)4/Fe3O4/chitosan in DMSO/H2O2/Ac.ac solution. | |
The fluorescence lifetimes of ZnPc(COOH)4 and ZnPc(COOH)4/chitosan in DMSO/H2O solution are presented in Fig. 14.
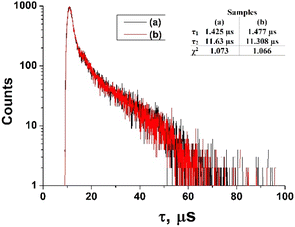 |
| Fig. 14 The fluorescence lifetimes of ZnPc(COOH)4 (a) and ZnPc(COOH)4/chitosan (b) in DMSO/H2O solution. | |
The fluorescence decays of ZnPc(COOH)4 and ZnPc(COOH)4/chitosan in DMSO/H2O solutions at about the same interval of excitation wavelengths (λexc = 684–772 nm) show a bi-exponential behaviour with lifetime values being 1.42 μs and 11.63 μs for the solution without chitosan, while, for the solution with chitosan, values of 1.47 μs and 11.31 μs are reported. The presence of chitosan does not induce significant variations in the fluorescence decay times. Thus, because the NH2 groups in chitosan can form the same ionic bonds with the COOH groups, even in the absence of Fe3O4, but only in the presence of Fe3O4, the fluorescence decay time changes. So, the presence of Fe3O4 NPs is vital for the main result of the chemical reactions of the developed composite.
In Fig. 15, the fluorescence lifetimes of ZnPc(COOH)4/Fe3O4 and ZnPc(COOH)4/Fe3O4/chitosan in DMSO/phys.sol. 0.9% and DMSO/H2O2/Ac.ac solutions are illustrated. The fluorescence decay curve for ZnPc(COOH)4/Fe3O4 yielded two lifetimes of 1.47 μs and 10.98 μs. The values are smaller than those of the ZnPc(COOH)4/Fe3O4/chitosan composite, although the contribution of the shorter lifetime component is less than 13%. The longer lifetime in the ZnPc(COOH)4/Fe3O4/chitosan composite can be explained by the magnetic nanoparticles’ functionalization with chitosan. The fluorescence quantum yield values of the ZnPc(COOH)4/Fe3O4 or ZnPc(COOH)4/Fe3O4/chitosan composites in DMSO/phys.sol. 0.9% and DMSO/H2O2/Ac.ac solutions of 1
:
1 ratio were found to be lower than that for non-substituted ZnPc in non-diluted DMSO (0.67 in ref. 25) due to increased intersystem crossing in the presence of the Fe3O4 NPs. The photophysical parameters: triplet (ΦT) quantum yields and the triplet-state lifetimes (τT), for ZnPc(COOH)4 linked to magnetic nanoparticles are presented in Table 2.
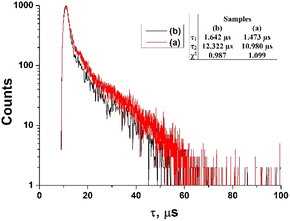 |
| Fig. 15 The fluorescence lifetimes of ZnPc(COOH)4/Fe3O4 in DMSO/phys.sol. 0.9% (a) and ZnPc(COOH)4/Fe3O4/chitosan in DMSO/H2O2/Ac.ac (b) solutions. | |
Table 2 Photophysical parameters: triplet quantum yields (ΦT) and the triplet-state lifetimes (τT) for all compounds
|
Solvent |
λabs, nm |
λemis, nm |
ΦT |
τT, μs |
ZnPc(COOH)4 |
DMSO/H2O |
697 |
765 |
0.25 |
11.63 |
ZnPc(COOH)4/chitosan |
DMSO/H2O |
699 |
775 |
0.27 |
11.31 |
ZnPc(COOH)4/Fe3O4 |
DMSO/phys.sol. 0.9% |
702 |
826 |
0.23 |
10.98 |
ZnPc(COOH)4/Fe3O4/chitosan |
DMSO/H2O2/Ac.ac |
702 |
784 |
0.56 |
12.32 |
So, we suppose that the surface interaction between the amino groups of the chitosan/Fe3O4 and the carboxylic groups of ZnPc(COOH)4 most probably forms an electrostatic interaction. In addition to the electrostatic interaction between charged surfaces of ZnPc(COOH)4 and chitosan/Fe3O4, coordination bonds between the Zn2+ ions of phthalocyanine and the oxygen atoms of chitosan/Fe3O4 can be formed.26,27 Also, hydrogen bonds between the nitrogen atoms of phthalocyanine and the hydrogen atoms of chitosan/Fe3O4 are also possible, as shown in the scheme presented in Fig. 16.
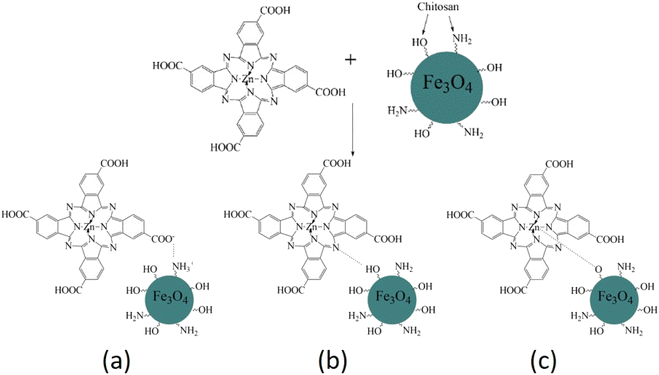 |
| Fig. 16 Scheme of the possible interactions (electrostatic (a), hydrogen (b) and coordination bonds (c)) of ZnPc(COOH)4 with the chitosan-functionalized Fe3O4 nanoparticles. | |
So, significant efforts have been made to develop the ZnPc(COOH)4/Fe3O4/chitosan composite that has strong absorption of long-wavelength light and a triplet quantum yield of 0.56 that can be promising for PDT. But further studies will continue to improve the triplet-state lifetime and the triplet quantum yield, and elucidate the physiochemical processes in this composite. Moreover, in vitro and in vivo studies are required to elucidate the PDT effects.
Conclusions
In this study we have developed:
(1) a soluble ZnPc(COOH)4 photosensitizer in DMSO/H2O, ratio 1
:
1, with high absorption at 702 nm, excitation/emission wavelengths of 615/765 nm and fluorescence lifetimes of 1.42 μs and 11.63 μs.
(2) Fe3O4/chitosan magnetic nanoparticles with a mean crystallite size of the nanoparticles up 14.80 nm using the suspension cross-linking technique.
(3) ZnPc(COOH)4 immobilized on chitosan-functionalized Fe3O4 nanoparticles through an immersion method with the aid of DMSO/H2O2/Ac.ac solution, exhibits higher triplet lifetimes of 1.6 μs and 12.3 μs.
The values of the triplet quantum yield (0.56) and the triplet-state lifetimes of ZnPc(COOH)4/Fe3O4/Ch make this composite a promising candidate for PDT.
Author contributions
Conceptualization: T. Potlog, S. Robu and I. Bulimestru; methodology: A. Popusoi and S. Robu; formal analysis: A. Popusoi; investigation: I. Lungu and A. Popusoi; data curation: A. Popusoi and I. Lungu; writing – original draft preparation: T. Potlog and S. Robu; writing – review and editing: I. Bulimestru, T. Potlog and S. Robu; supervision: T. Potlog. All authors have read and agreed to the published version of the manuscript.
Conflicts of interest
The authors declare no conflict of interest.
Acknowledgements
This research was supported by the 20.80009.5007.16 project of the Ministry of Education and Research of the Republic of Moldova. Also, profound thanks and appreciation to Research4Life.
Notes and references
- A. Brilkina, L. Dubasova, E. Sergeeva, A. Pospelov, N. Shilyagina, N. Shakhova and I. Balalaeva, Photobiological properties of phthalocyanine photosensitizers Photosens, Holosens and Phthalosens: A comparative in vitro analysis, J. Photochem. Photobiol., B, 2019, 191, 128–134 CrossRef CAS.
- M. Dimaano, C. Rozario, M. Nerandzic, C. Donskey, M. Lam, E. Baron and M. Hamblin, The Photodynamic Antibacterial Effects of Silicon Phthalocyanine (Pc) 4, Int. J. Mol. Sci., 2015, 16(12), 7851–7860 CrossRef CAS PubMed.
- S. Ghosh, K. Carter and J. Lovell, Liposomal Formulations of Photosensitizers, Biomaterials, 2019, 218, 119341 CrossRef CAS PubMed.
- D. Kessel and N. Oleinick, Cell Death Pathways Associated with Photodynamic Therapy: An Update, Photochem. Photobiol., 2018, 94(2), 213–218 CrossRef CAS PubMed.
- J. Huang, N. Chen and J. Huang, et al., Metal phthalocyanine as photosensitizer for photodynamic therapy (PDT)-preparation, characterization and anticancer activities of an amphiphilic phthalocyanine ZnPcS2P2, Sci. China, Ser. B: Chem., 2000, 44, 113–122 CrossRef.
- W. Liu, N. Chen, H. Jin, J. Huang, J. Wei, J. Bao and A. Wang, Intravenous repeated-dose toxicity study of ZnPcS2P2-based-photodynamic therapy in beagle dogs, Regul. Toxicol. Pharmacol., 2007, 47(3), 221–231 CrossRef CAS PubMed.
- J. Rak, P. Pouckova, J. Benes and D. Vetvicka, Drug Delivery Systems for Phthalocyanines for Photodynamic Therapy, Anticancer Res., 2019, 39(7), 3323–3339 CrossRef CAS PubMed.
- M. Miretti, C. Prucca, T. Tempesti and M. Baumgartner, Current Phthalocyanines Delivery Systems in Photodynamic Therapy: An Updated Review, Curr. Med. Chem., 2021, 28(26), 5339–5367 CrossRef CAS PubMed.
- X. Jia and L. Jia, Nanoparticles Improve Biological Functions of Phthalocyanine Photosensitizers Used for Photodynamic Therapy, Curr. Drug Metab., 2012, 13(8), 1119–1122 CrossRef CAS PubMed.
- B.-Y. Zheng, L. Wang, Q.-Y. Hu, J. Shi, M.-R. Ke and J.-D. Huang, Novel unsymmetrical silicon(IV) phthalocyanines as highly potent anticancer photosensitizers. Synthesis, characterization, and in vitro photodynamic activities, Dyes Pigm., 2020, 177, 108286 CrossRef CAS.
- C. Anine and A. Heidi, Aluminium(III) phthalocyanine chloride tetrasulphonate is an effective photosensitizer for the eradication of lung cancer stem cells, R. Soc. Open Sci., 2021, 8, 210148 CrossRef PubMed.
- I. Özçeşmeci, A. Tekin and A. Gül, Synthesis and aggregation behavior of zinc phthalocyanines substituted with bulky naphthoxy and phenylazonaphthoxy groups: An experimental and theoretical study, Synth. Met., 2014, 189, 100–110 CrossRef.
- J.-Y. Liu, J. Li, X. Yuan, W.-M. Wang and J.-P. Xue, In vitro photodynamic activities of zinc(II) phthalocyanines substituted with pyridine moieties, Photodiagn. Photodyn. Ther., 2016, 13, 341–343 CrossRef CAS PubMed.
- P. Sangaiya and R. Jayaprakash, A Review on Iron Oxide Nanoparticles and Their Biomedical Applications, J. Supercond. Novel Magn., 2018, 31, 3397–3413 CrossRef CAS.
- Y. Liu, T. Cui, Y. Li, Y. Zhao, Y. Ye, W. Wu and G. Tong, Effects of crystal size and sphere diameter on static magnetic and electromagnetic properties of monodisperse Fe3O4 microspheres, Mater. Chem. Phys., 2016, 173, 152–160 CrossRef CAS.
- R. Neha, A. Jaiswal, J. Bellare and N. Sahu, Synthesis of Surface Grafted Mesoporous Magnetic Nanoparticles for Cancer Therapy, J. Nanosci. Nanotechnol., 2017, 17(8), 5181–5188 CrossRef CAS.
- K. Mylkie, P. Nowak, P. Rybczynski and M. Ziegler-Borowska, Polymer-Coated Magnetite Nanoparticles for Protein Immobilization, Materials, 2021, 14(2), 248 CrossRef CAS PubMed.
- D. Eyre, Collagen cross-linking amino acids, Methods Enzymol., 1987, 144, 115–139 CrossRef CAS PubMed.
- G. Hojnik Podrepšek, Z. Knez and M. Leitgeb, Development of Chitosan Functionalized Magnetic Nanoparticles with Bioactive Compounds, Nanomaterials, 2020, 10(10), 1913 CrossRef PubMed.
- L. Luo, L. Zhu, Y. Xu, L. Shen, X. Wang, Y. Ding and D. Deng, Hydrogen peroxide biosensor based on horseradish peroxidase immobilized on chitosan-wrapped NiFe2O4 nanoparticles, Microchim. Acta, 2011, 174(1–2), 55–61 CAS.
- R. El-kharrag, S. Abdel Halim, A. Amin and Y. Greish, Synthesis and characterization of chitosan-coated magnetite nanoparticles using a modified wet method for drug delivery applications, Int. J. Polym. Mater. Polym. Biomater., 2019, 68(1–3), 73–82 CrossRef CAS.
- S. Chaki, T. Malek, M. Chaudhary, J. Tailor and M. Deshpande, Magnetite Fe3O4 nanoparticles synthesis by wet chemical reduction and their characterization, Adv. Nat. Sci.: Nanosci. Nanotechnol., 2015, 6(3), 035009 Search PubMed.
- L. He, L. Yao, F. Liu, B. Qin, R. Song and W. Huang, Magnetic Fe3O4@Chitosan Nanoparticle: Synthesis, Characterization and Application as Catalyst Carrier, J. Nanosci. Nanotechnol., 2010, 10(10), 6348–6355 CrossRef CAS PubMed.
- A. Khalil, et al., Study UV-visible and FTIR Characterization of ZnPc Dye using double solvent, J. Global Pharma Technol., 2020, 12(6), 210–216 CAS.
- A. Ogunsipe, D. Maree and T. Nyokong, Solvent effects on the photochemical and fluorescence properties of zinc phthalocyanine derivatives, J. Mol. Struct., 2003, 650(1–3), 131–140 CrossRef CAS.
- R. Mota, J. Lima, F. Denardin and E. Mazzetto, Nanomaterials Based on Fe3O4 and Phthalocyanines Derived from Cashew Nut Shell Liquid, Molecules, 2019, 24(18), 3284 CrossRef PubMed.
- T. Nyokong and E. Antunes, Influence of nanoparticle materials on the photophysical behavior of phthalocyanines, Coord. Chem. Rev., 2013, 257(15–16), 2401–2418 CrossRef CAS.
|
This journal is © The Royal Society of Chemistry 2022 |
Click here to see how this site uses Cookies. View our privacy policy here.