DOI:
10.1039/D2RA05655K
(Paper)
RSC Adv., 2022,
12, 34282-34292
A convenient catalytic method for preparation of new tetrahydropyrido[2,3-d]pyrimidines via a cooperative vinylogous anomeric based oxidation†
Received
7th September 2022
, Accepted 21st November 2022
First published on 30th November 2022
Abstract
In this study, a novel functionalized metal–organic frameworks MIL-125(Ti)-N(CH2PO3H2)2 was designed and synthesized via post-modification methodology. Then, MIL-125(Ti)-N(CH2PO3H2)2 as a mesoporous catalyst was applied for the synthesis of a wide range of novel tetrahydropyrido[2,3-d]pyrimidines as bioactive candidate compounds by one-pot condensation reaction of 3-(1-methyl-1H-pyrrol-2-yl)-3-oxopropanenitrile, 6-amino-1,3-dimethylpyrimidine-2,4(1H,3H)-dione and aromatic aldehydes at 100 °C under solvent-free condition. Interestingly, the preparation of tetrahydropyrido[2,3-d]pyrimidine was achieved via vinylogous anomeric based oxidation mechanism with a high yield and short reaction time.
1 Introduction
Nowadays, metal–organic frameworks (MOFs) have been known as a unique type of porous solid materials, because they have been utilized in different fields such as drug delivery, catalyst, photocatalyst, battery, super capacitor, sensor, gas storage, separation and adsorption of molecules.1–3 These materials consist of multifunctional organic materials bound to metal or metal clusters through coordinated components such as carboxylates to form crystalline materials with high surface area and high thermal stability.4–8 Among them, the post-modification of metal–organic frameworks can be carried out with metal, acid and basic groups for preparation of biological molecules, which make motivational enzymes attractive for catalytic processes.9–11 Thus, post-modification improves the performance of the structure of metal–organic frameworks (MOFs) by applying changes in surface, pore size, thermal and chemical stability.12–14 The structure of titanium (Ti) based metal–organic frameworks is shown in Fig. 1.15 The research team developed post-modification of metal–organic frameworks based on Cr, Al and Zr,16–20 glycoluril,21 mesoporous materials (SBA-15),22 melamine,23 and carbon quantum dots (CQDs)24 with phosphorus acid tags as a porous catalyst for the preparation of biological compounds.
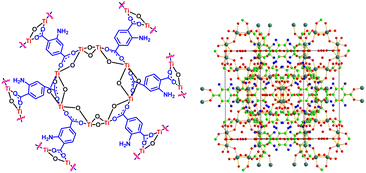 |
| Fig. 1 Structure of titanium-based metal–organic frameworks MIL-125(Ti)-NH2. | |
Heterocyclic compounds containing N and/or O heteroatoms show biological properties.25,26 Therefore, the attention of many scientists has been attracted to the synthesis of these compounds. Amongst them, pyrido[2,3-d]pyrimidines are a well-known group of these compounds that show great importance of biological properties like adenosine kinase inhibitors, anti-tumor, antifungal, antibacterial, anti-proliferative CDK2 inhibitor, antipyretic, anticonvulsant agents, and analgesic while being used in CNS depressant activity (Fig. 2).27–31 The “anomeric effect” (AE) explains stereo-electronic interactions in the synthesis of molecules containing at least one heteroatom.32 The term cooperative anomeric effect has been introduced for electron sharing from two and/or more heteroatoms lone pairs to one acceptor antibonding orbital another atom. When anomeric effect developed via double bonds has been named “vinylogous anomeric effect”. The “geminal anomeric effect” is compared with vinylogous anomeric effect (Fig. 3). In fact, the cooperative vinylogous anomeric effect is introduced for electron sharing via double bonds. Conversely, the geminal anomeric effect is introduced for electron donating and accepting from vicinal atoms.33 Recently, we have introduced a new concept entitled “cooperative vinylogous anomeric based oxidation” for the synthesis of heterocycle compounds via a susceptible intermediate in the course of reaction.34 In addition, this key phenomenon is observed in the oxidation/reduction mechanism and hydride transfer from NADPH/NADP+ and/or NADH/NAD+ systems.35–37
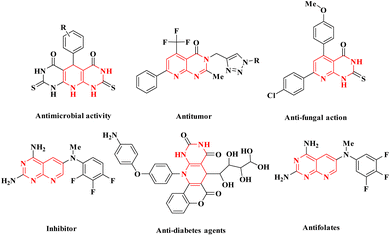 |
| Fig. 2 Structure of pyrido[2,3-d]pyrimidines as a pharmacological candidate. | |
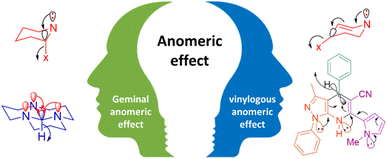 |
| Fig. 3 Comparison of geminal and vinylogues anomeric effect. | |
Herein, based on the approach of post-modification, we have designed and synthesized novel MIL-125(Ti)-N(CH2PO3H2)2 as an efficient functionalized catalyst. This catalyst was tested for the preparation of novel tetrahydropyrido[2,3-d]pyrimidines by one-pot condensation reaction of aldehydes, 6-amino-1,3-dimethylpyrimidine-2,4(1H,3H)-dione and 3-(1-methyl-1H-pyrrol-2-yl)-3-oxopropanenitrile via a cooperative vinylogous anomeric based oxidation mechanism (Scheme 1).
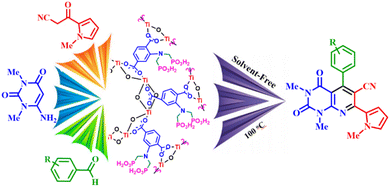 |
| Scheme 1 Synthesis of novel tetrahydropyrido[2,3-d]pyrimidines using MIL-125(Ti)-N(CH2PO3H2)2 as a porous catalyst. | |
2 Experimental
2.1 Materials and methods
All materials and solvents such as 2-amino terephthalic acid (NH2-H2BDC) (Merck, 95%), DMF (Merck, 99%), titanium tetraisopropanolate (TTIP) (Aldrich, 99.8%), methanol (Merck, 96%), ethanol (Merck, 99%), paraformaldehyde, phosphorous acid, p-toluene sulfonic acid (p-TSA), 6-amino-1,3-dimethylpyrimidine-2,4(1H,3H)-dione1-methyl-1H-pyrrole (Merck, 96%), 2-cyanoacetic acid were purchased and used without further purification.
2.2 Instrumental techniques
The (FT-IR) technique model device, (PerkinElmer Spectrum Version 10.02.00) was used to identify the functional groups. The morphology and microstructure of the different stages of synthesized catalyst were characterized using a SEM technique (TESCAN MIRA-II (Czechia)). EDS was carried out by the model (TESCAN MIRA-II (Czechia)). The thermal stability of the prepared porous catalyst was determined by a thermogravimetric analyzer/derivative thermogravimetry (TGA/DTG, TGA2, Mettler Toledo) at a heating rate of 10 °C min−1 under N2 atmosphere. BET technique with model device (BELSORP-mini-II) was used to determine the surface area and pore size of different stages of the synthesized catalyst and XRD patterns of the different stages synthesized catalyst were detected by X-ray diffractometer (PHILIPS PW1730 (Netherlands)).
2.3 General procedure for the preparation of MIL-125(Ti)-N(CH2PO3H2)2
Firstly, MIL-125(Ti)-NH2 was synthesized according to the literature survey.38 In the following, a mixture of MIL-125(Ti)-NH2, (0.5 g), paraformaldehyde (3 mmol, 0.09 g), phosphorous acid (2 mmol, 0.164 g) and p-TSA (0.02 g) was refluxed in EtOH (50 mL) as a solvent for 12 h. After this time, the obtained solid was filtered by centrifugation (1000 rpm, 10 min), and washed with EtOH (2 × 10 mL) at room temperature. The precipitate was dried under vacuum oven at 80 °C for 12 h to give MIL-125(Ti)-N(CH2PO3H2)2 (Scheme 2).
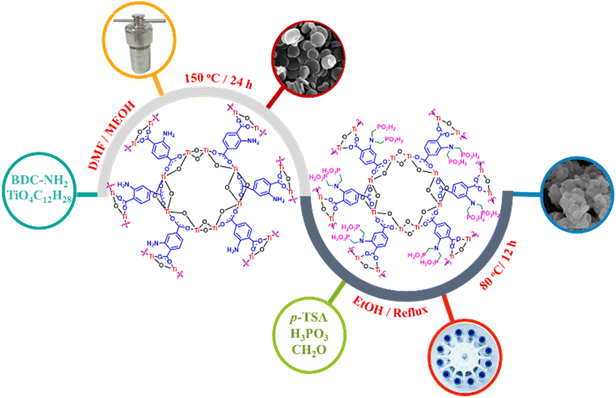 |
| Scheme 2 Synthesis of MIL-125(Ti)-NH2 and MIL-125(Ti)-N(CH2PO3H2)2. | |
2.4 General procedure for the synthesis of novel tetrahydropyrido[2,3-d]pyrimidines using MIL-125(Ti)-N(CH2PO3H2)2 as a porous catalyst
Initially, 3-(1-methyl-1H-pyrrol-2-yl)-3-oxopropanenitrile was prepared according to the literature survey (Scheme 3).39 Then, in a 25 mL round-bottomed flask a mixture of aryl aldehydes (1 mmol), 6-amino-1,3-dimethylpyrimidine-2,4(1H,3H)-dione (1 mmol, 0.155 g), 3-(1-methyl-1H-pyrrol-2-yl)-3-oxopropanenitrile (1 mmol, 0.148 g) and MIL-125(Ti)-N(CH2PO3H2)2 (10 mg) as a porous catalyst was stirred at 100 °C under solvent-free condition. After the completion of the reactions which were monitored by the TLC technique (n-hexane
:
ethyl acetate; 7
:
3). Then, the described catalyst was separated from the reaction mixture by centrifugation (1000 rpm) after adding hot EtOH (20 mL) as a solvent. Finally, after the evaporation of the solvent at room temperature, the pure product was obtained by washing with hot ethanol and water (Scheme 3).
 |
| Scheme 3 Preparation of 3-(1-methyl-1H-pyrrol-2-yl)-3-oxopropanenitrile. | |
2.5 Spectral data
2.5.1 5-(4-Chlorophenyl)-1,3-dimethyl-7-(1-methyl-1H-pyrrol-2-yl)-2,4-dioxo-1,2,3,4 tetrahydropyrido[2,3-d]pyrimidine-6-carbonitrile (A1). Yellow solid; mp: 248–250 °C; FT-IR (KBr, cm−1): 3113, 2998, 2949, 2219, 1709, 1663. 1H NMR (400 MHz, DMSO-d6) δppm 7.56 (d, J = 8.5 Hz, 2H), 7.37 (d, J = 8.5 Hz, 2H), 7.25 (t, J = 1.6 Hz, 1H), 7.20 (dd, J = 4.1, 1.6 Hz, 1H), 6.26 (dd, J = 4.1, 2.5 Hz, 1H), 4.01 (s, 3H), 3.65 (s, 3H), 3.16 (s, 3H).
2.5.2 1,3-Dimethyl-7-(1-methyl-1H-pyrrol-2-yl)-2,4-dioxo-5-(p-tolyl)-1,2,3,4-tetrahydropyrido[2,3-d]pyrimidine-6-carbonitrile (A2). Yellow solid; mp: 228–230 °C; FT-IR (KBr, cm−1): 3123, 2960, 2873, 2220, 1717, 1674. 1H NMR (400 MHz, DMSO-d6) δppm 7.39 (d, J = 7.9 Hz, 2H), 7.34 (t, J = 1.6 Hz, 1H), 7.32 (s, 1H), 7.31–7.28 (m, 2H), 6.36 (dd, J = 4.0, 2.6 Hz, 1H), 4.11 (s, 3H), 3.75 (s, 3H), 3.26 (s, 3H), 2.52 (s, 3H). 13C NMR (101 MHz, DMSO-d6) δppm 158.8, 158.54, 154.4, 151.8, 150.6, 137.7, 133.7, 130.7, 128.4, 127.4, 127.2, 117.2, 117.0, 108.2, 104.9, 101.6, 37.30, 30.32, 28.11, 20.95.
2.5.3 5-(4-Methoxyphenyl)-1,3-dimethyl-7-(1-methyl-1H-pyrrol-2-yl)-2,4-dioxo-1,2,3,4-tetrahydropyrido[2,3-d]pyrimidine-6-carbonitrile (A3). Yellow solid; mp: 230–233 °C; FT-IR (KBr, cm−1): 3369, 3142, 2981, 2219, 1709, 1662. 1H NMR (400 MHz, DMSO-d6) δppm 7.27 (d, J = 8.7 Hz, 2H), 7.24 (t, J = 1.6 Hz, 1H), 7.19 (dd, J = 4.0, 1.6 Hz, 1H), 7.03 (d, J = 8.7 Hz, 2H), 6.25 (dd, J = 4.0, 2.6 Hz, 1H), 4.01 (s, 3H), 3.85 (s, 3H), 3.65 (s, 3H), 3.17 (s, 3H). 13C NMR (101 MHz, DMSO-d6) δppm 159.4, 158.6, 158.6, 154.4, 151.9, 150.6, 130.7, 129.2, 128.5, 127.2, 117.2, 117.2, 113.2, 108.2, 104.9, 101.8, 55.0, 37.3, 30.3, 28.1.
2.5.4 1,3-Dimethyl-7-(1-methyl-1H-pyrrol-2-yl)-2,4-dioxo-5-phenyl-1,2,3,4-tetrahydropyrido[2,3-d]pyrimidine-6-carbonitrile (A4). Yellow solid; mp: 228–230 °C; FT-IR (KBr, cm−1): 3116, 2951, 2213, 1714, 1670. 1H NMR (400 MHz, DMSO-d6) δppm 7.50–7.46 (m, 3H), 7.32 (dd, J = 6.6, 2.9 Hz, 2H), 7.24 (t, J = 1.6 Hz, 1H), 7.19 (dd, J = 4.1, 1.6 Hz, 1H), 6.26 (dd, J = 4.0, 2.6 Hz, 1H), 4.02 (s, 3H), 3.66 (s, 3H), 3.16 (s, 3H). 13C NMR (101 MHz, DMSO-d6) δppm 158.6, 158.5, 154.4, 151.8, 150.7, 136.7, 130.8, 128.4, 127.8, 127.4, 127.2, 117.2, 116.9, 108.3, 104.8, 101.5, 37.3, 30.3, 28.1.
2.5.5 1,3-Dimethyl-7-(1-methyl-1H-pyrrol-2-yl)-2,4-dioxo-5-(m-tolyl)-1,2,3,4-tetrahydropyrido[2,3-d]pyrimidine-6-carbonitrile (A5). Yellow solid; mp: 180–182 °C; FT-IR (KBr, cm−1): 3118, 2920, 2219, 1714, 1668. 1H NMR (400 MHz, DMSO-d6) δppm 7.37 (t, J = 7.6 Hz, 1H), 7.28 (d, J = 7.7 Hz, 1H), 7.24 (t, J = 1.9 Hz, 1H), 7.19 (dd, J = 4.1, 1.7 Hz, 1H), 7.13–7.08 (m, 2H), 6.25 (dd, J = 4.1, 2.5 Hz, 1H), 4.01 (s, 3H), 3.65 (s, 3H), 3.16 (s, 3H), 2.37 (s, 3H). 13C NMR (101 MHz, DMSO-d6) δppm 158.8, 158.4, 154.3, 151.8, 150.7, 136.8, 136.7, 130.7, 129.0, 127.8, 127.6, 127.2, 124.5, 117.2, 116.9, 108.3, 104.8, 101.5, 37.3, 30.3, 28.1, 21.0.
2.5.6 5-(4-Fluorophenyl)-1,3-dimethyl-7-(1-methyl-1H-pyrrol-2-yl)-2,4-dioxo-1,2,3,4-tetrahydropyrido[2,3-d]pyrimidine-6-carbonitrile (A6). Yellow solid; mp: 289–290 °C; FT-IR (KBr, cm−1): 3119, 2955, 2213, 1716, 1662. 1H NMR (400 MHz, DMSO-d6) δppm 7.41–7.37 (m, 2H), 7.35–7.30 (m, 2H), 7.25 (t, J = 1.7 Hz, 1H), 7.20 (dd, J = 4.1, 1.7 Hz, 1H), 6.26 (dd, J = 4.1, 2.5 Hz, 1H), 4.01 (s, 3H), 3.66 (s, 3H), 3.17 (s, 3H). 13C NMR (101 MHz, DMSO-d6) δppm 163.4, 161.0, 158.6, 157.7, 154.3, 151.8, 150.6, 133.0, 130.9, 129.9, 127.2, 117.3, 117.0, 115.0, 114.8, 108.3, 105.0, 101.6, 37.3, 30.3, 28.1.
2.5.7 5-(4-Hydroxyphenyl)-1,3-dimethyl-7-(1-methyl-1H-pyrrol-2-yl)-2,4-dioxo-1,2,3,4-tetrahydropyrido[2,3-d]pyrimidine-6-carbonitrile (A7). Yellow solid; mp: >300 °C; FT-IR (KBr, cm−1): 3306, 3080, 2942, 2223, 1727, 1653. 1H NMR (400 MHz, DMSO-d6) δppm 9.72 (s, 1H), 7.23 (t, J = 1.6 Hz, 1H), 7.18 (dd, J = 4.1, 1.7 Hz, 1H), 7.14 (d, J = 8.6 Hz, 2H), 6.84 (d, J = 8.6 Hz, 2H), 6.25 (dd, J = 4.0, 2.6 Hz, 1H), 4.00 (s, 3H), 3.64 (s, 3H), 3.17 (s, 3H). 13C NMR (101 MHz, DMSO-d6) δppm 159.1, 158.5, 157.8, 154.4, 151.9, 150.7, 130.6, 129.2, 127.3, 126.8, 117.3, 117.2, 114.6, 108.2, 104.9, 101.9, 37.2, 30.3, 28.
2.5.8 5-(2-Methoxyphenyl)-1,3-dimethyl-7-(1-methyl-1H-pyrrol-2-yl)-2,4-dioxo-1,2,3,4-tetrahydropyrido[2,3-d]pyrimidine-6-carbonitrile (A8). Yellow solid; mp: 205–208 °C; FT-IR (KBr, cm−1): 3447, 2219, 1718, 1665. 1H NMR (400 MHz, DMSO-d6) δppm 7.24–7.14 (t, J = 7.1 Hz, 1H), 7.18 (m, 4H), 7.10–7.03 (m, 1H), 6.25 (s, 1H), 4.02 (s, 3H), 3.73 (s, 3H), 3.65 (s, 3H), 3.17 (s, 3H). 13C NMR (101 MHz, DMSO-d6) δppm 158.3, 156.3, 155.5, 154.4, 151.8, 150.6, 130.8, 130.2, 128.5, 127.2, 125.5, 120.3, 117.1, 116.9, 111.1, 108.3, 105.3, 101.7, 55.5, 37.4, 30.3, 28.0.
2.5.9 5-(4-Bromophenyl)-1,3-dimethyl-7-(1-methyl-1H-pyrrol-2-yl)-2,4-dioxo-1,2,3,4-tetrahydropyrido[2,3-d]pyrimidine-6-carbonitrile (A9). Yellow solid; mp: 266–268 °C; FT-IR (KBr, cm−1): 3116, 2952, 2221, 1715, 1669. 1H NMR (400 MHz, DMSO-d6) δppm 7.70 (d, J = 8.4 Hz, 2H), 7.30 (d, J = 8.5 Hz, 2H), 7.25 (t, J = 1.7 Hz, 1H), 7.20 (dd, J = 4.1, 1.7 Hz, 1H), 6.26 (dd, J = 4.1, 2.5 Hz, 1H), 4.01 (s, 3H), 3.65 (s, 3H), 3.16 (s, 3H). 13C NMR (101 MHz, DMSO-d6) δppm 158.6, 157.3, 154.4, 151.8, 150.6, 136.0, 130.9, 130.9, 129.7, 127.2, 122.0, 117.3, 116.9, 108.4, 104.7, 101.2, 37.3, 30.3, 28.1.
2.5.10 1,3-Dimethyl-7-(1-methyl-1H-pyrrol-2-yl)-5-(4-nitrophenyl)-2,4-dioxo-1,2,3,4-tetrahydropyrido[2,3-d]pyrimidine-6-carbonitrile (A10). Yellow solid; mp: 233–235 °C; FT-IR (KBr, cm−1): 3427, 2948, 2219, 1712, 1664. 1H NMR (400 MHz, DMSO-d6) δppm 8.37 (d, J = 8.8 Hz, 2H), 7.66 (d, J = 8.8 Hz, 2H), 7.27 (t, J = 1.6 Hz, 1H), 7.22 (dd, J = 4.1, 1.6 Hz, 1H), 6.27 (dd, J = 4.1, 2.5 Hz, 1H), 4.03 (s, 3H), 3.67 (s, 3H), 3.16 (s, 3H). 13C NMR (101 MHz, DMSO-d6) δppm 158.7, 156.3, 154.4, 151.8, 150.6, 147.4, 143.9, 131.2, 129.1, 127.1, 123.2, 117.5, 116.7, 108.5, 104.6, 100.6, 37.4, 30.3, 28.1.
2.5.11 5-(2,4-Dichlorophenyl)-1,3-dimethyl-7-(1-methyl-1H-pyrrol-2-yl)-2,4-dioxo-1,2,3,4-tetrahydropyrido[2,3-d]pyrimidine-6-carbonitrile (A11). Yellow solid; mp: 210–213 °C; FT-IR (KBr, cm−1): 3109, 2942, 2219, 1722, 1659. 1H NMR (400 MHz, DMSO-d6) δppm 7.83 (d, J = 2.0 Hz, 1H), 7.60 (dd, J = 8.3, 2.0 Hz, 1H), 7.40 (d, J = 8.3 Hz, 1H), 7.28 (t, J = 1.7 Hz, 1H), 7.24 (dd, J = 4.1, 1.7 Hz, 1H), 6.28 (dd, J = 4.1, 2.5 Hz, 1H), 4.04 (s, 3H), 3.66 (s, 3H), 3.18 (s, 3H). 13C NMR (101 MHz, DMSO-d6) δppm 158.4, 154.5, 154.5, 151.9, 150.5, 134.9, 134.1, 131.7, 131.4, 130.4, 128.6, 127.5, 127.0, 117.5, 116.4, 108.6, 104.8, 100.6, 37.5, 30.3, 28.1.
2.5.12 5-(3-Hydroxyphenyl)-1,3-dimethyl-7-(1-methyl-1H-pyrrol-2-yl)-2,4-dioxo-1,2,3,4-tetrahydropyrido[2,3-d]pyrimidine-6-carbonitrile (A12). Yellow solid; mp: 156–158 °C; FT-IR (KBr, cm−1): 3116, 2945, 2216, 1714, 1667. 1H NMR (400 MHz, DMSO-d6) δppm 9.58 (s, 1H), 7.26 (dd, J = 13.4, 5.2 Hz, 2H), 7.18 (dd, J = 4.1, 1.6 Hz, 1H), 6.85 (dd, J = 8.1, 1.7 Hz, 1H), 6.71–6.66 (m, 2H), 6.25 (dd, J = 4.0, 2.5 Hz, 1H), 4.01 (s, 3H), 3.65 (s, 3H), 3.17 (s, 3H). 13C NMR (101 MHz, DMSO-d6) δppm 158.6, 158.3, 156.8, 154.3, 151.8, 150.7, 137.9, 130.7, 129.1, 127.2, 117.9, 117.2, 116.9, 115.3, 114.2, 108.3, 104.8, 101.4, 37.3, 30.3, 28.1.
2.5.13 5-(2-Chlorophenyl)-1,3-dimethyl-7-(1-methyl-1H-pyrrol-2-yl)-2,4-dioxo-1,2,3,4-tetrahydropyrido[2,3-d]pyrimidine-6-carbonitrile (A13). Yellow solid; mp: >300 °C; FT-IR (KBr, cm−1): 3113, 2949, 2998, 2219, 1709, 1663. 1H NMR (400 MHz, DMSO-d6) δppm 7.63–7.60 (m, 1H), 7.54–7.45 (m, 2H), 7.34 (dd, J = 7.4, 1.8 Hz, 1H), 7.28 (t, J = 1.6 Hz, 1H), 7.22 (dd, J = 4.1, 1.6 Hz, 1H), 6.28 (dd, J = 4.1, 2.5 Hz, 1H), 4.04 (s, 3H), 3.67 (s, 3H), 3.17 (s, 3H). 13C NMR (101 MHz, DMSO-d6) δppm 158.3, 155.6, 154.5, 151.9, 150.5, 135.8, 131.2, 130.4, 130.2, 129.0, 128.9, 127.2, 127.0, 117.4, 116.4, 108.54, 37.5, 30.3, 28.1.
2.5.14 5-(4-Isopropylphenyl)-1,3-dimethyl-7-(1-methyl-1H-pyrrol-2-yl)-2,4-dioxo-1,2,3,4-tetrahydropyrido[2,3-d]pyrimidine-6-carbonitrile (A14). Yellow solid; mp: 190–192 °C; FT-IR (KBr, cm−1): 3110, 2955, 2853, 2219, 1712, 1663. 1H NMR (400 MHz, DMSO-d6) δppm 7.35 (d, J = 8.1 Hz, 2H), 7.27–7.23 (m, J = 8.0 Hz, 3H), 7.18 (dd, J = 4.1, 1.6 Hz, 1H), 6.25 (dd, J = 4.0, 2.6 Hz, 1H), 4.01 (s, 3H), 3.65 (s, 3H), 3.16 (s, 3H), 2.99 (hep, J = 6.9 Hz, 1H), 1.29 (d, J = 6.9 Hz, 6H). 13C NMR (101 MHz, DMSO-d6) δppm 158.8, 158.5, 154.4, 151.9, 150.7, 148.4, 134.0, 130.7, 127.5, 127.2, 125.7, 117.2, 117.0, 108.3, 104.9, 101.6, 37.2, 33.1, 30.3, 28.1, 23.7.
2.5.15 5-(3-Ethoxy-4-hydroxyphenyl)-1,3-dimethyl-7-(1-methyl-1H-pyrrol-2-yl)-2,4-dioxo-1,2,3,4-tetrahydropyrido[2,3-d]pyrimidine-6-carbonitrile (A15). Yellow solid; mp: 246–248 °C; FT-IR (KBr, cm−1): 3106, 3060, 2942, 2218, 1715, 1670. 1H NMR (400 MHz, DMSO-d6) δppm 9.21 (s, 1H), 7.23 (t, J = 1.9 Hz, 1H), 7.18 (dd, J = 4.0, 1.6 Hz, 1H), 6.93–6.85 (m, 2H), 6.74 (dd, J = 8.1, 1.9 Hz, 1H), 6.25 (dd, J = 4.0, 2.6 Hz, 1H), 4.04–3.98 (m, 5H), 3.64 (s, 3H), 3.17 (s, 3H), 1.33 (t, J = 6.9 Hz, 3H). 13C NMR (101 MHz, DMSO-d6) δppm 158.9, 158.5, 154.3, 151.8, 150.7, 147.3, 145.9, 130.6, 127.3, 127.2, 120.7, 117.3, 117.2, 115.0, 113.7, 108.2, 105.0, 101.9, 63.8, 37.2, 30.3, 28.1, 14.5.
2.5.16 1,3-Dimethyl-7-(1-methyl-1H-pyrrol-2-yl)-5-(naphthalen-1-yl)-2,4-dioxo-1,2,3,4-tetrahydropyrido[2,3-d]pyrimidine-6-carbonitrile (A16). Yellow solid; mp: 212–214 °C; FT-IR (KBr, cm−1): 3113, 2945, 2219, 1709, 1663. 1H NMR (400 MHz, DMSO-d6) δppm 8.04 (d, J = 8.3 Hz, 2H), 7.65–7.60 (m, 1H), 7.57–7.53 (m, 1H), 7.43 (d, J = 3.8 Hz, 2H), 7.39 (dd, J = 7.0, 0.7 Hz, 1H), 7.26 (t, J = 1.6 Hz, 1H), 7.18 (dd, J = 4.1, 1.6 Hz, 1H), 6.25 (dd, J = 4.1, 2.5 Hz, 1H), 4.07 (s, 3H), 3.71 (s, 3H), 3.07 (s, 3H). 13C NMR (101 MHz, DMSO-d6) δppm 158.4, 150.7, 136.8, 134.2, 130.7, 128.6, 127.7, 127.2, 126.2, 118.6, 117.2, 108.9, 108.3, 104.9, 37.2, 30.3, 28.1.
2.5.17 5,5′-(1,4-Phenylene)bis(1,3-dimethyl-7-(1-methyl-1H-pyrrol-2-yl)-2,4-dioxo-1,2,3,4-tetrahydropyrido[2,3-d]pyrimidine-6-carbonitrile) (A17). Yellow solid; mp: >300 °C; FT-IR (KBr, cm−1): 2951, 2928, 2216, 1716, 1667. 1H NMR (400 MHz, DMSO-d6) δppm 7.46 (s, 4H), 7.26–7.23 (m, J = 3.7 Hz, 4H), 6.28–6.25 (m, 2H), 4.03 (s, 6H), 3.68 (s, 6H), 3.23 (s, 3H), 3.18 (s, 3H). 13C NMR (101 MHz, DMSO-d6) δppm 155.1, 154.9, 153.1, 152.2, 151.4, 150.9, 143.5, 131.8, 131.7, 131.3, 131.0, 128.2, 127.2, 117.9, 117.6, 116.9, 115.9, 108.7, 108.4, 107.8, 106.5, 104.3, 99.7, 98.6, 37.8, 37.4, 30.4, 29.7, 28.1.
3 Results and discussion
Development of “anomeric based oxidation” concept, from synthesis of heterocycle compounds via a susceptible intermediate in the course of reaction is our main research demand because this key phenomenon is observed in the oxidation/reduction mechanism and hydride transfer from NADPH/NADP+ and/or NADH/NAD+ biological systems.35–37 With this aim, the described porous metal–organic framework containing phosphorous acid groups MIL-125(Ti)-N(CH2PO3H2)2 as a porous catalyst was synthesized and applied for the preparation of tetrahydropyrido[2,3-d]pyrimidines via a cooperative vinylogous anomeric based oxidation mechanism. To shed light on more details, the structure of MIL-125(Ti)-N(CH2PO3H2)2 as a porous material catalyst was thoroughly approved by various analyses; FT-IR, X-ray diffraction analysis (XRD), energy-dispersive X-ray spectroscopy (EDX), scanning electron microscope (SEM), N2 absorption and desorption, thermal gravimetric (TGA) and derivative thermogravimetry (DTG).
FT-IR analysis of MIL-125(Ti)-NH2 and MIL-125(Ti)-N(CH2PO3H2)2 were compered in Fig. 4. The bands at 3384 and 3462 cm−1 indicates the symmetric and asymmetric vibrations of the NH2 groups in MIL-125(Ti)-NH2. The broad peak at 2800–3600 cm−1 is related to the OH of PO3H2 functional groups in MIL-125(Ti)-N(CH2PO3H2)2. The absorption bands at 1659 cm−1 in MIL-125(Ti)-NH2 are assigned to C
O bond stretching of carboxylic acid group. Also, the absorption bands at 1031 and 1086 cm−1 are assigned to P–O bond stretching. Furthermore, the absorption band at 775 cm−1 is related to Ti metal. The changes between the FT-IR spectrums of MIL-125(Ti)-NH2 and MIL-125(Ti)-N(CH2PO3H2)2 verified the successful post-modification.
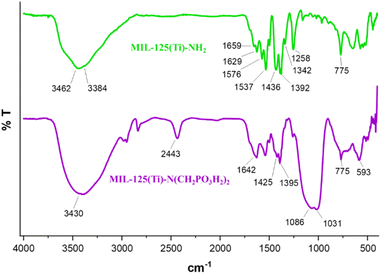 |
| Fig. 4 FT-IR spectra of MIL-125(Ti)-NH2 and MIL-125(Ti)-N(CH2PO3H2)2 as a porous catalyst. | |
The XRD patterns of MIL-125(Ti)-NH2 and MIL-125(Ti)-N(CH2PO3H2)2 were described in Fig. 5. As shown in Fig. 5, the pattern of peak of MIL-125(Ti)-NH2 is similar to the literature references.40–42 Also, the XRD results of post-modification with phosphorous acid groups is almost unchanged with MIL-125(Ti)-NH2. According to the XRD profile, the morphology and structure of the catalyst remained constant at different stages of synthesis.
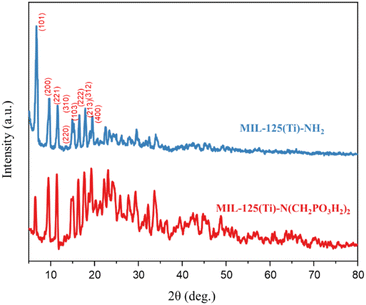 |
| Fig. 5 XRD patterns of MIL-125(Ti)-NH2 and MIL-125(Ti)-N(CH2PO3H2)2 as a porous catalyst. | |
The crystal scaffolds of MIL-125(Ti)-NH2 and MIL-125(Ti)-N(CH2PO3H2)2 were checked out by energy dispersive X-ray analysis (EDX) (Fig. 6). According to the EDX analysis approved the existence elements structure of C, N, and Ti in MIL-125(Ti)-NH2 and P, O, N, and Ti in MIL-125(Ti)-N(CH2PO3H2)2 respectively.
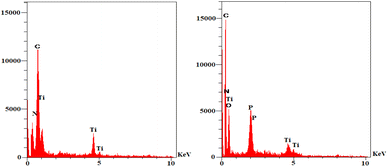 |
| Fig. 6 EDX analysis of MIL-125(Ti)-NH2 and MIL-125(Ti)-N(CH2PO3H2)2 as a porous catalyst. | |
In another study, the morphology of MIL-125(Ti)-NH2 and its post-modification were investigated by scanning electron microscope (SEM) (Fig. 7). As shown in Fig. 7a–c, the uniform tablet-like form and smooth surface shape of MIL-125(Ti)-NH2 exhibit, which is similar to the literature reference.40 Also, the morphology of MIL-125(Ti)-NH2 after post-modification with phosphorus acid tags is unchanged and stable (Fig. 7d–f). As shown, the obtained XRD pattern confirmed the resulting image from SEM analysis.
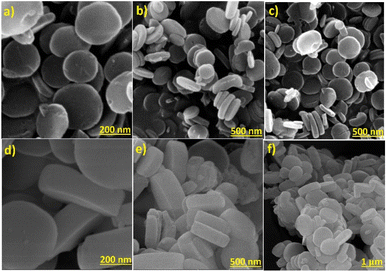 |
| Fig. 7 SEM images of MIL-125(Ti)-NH2 (a–c) and MIL-125(Ti)-N(CH2PO3H2)2 (d–f) as a porous catalyst. | |
The textural properties of MIL-125(Ti)-NH2 and MIL-125(Ti)-N(CH2PO3H2)2 were studied by N2 adsorption–desorption isotherms (Fig. 8a). The absence of the hysteresis loop indicates the absence of mesoporous in the samples. The calculated surface areas based on the BET equation are 1328 and 554 m2 g−1 for MIL-125(Ti)-NH2 and MIL-125(Ti)-N(CH2PO3H2)2 respectively. Furthermore, the total pore volumes for these samples are 0.5663 cm3 g−1 and 0.3856 cm3 g−1, respectively. The pore size distribution of both MIL-125(Ti)-NH2 and MIL-125(Ti)-N(CH2PO3H2)2 based on the BJH method are shown in (Fig. 8b). As shown in this figure the pore size of both samples are less than 2 nm revealing the presence of micropores in the samples.
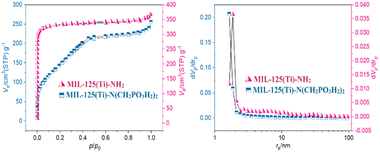 |
| Fig. 8 N2 absorption–desorption isotherm and pore size distribution based on BJH method for MIL-125(Ti)-NH2 (▲) and MIL-125(Ti)-N(CH2PO3H2)2 (■) as a porous catalyst. | |
In another investigation, thermal gravimetric (TG) and derivative thermogravimetry (DTG) were applied for thermal and behavioral stability of MIL-125(Ti)-N(CH2PO3H2)2 (Fig. 9). According to Fig. 9, the weight loss around 25–100 °C is related to the evaporation and removal solvents (organic and H2O) in the synthesis of the catalyst. Also, the weight loss around 450 °C is suggested to the decomposition and breaking of organic section (bond of N–C–PO3H2) of metal–organic frameworks (MOFs). Therefore, obtained data show thermal stability excellent of MIL-125(Ti)-N(CH2PO3H2)2, which can be used as a porous catalyst for organic reaction.
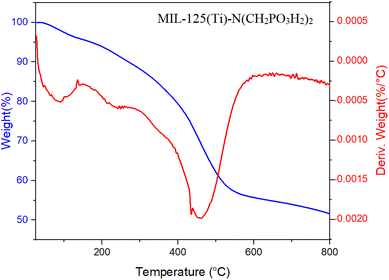 |
| Fig. 9 TG and DTG analysis of MIL-125(Ti)-N(CH2PO3H2)2 as a porous catalyst. | |
After the design, preparation, and characterization of MIL-125(Ti)-N(CH2PO3H2)2 as an acidic catalyst, the catalytic behavior of novel porous metal–organic framework was examined in the synthesis of tetrahydropyrido[2,3-d]pyrimidine derivatives via a cooperative vinylogous anomeric based oxidation. The above-mentioned catalyst was achieved one pat reaction of 4-choloro benzaldehyde (1 mmol, 0.140 g), 6-amino-1,3-dimethylpyrimidine-2,4(1H,3H)-dione (1 mmol, 0.155 g) and 3-(1-methyl-1H-pyrrol-2-yl)-3-oxopropanenitrile (1 mmol, 0.148 g) as a model reaction. The optimized reaction data is listed in Table 1. In this table, the model reaction was studied by using several solvents such as H2O, EtOH, CH2Cl2, CHCl3, EtOAc, DMF, MeOH and CH3CN (10 mL) in the presence of 10 mg of MIL-125(Ti)-N(CH2PO3H2)2 under reflux condition (Table 1, entries 10–17). Also, the model reaction was studied by using different amounts of catalysts (Table 1, entry 6–9) and temperatures (Table 1, entry 1–5). Considering the data reaction obtained (yield and time), the best results for the preparation of tetrahydropyrido[2,3-d]pyrimidines were achieved under solvent-free conditions at 100 °C and in the presence of MIL-125(Ti)-N(CH2PO3H2)2 (10 mg) as a porous catalyst (Table 1, entry 2).
Table 1 Effect of different amounts of catalysts, temperature and solvent (5 mL) in the synthesis of novel tetrahydropyrido[2,3-d]pyrimidines
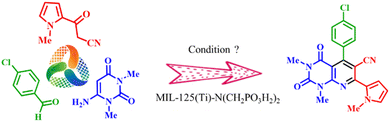
|
Entry |
Solvent |
Catalyst (mg) |
Temp. (°C) |
Time (min) |
Yield (%) |
1 |
— |
10 |
110 |
40 |
83 |
2 |
— |
10 |
100 |
35 |
90 |
3 |
— |
10 |
90 |
50 |
82 |
4 |
— |
10 |
50 |
100 |
30 |
5 |
— |
10 |
25 |
120 |
— |
6 |
— |
20 |
100 |
35 |
85 |
7 |
— |
15 |
100 |
40 |
70 |
8 |
— |
5 |
100 |
100 |
55 |
9 |
— |
— |
100 |
180 |
— |
10 |
H2O |
10 |
Reflux |
180 |
— |
11 |
EtOH |
10 |
Reflux |
100 |
70 |
12 |
CH2Cl2 |
10 |
Reflux |
180 |
— |
13 |
CHCl3 |
10 |
Reflux |
100 |
— |
14 |
EtOAc |
10 |
Reflux |
120 |
10 |
15 |
DMF |
10 |
100 |
150 |
72 |
16 |
MeOH |
10 |
Reflux |
120 |
45 |
17 |
CH3CN |
10 |
Reflux |
120 |
55 |
After optimizing the reaction conditions for the preparation of novel tetrahydropyrido[2,3-d]pyrimidines, the performance and application of MIL-125(Ti)-N(CH2PO3H2)2 as a porous metal–organic framework catalyst was further evaluated. The obtained results are summarized in Table 2. According to this table, the synthesis of tetrahydropyrido[2,3-d]pyrimidines were carried out under optimal conditions by using various aromatic aldehydes, including electron-bearing groups, electron-accepting groups, and halogen groups. The obtained results show that the yield of the products is high and the time of conducting the experiments is short. These results show the suitable catalytic performance of MIL-125(Ti)-N(CH2PO3H2)2, which can be extended to synthesis other organic compounds.
Table 2 Synthesis of tetrahydropyrido[2,3-d]pyrimidine derivatives using MIL-125(Ti)-N(CH2PO3H2)2 as a porous catalysta
Reaction conditions: a mixture of aryl aldehydes (1 mmol), 6-amino-1,3-climethylpyrimidine-2,4(1H,3H)-dione (1 mmol, 0.155 g), 3-(1-methyl-1H-pyrrol-2-y1)-3-oxopropanenitrile (1 mmol, 0.148 g) and MIL-125(Ti)-N(CH2PO3H2)2 (10 mg) under solvent-free condition at 100 °C. |
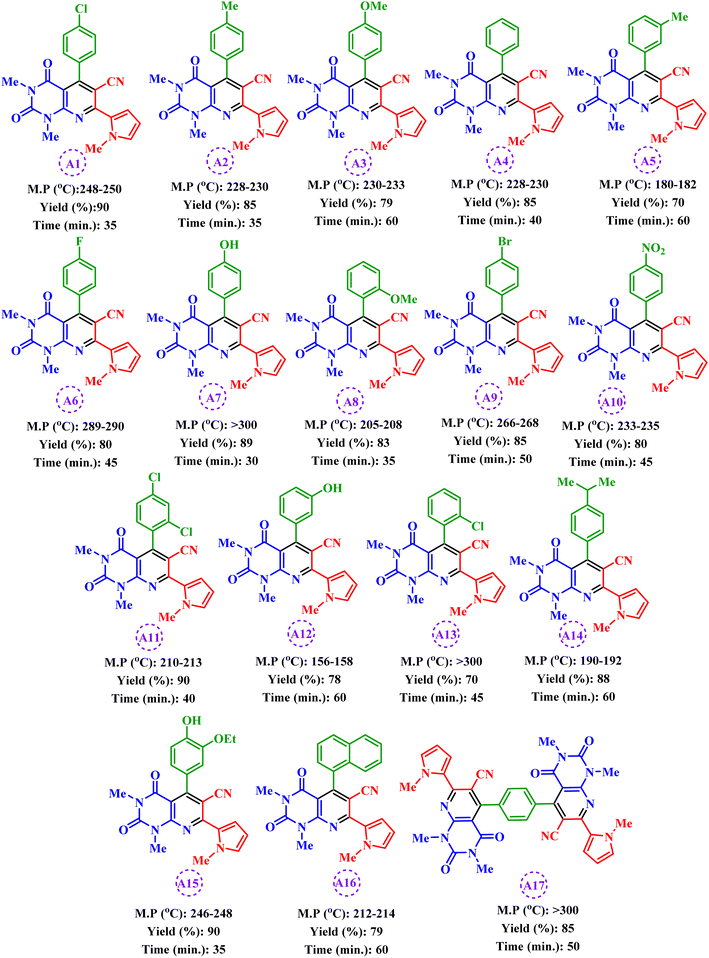 |
In the suggested mechanism, the aldehyde is activated by the catalyst and reacts with 3-(1-methyl-1H-pyrrol-2-yl)-3-oxopropanenitrile, which has been converted to its tautomeric form by the catalyst, and intermediate (I) is created. Intermediate (I) as a Michael acceptor is activated by the catalyst and is attacked by the uracil nucleophile. The intermediate (II) produced in this step is converted to intermediate (III) by proton transfer and tautomerization. Intermediate (III) is converted into intermediate (IV) by performing an intramolecular cyclization reaction and losing another H2O molecule. In the next step, intermediate (III) is converted into intermediate (IV) by performing an intramolecular cyclization reaction and losing another H2O molecule. In the next step, intermediate (IV) via “a cooperative geminal-vinylogous anomeric affect” through simultaneously electron sharing from phenyl group and lone pair electrons of N atoms through C
C bonds into the antibonding of C–H bond causes the releasing of hydride which has been named “cooperative geminal-vinylogous anomeric based oxidation” mechanism in the presence and absence of oxygen pathways A and B in Scheme 4 (in the main manuscript text) respectively. During this mechanism, hydrogen molecule (–H2) or hydrogen peroxide molecule (–H2O2) whose oxygen is supplied from the air is released. The above-mentioned concept has been reviewed comprehensively.34,43 To investigate the activation of aldehyde by catalyst, p-chloro benzaldehyde was activated with MIL-125(Ti) and MIL-125(Ti)-N(CH2PO3H2)2 at room temperature. Then the FT-IR spectra of the reaction mixtures were studied. The absorption band of C
O of the p-chloro benzaldehyde at 1692 cm−1 was changed to 1694 and 1702 cm−1 in the presence of MIL-125(Ti) and MIL-125(Ti)-N(CH2PO3H2)2 respectively (Fig. S1, see in ESI†).
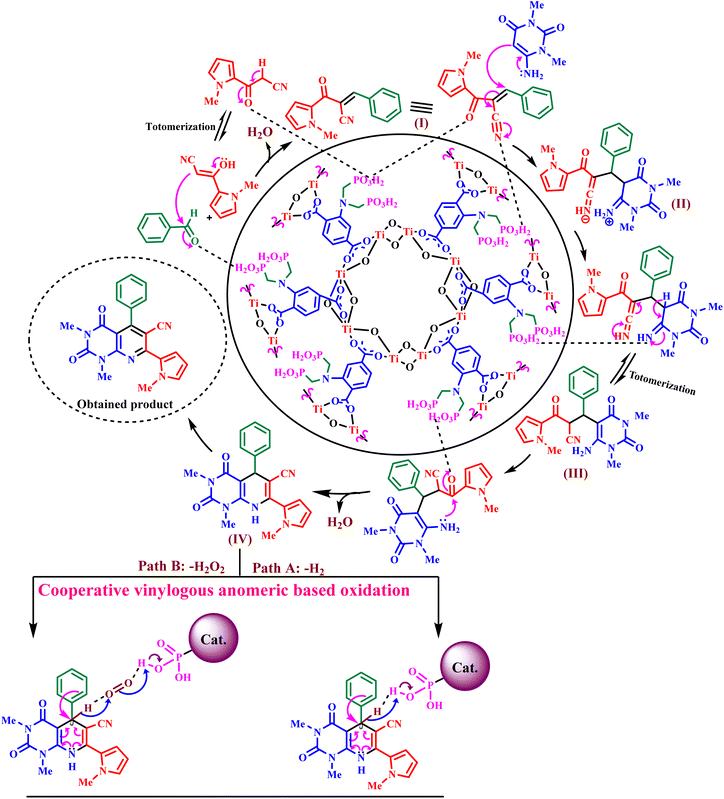 |
| Scheme 4 Plausible mechanism in the synthesis of tetrahydropyrido[2,3-d]pyrimidine derivatives. | |
To evaluate reactivity of catalyst, the reaction of 4-choloro benzaldehyde (1 mmol, 0.140 g), 6-amino-1,3-dimethylpyrimidine-2,4(1H,3H)-dione (1 mmol, 0.155 g) and 3-(1-methyl-1H-pyrrol-2-yl)-3-oxopropanenitrile (1 mmol, 0.148 g) as a model reaction was evaluated by MIL-125(Ti)-N(CH2PO3H2)2 and other catalyst such as organic, inorganic and solid acids (Table 3). As shown in Table 3, the MIL-125(Ti)-N(CH2PO3H2)2 is the best performance for the synthesis of tetrahydropyrido[2,3-d]pyrimidines. Also, the recyclability of MIL-125(Ti)-N(CH2PO3H2)2 was checked out in the model reaction. The results show that the MIL-125(Ti)-N(CH2PO3H2)2 has the potential to be recycled and reused up to 4 times without significant decreasing its catalytic activity (Fig. 10).
Table 3 Evaluation of various catalyst for the synthesis of novel tetrahydropyrido[2,3-d]pyrimidines in comparison with MIL-125(Ti)-N(CH2PO3H2)2
Entry |
Catalysta |
Amount of catalyst |
Time (min) |
Yield (%) |
The reaction of 4-choloro benzaldehyde (1 mmol, 0.140 g), 6-amino-1,3-dimethylpyrimidine-2,4(1H,3H)-dione (1 mmol, 0.155 g) and 3-(1-methyl-1H-pyrrol-2-yl)-3-oxopropanenitrile (1 mmol, 0.148 g) at 100 °C under solvent-free condition. |
1 |
p-TSA |
10 mol% |
100 |
20 |
2 |
NaOH |
10 mol% |
120 |
30 |
3 |
Et3N |
10 mol% |
120 |
25 |
4 |
GTMPA21 |
10 mol% |
100 |
43 |
5 |
MIL-53(Al)-N(CH2PO3H2)2 (ref. 18 and 19) |
10 mg |
60 |
75 |
6 |
CQDs-N(CH2PO3H2)2 (ref. 24) |
10 mg |
80 |
60 |
7 |
APVPB44 |
10 mg |
120 |
35 |
8 |
GTBSA45 |
10 mol% |
180 |
65 |
9 |
Fe3O4@Co(BDC)-NH2 (ref. 46) |
10 mg |
110 |
53 |
10 |
[PVI-SO3H]FeCl4 (ref. 47) |
10 mg |
100 |
40 |
11 |
SSA48,49 |
10 mg |
80 |
35 |
12 |
MIL-125(Ti)-NH2 |
10 mg |
120 |
52 |
13 |
PO3H3 |
10 mol% |
90 |
40 |
14 |
MIL-125(Ti)-N(CH2PO3H2)2this work |
10 mg |
35 |
90 |
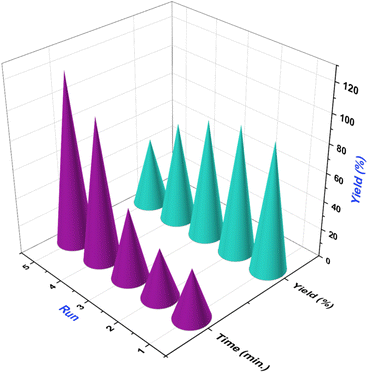 |
| Fig. 10 Reusability of MIL-53(Al)-N(CH2PO3H2)2 for the synthesis of novel tetrahydropyrido[2,3-d]pyrimidines. | |
4 Conclusions
In this paper, we reported new metal–organic frameworks (MOFs) containing phosphorous acid tags namely MIL-125(Ti)-N(CH2PO3H2)2 as a porous catalyst. This mesoporous catalyst was characterized by several techniques such as FT-IR, EDX, XRD, SEM, N2 adsorption–desorption isotherms, BJH/BJH and TG/DTG analysis. Then, its catalytic application in the synthesis of new series of tetrahydropyrido[2,3-d]pyrimidines via a cooperative vinylogous anomeric-based oxidation mechanism. Easy separation of catalyst from mixture reaction, clean profile of reaction and widespread new synthesis of biologically active with good to excellent yields are most important of described work.
Conflicts of interest
The authors declare no competing financial interests.
Acknowledgements
We thank the Bu-Ali Sina University and Iran National Science Foundation (INSF) (Grant Number: 4012835) for financial support.
References
- A. R. Millward and O. M. Yaghi, J. Am. Chem. Soc., 2005, 127, 17998–17999 CrossRef CAS.
-
(a) H. Sepehrmansourie, Iran. J. Catal., 2021, 11, 207–215 CAS;
(b) H. Sepehrmansourie, M. Zarei, M. A. Zolfigol, S. Kalhor and H. Shi, Mol. Catal., 2022, 531, 112634 CrossRef CAS.
-
(a) M. J. Kalmutzki, C. S. Diercks and O. M. Yaghi, Adv. Mater., 2018, 30, 1704304 CrossRef PubMed;
(b) H. Sepehrmansourie, H. Alamgholiloo, N. Noroozi Pesyan and M. A. Zolfigol, Appl. Catal., B, 2022, 321, 122082 CrossRef.
- J. Lee, O. K. Farha, J. Roberts, K. A. Scheidt, S. T. Nguyen and J. T. Hupp, Chem. Soc. Rev., 2009, 38, 1450–1459 RSC.
- L. Liu, Y. Zhou, S. Liu and M. Xu, ChemElectroChem, 2018, 5, 6–19 CrossRef CAS.
- K. Huang, B. Wang, S. Guo and K. Li, Angew. Chem., 2018, 57, 13892–13896 CrossRef CAS.
- F. Ghamari, D. Raoufi, S. Alizadeh, J. Arjomandi and D. Nematollahi, J. Mater. Chem. A, 2021, 9, 15381–15393 RSC.
- X. Zhang, T. Kitao, D. Piga, R. Hongu, S. Bracco, A. Comotti, P. Sozzani and T. Uemura, Chem. Sci., 2020, 11, 10844–10849 RSC.
- N. Ottman, L. Ruokolainen, A. Suomalainen, H. Sinkko, P. Karisola, J. Lehtimäki, M. Lehto, I. Hanski, H. Alenius and N. Fyhrquist, J. Allergy Clin. Immunol., 2019, 143, 1198–1206 CrossRef CAS PubMed.
-
(a) X. Kong, H. Deng, F. Yan, J. Kim, J. A. Swisher, B. Smit, O. M. Yaghi and J. A. Reimer, Science, 2013, 341, 882–885 CrossRef CAS;
(b) H. Sepehrmansourie, M. Zarei, M. A. Zolfigol, S. Babaee, S. Azizian and S. Rostamnia, Sci. Rep., 2022, 12, 14145 CrossRef CAS PubMed;
(c) E. Tavakoli, H. Sepehrmansourie, M. Zarei, M. A. Zolfigol, A. Khazaei and M. Hosseinifard, New J. Chem., 2022, 46, 19054–19061 RSC.
- F. Wang, C. Wang, Z. Yu, K. Xu, X. Li and Y. Fu, Polyhedron, 2016, 105, 49–55 CrossRef CAS.
- S. L. Griffin and N. R. Champness, Coord. Chem. Rev., 2020, 414, 213295 CrossRef CAS.
- D. Banerjee and J. B. Parise, Cryst. Growth Des., 2011, 11, 4704–4720 CrossRef CAS.
- H. L. Nguyen, J. Phys.: Energy, 2021, 3, 021003 CAS.
- A. P. Smalley, D. G. Reid, J. C. Tan and G. O. Lloyd, CrystEngComm, 2013, 15, 9368–9371 RSC.
- A. M. Naseri, M. Zarei, S. Alizadeh, S. Babaee, M. A. Zolfigol, D. Nematollahi, J. Arjomandi and H. Shi, Sci. Rep., 2021, 11, 16817 CrossRef CAS PubMed.
- H. Sepehrmansouri, M. Zarei, M. A. Zolfigol, A. R. Moosavi-Zare, S. Rostamnia and S. Moradi, Mol. Catal., 2020, 481, 110303 CrossRef CAS.
- S. Kalhor, M. Zarei, M. A. Zolfigol, H. Sepehrmansourie, D. Nematollahi, S. Alizadeh, H. Shi and J. Arjomandi, Sci. Rep., 2021, 11, 19370 CrossRef CAS.
- S. Babaee, M. Zarei, H. Sepehrmansourie, M. A. Zolfigol and S. Rostamnia, ACS Omega, 2020, 5, 6240–6249 CrossRef CAS PubMed.
- F. Jalili, M. Zarei, M. A. Zolfigol and A. Khazaei, RSC Adv., 2022, 12, 9058–9068 RSC.
-
(a) S. Moradi, M. A. Zolfigol, M. Zarei, D. A. Alonso and A. Khoshnood, ChemistrySelect, 2018, 3, 3042–3047 CrossRef CAS;
(b) B. Danishyar, H. Sepehrmansourie, M. Zarei, M. A. Zolfigol, M. A. As' Habi and Y. Gu, Polycyclic Aromat. Compd., 2022, 1–21 CrossRef.
- F. Jalili, M. Zarei, M. A. Zolfigol, S. Rostamnia and A. R. Moosavi-Zare, Microporous Mesoporous Mater., 2020, 294, 109865 CrossRef CAS.
- J. Afsar, M. A. Zolfigol, A. Khazaei, M. Zarei, Y. Gu, D. A. Alonso and A. Khoshnood, Mol. Catal., 2020, 482, 110666 CrossRef CAS.
- M. Mohammadi Rasooll, M. Zarei, M. A. Zolfigol, H. Sepehrmansourie, A. Omidi, M. Hasani and Y. Gu, RSC Adv., 2021, 11, 25995–26007 RSC.
- G. Jubete, R. Puig de la Bellacasa, R. Estrada-Tejedor, J. Teixidó and J. I. Borrell, Molecules, 2019, 24, 4161 CrossRef PubMed.
- M. N. Yousif, A. R. El-Gazzar and M. M. El-Enany, Mini-Rev. Org. Chem., 2021, 18, 43–54 CrossRef CAS.
- C. Kurumurthy, P. S. Rao, P. S. Rao, B. Narsaiah, L. Velatooru, R. Pamanji and J. V. Rao, Eur. J. Med. Chem., 2011, 46, 3462–3468 CrossRef CAS.
- A. Bazgir, M. M. Khanaposhtani, R. Ghahremanzadeh and A. A. Soorki, C. R. Chim., 2009, 12, 1287–1295 CrossRef CAS.
- V. Cody, J. Pace, O. A. Namjoshi and A. Gangjee, Acta Crystallogr., Sect. F: Struct. Biol. Commun., 2015, 71, 799–803 CrossRef CAS.
- A. Gangjee, O. A. Namjoshi, S. Raghavan, S. F. Queener, R. L. Kisliuk and V. Cody, J. Med. Chem., 2013, 56, 4422–4441 CrossRef CAS.
- Z. Toobaei, R. Yousefi, F. Panahi, S. Shahidpour, M. Nourisefat, M. M. Doroodmand and A. Khalafi-Nezhad, Carbohydr. Res., 2015, 411, 22–32 CrossRef CAS.
-
(a) E. Juaristi and G. Cuevas, Tetrahedron, 1992, 48, 5019–5087 CrossRef CAS;
(b) V. Alabugin, L. Kuhn, N. V. Krivoshchapov, P. Mehaffy and M. G. Medvedev, Chem. Soc. Rev., 2021, 50, 10212 RSC.
-
(a) I. V. Alabugin, Stereoelectronic effects: a bridge between structure and reactivity, John Wiley & Sons, 2016 CrossRef;
(b) I. V. Alabugin, L. Kuhn, M. G. Medvedev, N. V. Krivoshchapov, V. A. Vil, I. A. Yaremenko, P. Mehaffy, M. Yarie, A. O. Terent'ev and M. A. Zolfigol, Chem. Soc. Rev., 2021, 50, 10253 RSC.
- M. Yarie, Iran. J. Catal., 2017, 7, 85–88 CAS.
- C. B. Bai, N. X. Wang, Y. Xing and X. W. Lan, Synlett, 2017, 28, 402–414 CAS.
- T. He, R. Shi, Y. Gong, G. Jiang, M. Liu, S. Qian and Z. Wang, Synlett, 2016, 27, 1864–1869 CrossRef CAS.
- G. Hamasaka, H. Tsuji and Y. Uozumi, Synlett, 2015, 26, 2037–2041 CrossRef CAS.
- S. N. Kim, J. Kim, H. Y. Kim, H. Y. Cho and W. S. Ahn, Catal. Today, 2013, 204, 85–93 CrossRef CAS.
- H. M. Al-Matar, A. Y. Adam, K. D. Khalil and M. H. Elnagdi, Arkivoc, 2012, 6, 1–15 Search PubMed.
- Y. Zhao, W. Cai, J. Chen, Y. Miao and Y. Bu, Front. Chem., 2019, 7, 789 Search PubMed.
- R. Zhang, G. Li and Y. Zhang, Photochem. Photobiol. Sci., 2017, 16, 996–1002 CrossRef CAS.
- M. Nasalevich, R. Becker, E. Ramos-Fernandez, S. Castellanos, S. Veber, M. Fedin, F. Kapteijn, J. Reek, J. Van Der Vlugt and J. Gascon, Energy Environ. Sci., 2015, 8, 364–375 RSC.
- M. Yarie, Iran. J. Catal., 2020, 10, 79–83 CAS.
- E. Noroozizadeh, A. R. Moosavi-Zare, M. A. Zolfigol, M. Zarei, R. Karamian, M. Asadbegy, S. Yari and S. H. Moazzami Farida, J. Iran. Chem. Soc., 2018, 15, 471–481 CrossRef CAS.
- M. Zarei, H. Sepehrmansourie, M. A. Zolfigol, R. Karamian and S. H. Moazzami Farida, New J. Chem., 2018, 42, 14308–14317 RSC.
- H. Sepehrmansourie, M. Zarei, M. A. Zolfigol, S. Babaee and S. Rostamnia, Sci. Rep., 2021, 11, 5279 CrossRef CAS PubMed.
- H. Sepehrmansourie, M. Zarei, R. Taghavi and M. A. Zolfigol, ACS Omega, 2019, 4, 17379–17392 CrossRef CAS PubMed.
- M. A. Zolfigol, Tetrahedron, 2001, 57, 9509–9511 CrossRef CAS.
- H. Sepehrmansourie, Iran. J. Catal., 2020, 10, 175–179 CAS.
|
This journal is © The Royal Society of Chemistry 2022 |
Click here to see how this site uses Cookies. View our privacy policy here.