DOI:
10.1039/D2RA05158C
(Paper)
RSC Adv., 2022,
12, 28207-28216
A Ferrier glycosylation/cis-dihydroxylation strategy to synthesize Leishmania spp. lipophosphoglycan-associated βGal(1,4)Man disaccharide†
Received
17th August 2022
, Accepted 27th September 2022
First published on 4th October 2022
Abstract
The Galβ(1→4)Man disaccharide, found in the cell surface lipophosphoglycan (LPG) of Leishmania species, has been synthesized by a Ferrier glycosylation/cis-dihydroxylation strategy. This stereoselective method proved efficient for synthesizing the target saccharide in good yield. In addition, we prepared two clickable O-glycoside and phospho-glycoside versions of Galβ(1→4)Man to enable conjugation to protein carriers for further immunological and antibody-binding studies.
Introduction
Leishmaniasis is a poverty-associated parasitic disease that imposes a heavy burden on the public health systems of least developed countries, particularly those of South Asia, East Africa, and Latin America. The protozoan Leishmania spp. (Trypanosomatidae), the etiological agent of leishmaniasis, is a vector-borne obligate intracellular parasite transmitted by hematophagous sandflies. More than 20 human-infective Leishmania species have been described to date, together with 70 different types of sandflies (Phlebotomus and Lutzomyia) identified as vectors.1,2 Depending on the infecting Leishmania species, individuals can present one of the three disease phenotypes: self-resolvent cutaneous leishmaniasis (CL), disfiguring mucocutaneous leishmaniasis (MCL), or potentially fatal visceral leishmaniasis (VL).2 Although leishmaniasis is an underreported infection, current estimates for CL range from 700
000 to 1.2 million worldwide cases each year, and yearly VL cases are about 100
000.3 Available anti-leishmaniasis treatments include pentavalent antimonials, liposomal amphotericin B, miltefosine, and paromomycin.4 These drugs are effective in treating leishmaniasis; however, side effects, poor patient compliance, and parasite resistance motivate the search for new and improved drugs. Moreover, the lack of an effective anti-leishmaniasis vaccine to prevent and treat the infection is a critical need.
A dense glycocalyx covers the Leishmania spp. cell membrane playing a pivotal role in the parasite's survival and infectivity. The chemical structure of this sugar coat varies according to the parasite species, its life cycle stage, and the host type.5 Fig. 1A shows the structure of Leishmania's lipophosphoglycan (LPG), the predominant membrane-glycan in promastigotes, the human-infective form.6 Its structure comprises a phosphatidylinositol anchor, a conserved oligosaccharide core, a linear phosphoglycan (PG), and a neutral mannose-rich oligosaccharide cap. The chemical structure of LPG varies among Leishmania species. The differences are typically found in the structures of the capping oligosaccharides and the type and sequence of the branching sugars connected to the conserved linear PG, a phospho-polysaccharide comprising the [-6)-β-D-Gal(1→4)α-D-Man-(1→PO3O] repeating unit. For example, in Leishmania donovani, the etiological agent of VL in the Old World, promastigotes PG is unsubstituted and remains linear.7 In contrast, in L. infantum (L. chagasi), the causative agent of New World's VL, PG carries Glcβ(1→3) substitutions.8
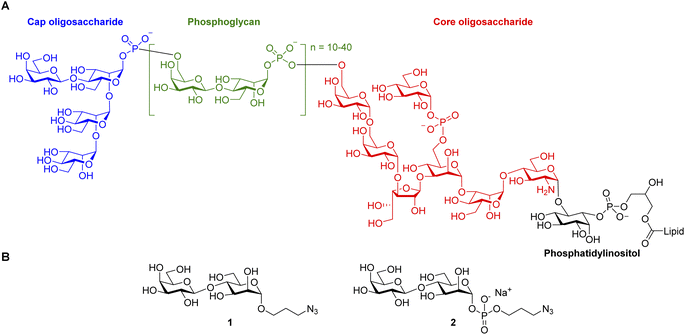 |
| Fig. 1 (A) Structure of Leishmania donovani lipophosphoglycan; (B) structures of the clickable Galβ(1→4)Man O- and phospho-glycosides 1 and 2 synthesized in this work. | |
Given its role as an immune determinant, LPG is an attractive target for developing glycoconjugate vaccine candidates against leishmaniasis.9 The groups of Nikolaev, Vishwakarma, and Seeberger have accomplished the synthesis of different LPG fragments and proved the suitability of protein-conjugated LPG subunits as immunogens.10 However, despite the promising preliminary immunization results,11 an effective anti-leishmaniasis vaccine remains elusive.
Because of our interest in anti-parasitic immunotherapies12 and the development of virus-like particles (VLP) glycoconjugates to boost anti-carbohydrate immune responses,13 we have centered our attention on LPG to explore VLP-conjugated anti-leishmaniasis vaccine candidates. Here, we report an expeditious synthetic route to prepare Galβ(1→4)Man, a disaccharide exclusively found in the linear phosphoglycan and the capping oligosaccharides of Leishmania's LPG. Two clickable versions of Galβ(1→4)Man (Fig. 1B), namely 3-azidopropyl 4-O-β-D-galactopyranosyl-α-D-mannopyranoside (1) and 3-azidopropyl 4-O-β-D-galactopyranosyl-α-D-mannopyranosyl phosphate (2), were prepared to enable conjugation to the protein carrier and subsequent performance of immunological studies.
Results and discussion
Synthesis of Galβ(1→4)Man disaccharide
Scheme 1 shows the synthetic strategy to prepare Galβ(1→4)Man. We envisioned the combination of the Ferrier glycosylation14 and osmium-catalyzed cis-dihydroxylation,15 both high-yielding and stereoselective reactions, as a convenient sequence to synthesize the target disaccharide from the inexpensive and readily available starting material D-lactose per-acetate. First. we synthesized the Ferrier glycosyl donor hexa-O-acetyl-D-lactal 3 in 42% yield by adjusting the procedure of Zhao et al.16 which involves forming the lactosyl bromide from lactose per-acetate followed by reduction with Zn dust. The subsequent Ferrier glycosylation14,17 of benzyl alcohol with lactal 3, catalyzed by BF3·Et2O, afforded a mixture of 2,3-unsaturated benzyl glycosides 4α and 4β (α/β = 6
:
1) in 92% yield. The osmium-catalyzed cis-dihydroxylation of the anomeric mixture gave diols 5 (major product) and 6 in excellent yield, which were readily separated by normal-phase flash chromatography. The treatment of diols 5 and 6 with Ac2O in pyridine yielded the respective per-acetates 7 and 8. Given the convenient peak separation in their 1H NMR spectra, we performed the stereochemical configuration analysis of the oxidation adducts on their respective per-acetylated derivatives.
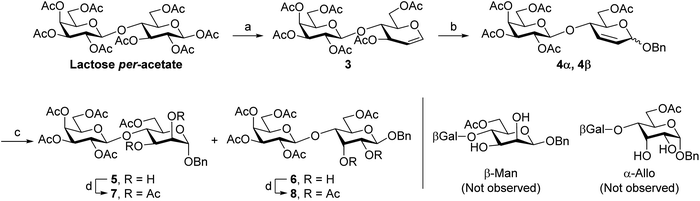 |
| Scheme 1 Synthesis of Galβ(1→4)αMan disaccharide via Ferrier glycosylation/cis-dihydroxylation sequence: (a) synthesis of D-lactal: (i) HBr (34% in AcOH), CH2Cl2, 0 °C to room temperature. (ii) Zn, NaH2PO4 (sat.), acetone, (42% two steps); (b) BnOH, BF3·Et2O, dioxane, room temperature. (66%, α/β = 6 : 1); (c) OsO4, NMO, H2O, acetone, room temperature (92%); (d) Ac2O, pyridine, CH2Cl2, room temperature (88% for 7, 81% for 8). | |
In the 1H NMR spectrum of acetate 7, derived from the major cis-dihydroxylation product 5, proton H3 (δ 5.32 ppm) appears as a doublet of doublets with coupling constants 3JH3–H4 and 3JH3–H2 of 8.8 and 3.6 Hz respectively.
The large value for the 3JH3–H4 unambiguously identifies a trans-diaxial correlation between H3 and H4. The anomeric proton H1 (δ 4.74 ppm) appears as a doublet with a small 3JH1–H2 (1.8 Hz). These attributes are consistent with an α-mannose configuration.18 In acetate 8, derived from the minor cis-dihydroxylation product 6, H3 (δ 5.76 ppm) appears as a triplet with 3JH3–H4 and 3JH3–H2 values of ∼2.5 Hz, indicating a cis correlation with H4. The proton H1 (δ 4.83 ppm) showed as a doublet with a large 3JH1–H2 value (8.3 Hz), confirming a β-allose configuration for the minor oxidation product.
The results show that the osmium-catalyzed cis-dihydroxylation of disaccharide derivatives 2,3-dideoxy-4-O-β-D-galactopyranosyl-D-erythro-hex-2-enopyranosides exhibit the same stereospecificity reported for the oxidation of the monosaccharide 2,3-dideoxy-D-erythro-hex-2-enopyranoside congeners: oxidation of 2,3-unsaturated α-glycosides leads to the formation of α-mannosides whereas the oxidation of the respective β-anomers yields β-allosides.19
Since the formation of the β-glycoside in the Ferrier glycosylation limits the yield of the target Galβ(1→4)Man disaccharide 5, we attempted to improve the α-stereoselectivity of this reaction by testing different solvents (MeCN, dioxane, THF, and Et2O), common Lewis acid catalysts (FeCl3,20 TMSOTf,21 and I2 (ref. 22)), and Lewis acids reported to afford high α-glycoside yields in Ferrier glycosylations (Y(OTf)3,23 Gd(OTf)3 (ref. 24)). However, in our hands, these attempts did not significantly improve the α-selectivity of this step using D-lactal 3 as a glycosyl donor.
Synthesis of azide-functionalized Galβ(1→4)αMan O-glycoside 1. To facilitate immobilization onto surfaces and conjugation with protein carriers for further immunological studies, we installed a clickable25 azide-functionalized aglycone on Galβ(1→4)αMan (Scheme 2). The Pd-catalyzed hydrogenolysis of benzyl glycoside 7, afforded the respective anomeric alcohol 9 in quantitative yield. The subsequent treatment of this intermediate with Cl3CCN and DBU in MeCN generated the respective imidate 10 which was coupled to 3-azidopropanol by TMSOTf-catalyzed imidate activation in Et2O at 0 °C. The azide-functionalized glycoside 11 was obtained in 70% yield as a single anomer. The removal of the acetyl protective groups via Zemplén transesterification26 yielded the target azide-functionalized Galβ(1→4)Man α-glycoside 1.
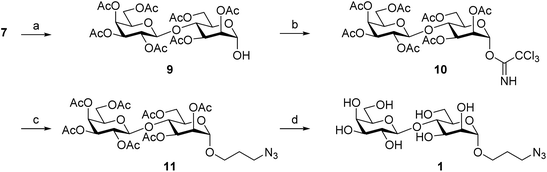 |
| Scheme 2 Synthesis of clickable Galβ(1→4)Man O-glycoside. (a) H2, Pd/C, EtOH (96%); (b) Cl3CCN, DBU, CH2Cl2 (68%); (c) 3-azidopropanol, TMSOTf, CH2Cl2 (70%); (d) NaOMe, MeOH, Amberlite IR120 (H+) (97%). | |
Synthesis of azide-functionalized Galβ(1→4)αMan phospho-glycoside 2. The H-phosphonate 12 was prepared by treating the anomeric alcohol 9 with a freshly prepared solution of the phosphorylating reagent triimidazolylphosphine (PIm3),27 made by reacting PCl3 with imidazole in MeCN (Scheme 3). The addition of alcohol 9 to a PIm3 solution forms the respective phosphorodiamidite intermediate which upon hydrolysis with triethylammonium bicarbonate buffer (TEAB, pH 7.2) affords the triethylammonium Galβ(1→4)αMan H-phosphonate 12 in 42% yield. The successful H-phosphonylation was confirmed by the 31P NMR spectrum of 12, which showed a characteristic H-phosphonate signal at δP −0.10 ppm (dd, 1JP–H = 638 Hz, 1JP–H1 = 9.8 Hz). After confirming H-phosphonate identity, we coupled 12 to 3-azidopropanol in THF using pivaloyl chloride as a coupling reagent. The H-phosphonate diester intermediate, that is formed in this reaction, was oxidized in situ with I2 and H2O/pyridine to afford the respective phosphate diester 13 in 30% yield. The 31P NMR spectrum of 13 showed phosphorus at δP −3.09 (d, 3JP–H1 = 6.9 Hz) confirming the H-phosphonate oxidation to phosphate. Removal of the acetyl protecting groups afforded the target azide-functionalized Galβ(1→4)αMan phospho-glycoside 2.
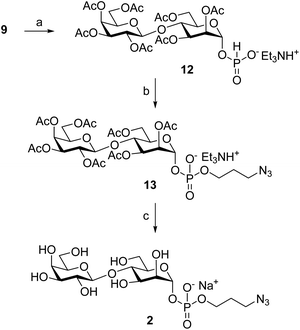 |
| Scheme 3 Synthesis of azide-functionalized Galβ(1→4)αMan phospho-glycoside 2. (a) PCl3, imidazole, Et3N, MeCN (42%); (b) (i) 3-azidopropanol, PivCl, THF; (ii) I2, H2O/pyridine (5 : 95) (30% two steps); (c) NaOMe, MeOH, Amberlite IR 120(+) (67%). | |
Conclusions
We have synthesized the βGal(1→4)αMan disaccharide, the phospho-polysaccharide repeating unit found in the cell surface lipophosphoglycan of all Leishmania species. The Ferrier glycosylation/cis-dihydroxylation sequence proved efficient in affording the target βGal(1→4)Man core in a stereoselective fashion from readily available lactose per-acetate. Two clickable versions of βGal(1→4)Man, azide-functionalized O-glycoside and phospho-glycoside, were also prepared to enable coupling to protein carriers and immobilization onto surfaces for further immunological and binding studies.
Experimental
General information
D-Lactose and all reagents were purchased to the highest purity available and used without previous purification. Yields refer to isolated yields after chromatographic purification and anomeric ratios were determined from the 1H NMR spectra of reaction crudes. Reactions were monitored by thin-layer chromatography (TLC) using SiliCycle SiliaPlate glass-backed TLC 250 mm with F-254 UV indicator. TLC visualization was performed under short wave UV light (254 nm) and charring with 5% H2SO4 in CH3OH. Normal phase flash chromatography was performed using SiliCycle SiliaFlash P60 silica gel (40–63 μm) and mixtures of EtOAc-hexanes and CH2Cl2–MeOH (ACS-grade solvents) as eluents. 1D and 2D NMR spectra were recorded on a Bruker Avance 400 spectrometer at 298 K. 1H NMR chemical shifts are reported in parts per million vs. tetramethylsilane (TMS) internal standard (δH 0.00 ppm). 13C{1H} NMR chemical shifts are reported in parts per million vs. the residual peak of the solvent.28 LCMS data were collected in a Waters e2695 HPLC system coupled to a Waters Acquity QDa single quadrupole mass detector. HRMS data for new compounds were collected in a Waters high-resolution Xevo G2-XS Q-ToF mass spectrometer coupled with an Acquity UPLC H-Class in the positive electrospray ionization mode.
3,6-Di-O-acetyl-4-O-(2,3,4,6-tetra-O-acetyl-β-D-galactopyranosyl)-1,5-anhydro-2-deoxy-D-arabino-hex-1-enitol (3). Following the procedure described by Zhao et al.16 In a round-bottomed flask, a solution of HBr (147 ml, 0.62 moles, 34% in HOAc) in dry CH2Cl2 (200 mL) was cooled to 0 °C with an ice bath. Then, a solution of lactose per-acetate (141.2 g, 0.21 moles) in dry CH2Cl2 (100 mL) was added dropwise through addition funnel. After completing the addition, the system was allowed to warm to room temperature and stirring was kept until TLC showed total consumption of the sugar (∼3 hours). The mixture was then diluted with water, transferred to a separation funnel, and extracted with CH2Cl2. The organic layer was then successively washed with H2O (3 times), and NaHCO3 (sat. 2 times). The organic phase was collected, dried over Na2SO4, filtered, and the volatiles removed in rotary evaporator. The resulting material (hepta-O-acetyl lactose bromide) was then re-dissolved in acetone (420 mL), and a saturated aqueous solution of NaH2PO4 (830 mL) was added. To the resulting biphasic system, Zn (170 g, 2.6 moles) was added portion-wise, and the suspension was maintained at room temperature under strong stirring for 12 hours. After that period, TLC showed total consumption of the starting bromide and the predominance of a slightly less polar spot. The solids were then removed by filtration through a Celite pad, and the organic filtrate was concentrated in rotary evaporator. The residue was re-dissolved in EtOAc, washed with water (2 times), dried over Na2SO4, filtered, and the volatiles removed in rotary evaporator. Flash chromatography purification of the residue afforded hexa-O-acetyl D-lactal 2 (49.4 g, 88.2 mmol, 42%) as a colourless syrup. The identity of per-acetylated lactal 3 was confirmed by comparing its 1H and 13C{1H} NMR spectra with reported data.29 Rf = 0.56 (EtOAc/n-Hex 3
:
2), 1H NMR (500 MHz, CDCl3): 6.35 (dd, J = 1.0 and 6.1 Hz, H-1), 5.34 (t, J = 4.3 Hz, H-3), 5.30 (dd, J = 1.0 and 3.3 Hz, H-4′), 5.13 (dd, J = 7.9 and 10.5 Hz, H-2′), 4.94 (dd, J = 3.4 and 10.5 Hz, H-3′), 4.77 (dd, J = 3.4 and 6.1 Hz, H-2), 4.59 (d, J = 8.0 Hz, H-1′), 4.37 (dd, J = 2.6 and 11.7 Hz, H-6), 4.13 (dd, J = 6.2 and 11.7 Hz, H-6), 4.08 (m, H-6′, H-5), 4.01 (dd, J = 7.3 and 11.2 Hz, H-6′), 3.93 (dd, J = 5.4 and 7.4 Hz, H-4), 3.84 (ddd, J = 1.0, 6.0 and 7.0 Hz, H-5′), 2.09 (s, 3H), 2.05 (s, 3H), 2.02 (s, 3H), 1.99 (s, 3H), 1.98 (s, 3H), 1.91 (s, 3H); APT 13C{1H} NMR (125 MHz, CDCl3): 170.4, 170.4, 170.2, 170.1, 169.9, 169.3, 145.4 (C-1), 101.0 (C-1′), 98.9 (C-2), 74.6 (C-4), 74.1 (C-5), 70.8 (C-3′), 70.7 (C-5′), 68.8 (C-3), 68.8 (C-4), 66.6 (C-4′), 61.8 (C-6), 60.9 (C-6′), 21.1, 20.8, 20.6, 20.6, 20.6, 20.5.
Benzyl 2,3-dideoxy-4-O-(2,3,4,6-tetra-O-acetyl-β-D-galactopyranosyl)-6-O-acetyl-α-D-erythro-hex-2-enopyranoside (4α and 4β). D-Lactal (2.0 g, 3.5 mmol) and benzylic alcohol (563 μL, 1.5 equiv.) were dissolved in dry CH2Cl2 (10.0 mL mmol−1) and cooled to 0 °C under nitrogen atmosphere. Then boron trifluoride (230 μL, 0.5 equiv.) was added dropwise, and the reaction was allowed to warm to room temperature. Reaction was stirred until TLC analysis showed total consumption of starting lactal (2 hours). Then the reaction is cooled down to 0 °C and quenched with Et3N (500 μL). Crude was diluted with CH2Cl2, transferred to a separatory funnel, and washed with water. Aqueous layer was re-extracted with CH2Cl2, and organic fractions were gathered, dried over Na2SO4, filtered, and concentrated in rotary evaporator. Silica gel flash chromatography (EtOAc/n-Hex) afforded 1.4 g (2.3 mmol, 66%) of a mixture of 4α and 4β. Physical data for major product 4a Rf = 0.5 (EtOAc/n-Hex, 1
:
3), 1H NMR (500 MHz, CDCl3): 7.35–7.26 (m, 5H), 6.11 (d, J = 10.2 Hz, H-3), 5.78 (ddd, J = 2.2, 2.2 and 10.2 Hz, H-2), 5.39 (d, J = 2.7 Hz, H-4′), 5.21 (dd, J = 8.0 and 10.4 Hz, H-2′), 5.08 (d, J = 2.2 Hz, H-1), 5.00 (dd, J = 3.4 and 10.4 Hz, H-3′), 4.79 (d, J = 11.8 Hz, OC
2Ph), 4.57 (d, J = 8.9 Hz, H-1′), 4.58 (d, J = 11.8 Hz, OC
2Ph), 4.20 (m, H-4, H-6′, H-6 and H-6), 4.11 (m, H-6′ and H-5), 3.92 (dd, J = 6.1 and 6.1 Hz, H-5′), 2.16 (s, 3H), 2.16 (s, 3H), 2.08 (s, 3H), 2.04 (s, 3H), 1.98 (s, 3H); APT 13C{1H} NMR (125 MHz, CDCl3): 170.7, 170.4, 170.3, 170.1, 169.4, 137.7, 131.6 (C-3), 128.4 (2C), 127.9 (2C), 127.8, 127.1 (C-2), 102.2 (d, C-1′), 93.6 (d, C-1), 73.4, 70.9 (C-3′), 70.8 (C-5′), 70.1 (O
H2Ph), 68.8 (C-2′), 67.6 (C-5), 66.9 (C-4′), 63.0 (C-6), 61.3 (C-6′), 20.9, 20.7, 20.7, 20.7, 20.6
Benzyl 4-O-(2,3,4,6-tetra-O-acetyl-β-D-galactopyranosyl)-6-O-acetyl-α-D-mannopyranoside (5) and Benzyl 4-O-(2,3,4,6-tetra-O-acetyl-β-D-galactopyranosyl)-6-O-acetyl-β-D-allopyranoside (6). The mixture of Ferrier adducts 4α and 4β (6.38 g, 10.5 mmol, α/β = 6
:
1) and N-methylmorpholine N-oxide (1.7 g, 12.5 mmol), were dissolved in acetone (40 mL) at room temperature. Then, OsO4 (1.3 ml, 4% sol. in water, 0.20 mmol) was added and the system was maintained for 12 hours at room temperature under vigorous stirring. After that period, TLC showed total consumption of the starting olefin and the predominance of two more polar spots. Then, the volatiles were removed in rotary evaporator and the resulting syrup was purified by normal phase flash chromatography using an isocratic EtOAc/n-Hex (7
:
3) elution system. Chromatographic separation afforded the diol 5 (3.6 g, 5.6 mmol, 53%) as a white foam, the diol 6 (758.3 mg, 1.18 mmol, 11%) as a white foam, and a mixed fraction of 5 and 6 (1.83 g, 5/6 = 12
:
1). The combined isolated yield for this reaction was 92%. Physical data for major derivative 5 (Galβ(1→4)Man): Rf = 0.57 (EtOAc), 1H NMR (500 MHz, CDCl3): 7.30–7.22 (m, 5H), 5.33 (dd, J = 0.7 and 3.4 Hz, H-4′), 5.17 (dd, J = 8.0 and 10.5 Hz, H-2′), 4.93 (dd, J = 3.4 and 10.5, H-3′), 4.88 (d, J = 1.0 Hz, H-1), 4.64 (d, J = 11.8 Hz, 1H), 4.45 (d, J = 8.0 Hz, H-1′), 4.44 (d, J = 11.8 Hz, 1H), 4.22 (dd, J = 2.0 and 11.8 Hz, H-6′), 4.11 (dd, J = 4.8 and 11.6 Hz, H-6), 4.06 (dd, J = 7.6 and 11.3 Hz, H-6), 4.01 (dd, J = 5.5 and 11.7 Hz, H-6′), 4.03 (m, H-2 and H-5′), 3.94 (dd, J = 1.0 and 8.7 Hz, H-3), 3.96 (m, 2H, H-2 and H-5′), 3.88 (dd, J = 3.5 and 8.6 Hz, H-3), 3.82 (ddd, J = 1.6, 5.4, and 9.9 Hz, H-5), 3.67 (dd, J = 8.9 and 9.6 Hz, H-4), 2.10 (s, 3H), 2.06 (s, 3H), 2.03 (s, 3H), 2.02 (s, 3H), 1.91 (s, 3H); APT 13C{1H} NMR (125 MHz, CDCl3): 170.6, 170.2, 169.9, 169.8, 169.4, 136.6, 128.4, 127.9 (x 2Cs), 127.8 (x 2Cs), 101.7 (C-1′), 97.9 (C-1), 80.4, 71.3, 70.7, 70.1, 69.4, 69.2, 68.5, 67.7, 66.7, 62.8, 61.6, 20.8, 20.5, 20.4, 20.4, 20.3; HRMS (ESI/Q-TOF) m/z: [M + Na]+ calcd for C29H38NaO16 665.2058; found 665.2137; physical data for minor derivative 6 (Galβ(1→4)All): Rf = 0.48 (EtOAc/n-Hex, 4
:
1), 1H NMR (400 MHz, CDCl3): 7.35 (m, 5H), 5.39 (d, J = 2.8 Hz, H-4′), 5.24 (dd, J = 8.2 and 10.3 Hz, H-2′), 5.01 (dd, J = 3.3 and 10.5 Hz, H-3′), 4.91 (d, J = 11.8 Hz, 1H, PhCH2O), 4.73 (d, J = 7.8 Hz, H-1), 4.64 (d, J = 11.8 Hz, 1H, PhCH2O), 4.57 (d, J = 8.0 Hz, H-1′), 4.36 (d, J = 2.1 Hz, H-3), 4.32 (dd, J = 1.6 and 12.2 Hz, H-6), 4.21 (dd, J = 7.8 and 11.2 Hz, H-6′), 4.06 (m, 2H, H-6′, H-6), 3.96 (m, 2H, H-5, H-5′), 3.64 (dd, J = 2.3 and 9.5 Hz, H-4), 3.51 (ddd, J = 3.0, 7.6, and 10.0 Hz, H-2), 2.78 (s, 1H, OH), 2.59 (d, J = 7.6 Hz, 1H, OH) 2.17 (s, 3H), 2.11 (s, 3H), 2.08 (s, 3H), 2.07 (s, 3H), 1.99 (s, 3H); APT 13C{1H} NMR (100 MHz, CDCl3): 170.7, 170.5, 170.1, 170.0, 169.5, 137.1, 128.5, 128.1, 127.9, 101.8 (C-1′), 99.5 (C-1), 78.1 (C-4), 71.4 (C-5′), 71.2 (O
H2Ph), 70.6 (C-2), 70.5 (C-3′), 69.6 (C-5), 69.5 (C-3), 68.4 (C-2′), 66.9 (C-4′), 63.2 (C-6), 61.4 (C-6′), 20.9, 20.6, 20.6, 20.5, 20.5; HRMS (ESI/Q-TOF) m/z: [M + Na]+ calcd for C29H38NaO16 665.2058; found 665.2141.
General procedure for acetylation
Diol, Ac2O (6.0 equiv.), and pyridine (3.0 equiv.) were dissolved in dry CH2Cl2 (5.0 mL mmol−1) at room temperature and stirred until TLC showed total consumption of the starting diol (∼15 min). Then, the mixture was cooled to 0 °C with an ice bath and the excess of Ac2O was quenched by addition of EtOH (30 equiv.). The volatiles were then removed in rotary evaporator and the residue was re-dissolved in EtOAc, transferred to a separation funnel, and washed with H2O followed by 1 M HCl, and NaHCO3. The organic layer was collected, dried over Na2SO4, filtered, and concentrated in rotary evaporator. The crude acetate was purified by normal phase flash chromatography using EtOAc/n-Hex (1
:
1 to 7
:
3) gradient as eluent system.
Benzyl 2,3-di-O-acetyl-4-O-(2,3,4,6-tetra-O-acetyl-β-D-galactopyranosyl)-6-O-acetyl-α-D-mannopyranoside (7). Following the general procedure for acetylation, diol 5 (2.11 g, 3.3 mmol), Ac2O (1.9 mL, 19.8 mmol), and pyridine (797 μL, 9.9 mmol), afforded the respective octaacetate 7 (2.13 g, 2.9 mmol, 88%) as a white solid. Rf = 0.44 (EtOAc/n-Hex, 3
:
2), 1H NMR (400 MHz, CDCl3): 7.31–7.22 (m, 5H), 5.32 (dd, J = 3.6 and 8.8 Hz, H-3), 5.27 (dd, J = 1.0 and 3.4 Hz, H-4′), 5.20 (dd, J = 1.9 and 3.6 Hz, H-2), 5.07 (dd, J = 7.9 and 10.5 Hz, H-2′), 4.89 (dd, J = 3.4 and 10.4 Hz, H-3′), 4.74 (d, J = 1.8 Hz, H-1), 4.62 (d, J = 11.9 Hz, 1H, OC
2Ph), 4.48 (d, J = 7.9 Hz, H-1′), 4.46 (d, J = 11.9 Hz, 1H, OC
2Ph), 4.29 (dd, J = 1.7 and 11.9 Hz, H-6a), 4.10 (m, 2H, H-6b and H-6a′), 3.96 (dd, J = 7.7 and 11.9 Hz, H-6b′), 3.86 (m, 2H, H-5 and H-4), 3.80 (dd, J = 1.0 and 7.7 Hz, H-5′), 2.08 (s, 3H), 2.07 (s, 3H), 2.04 (s, 3H), 1.99 (s, 3H), 1.98 (s, 3H), 1.97 (s, 3H), 1.90 (s, 3H); 13C{1H} NMR (100 MHz, CDCl3): 170.5, 170.4, 170.2, 170.1, 169.8, 169.3, 169.2, 136.2, 128.6 (×2C), 128.2 (×2C), 128.2, 101.1 (C-1), 96.4 (C-1), 74.0, 71.0, 70.4, 69.7, 69.6, 69.6, 69.2, 69.1, 66.6, 62.6, 60.8, 20.9, 20.8, 20.8, 20.6, 20.6, 20.6, 20.5; HRMS (ESI/Q-TOF) m/z: [M + Na]+ calcd for C33H42NaO18 749.2269; found 749.2399.
Benzyl 2,3-di-O-acetyl-4-O-(2,3,4,6-tetra-O-acetyl-β-D-galactopyranosyl)-6-O-acetyl-β-D-allopyranoside (8). Following the general procedure for acetylation, diol 6 (124.2 mg, 0.19 mmol), Ac2O (107 μL, 1.14 mmol), and pyridine (45 μL, 0.57 mmol) afforded the respective octaacetate 8 (109.0 mg, 0.15 mmol, 81%) as a white solid. Rf = 0.45 (EtOAc/n-Hex, 3
:
2), 1H NMR (400 MHz, CDCl3): 7.33 (m, 5H), 5.76 (t, J = 2.5 Hz, H-3), 5.33 (d, J = 2.6 Hz, H-4′), 5.15 (dd, J = 7.9 and 10.3 Hz, H-2′), 4.98 (dd, J = 3.2 and 10.4 Hz, H-3′), 4.89 (d, J = 12.1 Hz, 1H, OC
2Ph), 4.83 (d, J = 8.3 Hz, H-1), 4.77 (dd, J = 2.5 and 8.2 Hz, H-2), 4.63 (d, J = 12.1 Hz, 1H, OC
2Ph), 4.56 (d, J = 7.8 Hz, H-1′), 4.38 (d, J = 10.1 Hz, H-6), 4.03 (m, 4H, H-5, H-6′, H-6Gal, H-6′Gal), 3.91 (d, J = 6.4 and 6.4 Hz, H-5′), 3.80 (d, J = 2.5 and 9.3 Hz, H-4), 2.12 (s, 3H), 2.11 (s, 3H), 2.08 (s, 3H), 2.07 (s, 3H), 2.06 (s, 3H), 1.98 (s, 3H), 1.97 (s, 3H); 13C{1H} NMR (100 MHz, CDCl3): 170.6, 170.6, 170.1, 169.9, 169.6, 169.6, 169.5, 137.1, 128.3 (×2C), 127.8, 127.6 (×2C), 100.7 (C-1′), 97.4 (C-1), 74.9 (C-4), 70.9 (O
H2Ph), 70.8 (C-5′), 70.7 (C-3′), 70.6 (C-5), 70.1 (C-2), 68.9 (C-3), 68.7 (C-2′), 66.8 (C-4′), 62.9 (C-6), 61.3 (C-6′), 20.8, 20.8, 20.6, 20.6, 20.6, 20.5, 20.5; HRMS (ESI/Q-TOF) m/z: [M + Na]+ calcd for C33H42NaO18 749.2269; found 749.2330.
2,3-Di-O-acetyl-4-O-(2,3,4,6-tetra-O-acetyl β-D-galactopyranosyl)-6-O-acetyl-α-D-mannopyranose (9). In a round-bottomed flask, benzyl glycoside 7 (1.92 g, 2.6 mmol) was dissolved in EtOH (300 mL) and Pd/C (260 mg) was added under inert atmosphere (N2). The system was closed with a septum and the N2 replaced by a reducing H2 atmosphere via four vacuum/H2 cycles. The reaction was vigorously stirred at room temperature until TLC showed complete consumption of the starting benzyl glycoside (∼3 hours). The catalyst was removed by passing the suspension through a Celite® pad and the volatiles were removed by rotary evaporation. The resulting crude product was used in the next step without further purification. This procedure afforded the alcohol 9 (1.59 g, 2.5 mmol, 96%, α
:
β = 8
:
1) as a colorless syrup. Physical data for major anomer (α): Rf = 0.62 (EtOAc/n-Hex, 19
:
1), 1H NMR (400 MHz, CDCl3): 5.34 (dd, J = 3.5 and 9.5 Hz, H-3), 5.28 (d, J = 2.5 Hz, H-4′), 5.15 (dd, J = 1.5 and 3.3 Hz, H-2), 5.08 (d, J = 1.5 Hz, H-1), 5.05 (dd, J = 7.8 and 10.4 Hz, H-2′), 4.90 (dd, J = 3.3 and 10.5 Hz, H-3′), 4.49 (d, J = 7.9 Hz, H-1′), 4.38 (dd, J = 3.5 and 13.2 Hz, H-6Man), 4.10 (m, 3H, H-5, H-6Gal, and H-6′Man), 3.96 (dd, J = 7.6 and 11.0 Hz, H-6′Gal), 3.84 (m, 2H, H5′ and H4), 2.09 (s, 3H), 2.07 (s, 3H), 2.06 (s, 3H), 1.99 (s, 3H), 1.99 (s, 3H), 1.97 (s, 3H), 1.90 (s, 3H); 13C{1H} NMR (100 MHz, CDCl3): 170.8, 170.5, 170.3, 170.2, 170.1, 169.6, 169.3, 100.9 (C-1′), 91.8 (C-1), 74.2 (C-4), 70.9 (C-3′), 70.4 (C-5′), 70.3 (C-2), 69.2 (C-2′), 69.1 (C-3), 68.9 (C-5), 66.7 (C-4′), 62.6 (C-6), 60.9 (C-6′), 20.9, 20.9, 20.8, 20.6, 20.6, 20.6, 20.5; HRMS (ESI/Q-TOF) m/z: [M + Na]+ calcd for C26H36NaO18 659.1799; found 659.1939.
4-O-(2,3,4,6-tetra-O-acetyl-β-D-galactopyranosyl)-1-(2,2,2-trichloroethanimidate)-α-D-mannopyranose 2,3,6-triacetate (10). To a solution of alcohol 9 (535.2 mg, 0.84 mmol), and Cl3CCN (400 mL, 5.04 mmol) in dry CH2Cl2 (4.0 mL), 1,8-diazabicyclo[5.4.0]undec-7-ene (DBU, 37 mL, 0.25 mmol) was added and the system was stirred at room temperature for 18 hours. After that period, TLC showed total consumption of the starting material. The volatiles were then removed in rotary evaporator and the residue was directly purified by normal phase flash chromatography. The imidate 10 (446.1 mg, 0.57 mmol, 68%) was obtained as a yellowish foam. Rf = 0.47 (EtOAc/n-Hex/Et3N, 60
:
40
:
1), 1H NMR (400 MHz, CDCl3): 8.68 (s, 1H), 6.15 (d, J = 2.0 Hz, H-1), 5.37 (m, 2H, H-2, H-3), 5.29 (dd, J = 0.8 and 3.3 Hz, H-4′), 5.09 (dd, J = 8.0 and 10.5 Hz, H-2′), 4.91 (dd, J = 3.4 and 10.5 Hz, H-3′), 4.52 (d, J = 7.8 Hz, H-1′), 4.37 (dd, J = 1.9 and 11.9 Hz, H-6), 4.12 (dd, J = 6.1 and 11.1 Hz, H-6′), 4.10 (dd, J = 5.2 and 11.1 Hz, H-6), 4.05 (m, H-5), 3.97 (dd, J = 7.7 and 11.2 Hz, H-6′), 3.93 (dd, J = 9.6 and 9.6 Hz, H-4), 3.83 (dd, J = 7.4 and 7.4 Hz, H-5′), 2.11 (s, 3H), 2.09 (s, 3H), 2.04 (s, 3H), 1.99 (s, 3H), 1.98 (s, 3H), 1.98 (s, 3H), 1.91 (s, 3H). APT 13C{1H} NMR (100 MHz, CDCl3): 170.4, 170.4, 170.2, 170.1, 169.5, 169.3, 169.2, 160.0 (![[C with combining low line]](https://www.rsc.org/images/entities/i_char_0043_0332.gif)
NH), 101.4 (C-1′), 94.5 (C-1), 90.5 (
Cl3), 74.2 (C-4), 71.5 (C-5), 71.0 (C-3′), 70.5 (C-5′), 69.2 (C-3), 69.2 (C-2′), 67.9 (C-2), 66.5 (C-4′), 62.2 (C-6), 60.8 (C-6′), 20.8, 20.8, 20.8, 20.7, 20.7, 20.6, 20.6; HRMS (ESI/Q-TOF) m/z: [M + Na]+ calcd for C28H36Cl3NNaO18 802.0896; found 802.0851.
3-Azidopropyl 2,3-di-O-acetyl-4-O-(2,3,4,6-tetra-O-acetyl-β-D-galactopyranosyl)-6-O-acetyl-α-D-mannopyranoside (11). Imidate 10 (367.0 mg, 0.47 mmol) and 3-azidopropanol (142.5 mg, 1.41 mmol) were placed in a round-bottomed flask. Prior to glycosyl donor activation, the system was dried via azeotropic removal of H2O traces with toluene (3 cycles of ∼2.0 mL) by rotary evaporation. The reactants were then dissolved in dry CH2Cl2 (3.0 mL) and cooled to 0 °C with an ice bath. Imidate was activated by addition of BF3·Et2O (30 mL, 0.24 mmol) and the reaction was allowed to warm at room temperature under stirring. After 3 hours, TLC showed a significant amount of remaining imidate and more BF3Et2O (50 mL, 0.4 mmol) was added. After 1 hour, TLC showed reaction completion and the excess of Lewis acid was quenched with Et3N (1.0 mL). Then, the volatiles were removed by rotary evaporation and the residue directly purified by normal phase flash chromatography using EtOAc/n-Hex (3
:
7 to 3
:
2) gradient as eluent system. Glycoside 11 (240.0 mg, 0.33 mmol, 70%) was obtained as a colorless oil. Rf = 0.43 (EtOAc/n-Hex, 7
:
3), 1H NMR (500 MHz, CDCl3): 5.28 (dd, J = 1.0 and 3.4 Hz, H-4′), 5.25 (dd, J = 3.6 and 9.1 Hz, H-3), 5.15 (dd, J = 1.9 and 3.6 Hz, H-2), 5.07 (dd, J = 7.9 and 10.5 Hz, H-2′), 4.90 (dd, J = 3.4 and 10.4 Hz, H-3′), 4.68 (d, J = 1.9 Hz, H-1), 4.48 (dd, J = 8.0 Hz, H-1′), 4.35 (dd, J = 1.2 and 11.9 Hz, H-6Man), 4.12 (dd, J = 6.0 and 11.1 Hz, H-6′Man), 4.11 (dd, J = 1.3 and 11.6 Hz, H-6Gal), 3.96 (dd, J = 7.8 and 11.1 Hz, H-6′Gal), 3.82 (m, 3H, H-4, H-5, and H-5′), 3.71 (m, 1H), 3.43 (m, 1H), 3.35 (t, J = 6.5 Hz, 2H), 2.09 (s, 3H), 2.07 (s, 3H), 2.06 (s, 3H), 2.00 (s, 3H), 1.99 (s, 3H), 1.97 (s, 3H), 1.91 (s, 3H), 1.79 (m, 2H); APT 13C{1H} NMR (125 MHz, CDCl3): 170.5, 170.4, 170.2, 170.1, 169.8, 169.3, 169.2, 101.2 (C-1′), 97.4 (C-1), 74.4, 70.9 (C-3′), 70.4, 69.6 (C-2), 69.4 (C-3), 69.2 (C-2′), 69.1, 66.6 (C-4′), 64.8 (O
H2CH2CH2N3), 62.5 (C-6), 60.8 (C-6′), 48.1 (OCH2CH2
H2N3), 28.6 (OCH2
H2CH2N3), 20.9, 20.8, 20.8, 20.7, 20.6, 20.6, 20.5; HRMS (ESI/Q-TOF) m/z: [M + Na]+ calcd for C29H41N3NaO18 742.2283; found 742.2336.
3-Azidopropyl 4-O-β-D-galactopyranosyl-α-D-mannopyranoside (1). In a round-bottomed flask provided with a magnetic bar, per-acetylated disaccharide 11 (221.1 mg, 0.31 mmol) and NaOMe (∼10 mg) were dissolved in CH3OH (3.0 mL) at room temperature. The solution was stirred until TLC showed total consumption of the starting disaccharide (∼12 h). The reaction was then diluted with CH3OH (3.0 mL) and neutralized with Amberlite IR-120 (H form). The mixture was filtered, and the volatiles removed by rotary evaporation. This procedure afforded the target azide-derived Galβ(1→4)αMan disaccharide 1 as a white foam (127.6 mg, 0.30 mmol, 97%). Rf = 0.50 (MeOH/CH2Cl2, 1
:
4), 1H NMR (400 MHz, CD3OD): 4.78 (s, H-1), 4.36 (d, J = 7.4 Hz, H-1′), 3.90 (m, 4H), 3.81 (m, 4H), 3.73 (dd, J = 4.3 and 11.5 Hz, H-6), 3.63 (m, 2H), 3.53 (m, 3H), 3.41 (m, 2H), 1.88 (m, 2H); 13C{1H} NMR (100 MHz, CD3OD): 103.8 (C-1′), 100.0 (C-1), 76.9, 75.8, 73.4, 71.7, 71.2, 70.1, 69.8, 69.0, 64.1, 61.2, 60.7, 48.1, 28.5; HRMS (ESI/Q-TOF) m/z: [M + Na]+ calcd for C15H27N3NaO11 448.1543; found 448.1654.
2,3-Di-O-acetyl-4-O-(2,3,4,6-tetra-O-acetyl-β-D-galactopyranosyl)-6-O-acetyl-α-D-mannopyranosyl H-phosphonate (12). In a round-bottomed flask provided with a magnetic bar, imidazole (2.6 g, 38.2 mmol) and Et3N (5.4 mL, 38.7 mmol) were suspended in MeCN (30.0 mL) and cooled to 0 °C. PCl3 (1.1 mL, 12.6 mmol) was then added, and the resulting suspension was vigorously stirred at 0 °C for 20 minutes. Then, a solution of alcohol 9 (1.0 g, 1.6 mmol) in MeCN (5.0 mL) was added dropwise over 30 minutes under vigorous stirring. The reaction was allowed to warm to room temperature. After 4 h, TLC showed complete consumption of the starting disaccharide, and the system was quenched by addition of 1 M TEAB buffer (27 mL, pH 7.2) and left stirring for 20 minutes. The volatiles were then removed by rotary evaporation and the residue was re-dissolved in CHCl3, transferred to a separation funnel, and washed with 1 M TEAB buffer. The organic layer was collected, dried over Na2SO4, filtered, concentrated in rotary evaporator, and the resulting crude was purified by normal phase flash chromatography using a MeOH/CH2Cl2 gradient (1
:
24 to 1
:
9) containing 1% Et3N as mobile phase. Purification afforded H-phosphonate 12 (409.1 mg, 0.50 mmol, 42%) as a white solid. Rf = 0.50 (MeOH/CH2Cl2, 1
:
4), 1H NMR (400 MHz, CDCl3): 6.92 (d, J = 638 Hz, P–
), 5.47 (dd, 3JH1–H2 = 2.3 and 3JH–P = 10.5 Hz, H-1), 5.32 (dd, J = 3.4 and 9.5 Hz, H-3), 5.29 (d, J = 3.0 Hz, H-4′), 5.21 (m, H-2), 5.07 (dd, J = 7.4 and 10.0 Hz, H-2′), 4.89 (dd, J = 3.5 and 10.0 Hz, H-3′), 4.47 (d, J = 10.5 Hz, H-1′), 4.35 (dd, J = 3.2 and 12.0 Hz, H-6Man), 4.12 (m, 3H, H-5, H-6Gal, and H-6′Man), 3.96 (dd, J = 7.7 and 11.0 Hz, H-6′Gal), 3.84 (t, J = 9.3 Hz, H-5′), 3.82 (dd, J = 7.2 and 7.2 Hz, H-4), 3.02 (q, J = 7.3 Hz, 6H, N(C
2CH3)3), 2.09 (s, 3H), 2.06 (s, 3H), 2.05 (s, 3H), 1.99 (s, 3H), 1.98 (s, 3H), 1.94 (s, 3H), 1.91 (s, 3H), 1.30 (t, J = 7.3 Hz, 9H, N(CH2C
3)3); 13C{1H} NMR (100 MHz, CDCl3): 170.6, 170.4, 170.2, 170.1, 169.8, 169.6, 169.1, 101.1 (C-1′), 92.6 (d, 2JC–P = 3.7 Hz, C-1), 74.0 (C-5′), 70.9 (C-3′), 70.4 (C-4), 70.1 (d, 3JC–P = 7.2 Hz, C-2), 69.9 (C-5), 69.4 (C-3), 69.1 (C-2′), 66.7 (C-4′), 62.4 (C-6), 60.8 (C-6′), 45.7 (N(
H2CH3)3), 20.8, 20.8, 20.8, 20.6, 20.6, 20.6, 20.5, 8.6 (N(CH2
H3)3); 31P NMR (162 MHz, CDCl3): −0.10 (dd, 1JP–H = 638 Hz, 3JP–H1 = 9.8 Hz); HRMS (ESI/Q-TOF) m/z: [M − H]− calcd for C26H36O20P 699.1543; found 699.1545.
Triethylammonium 3-azidopropyl 2,3-di-O-acetyl-4-O-(2,3,4,6-tetra-O-acetyl-β-D-galactopyranosyl)-6-O-acetyl-α-D-mannopyranosyl phosphate (13). In a round-bottomed flask provided with a magnetic bar, H-phosphonate 12 (100.1 mg, 0.12 mmol) and 3-azidopropanol (14 μL, 0.15 mmol) were dissolved in dry THF (2.8 mL) and cooled to 0 °C with an ice bath. To the resulting solution, PivCl (37 μL, 0.3 mmol) was added and the system was stirred allowing it to warm to room temperature. After 3 hours, TLC showed complete consumption of the starting H-phosphonate and a solution of I2 (121.8 mg, 0.48 mmol) in pyridine/H2O (19
:
1, 1.0 mL) was added. TLC showed the oxidation of H-phosphonate to phosphate completes in less than 30 minutes. The reaction was then quenched by addition of 10% Na2S2O3 (6.0 mL), transferred to a separation funnel, and extracted with CHCl3. The organic layer was then successively washed with brine, and 1 M TEAB buffer. The collected organic fraction was then dried over Na2SO4, filtered, and concentrated in rotary evaporator. The resulting crude was purified by normal phase flash chromatography using a MeOH/CH2Cl2 gradient (1
:
99 to 1
:
9) containing 1% of Et3N. This procedure afforded the phosphodiester 13 (30.2 mg, 0.03 mmol, 30%) as a white solid. Rf = 0.5 (MeOH/CH2Cl2, 15
:
85), 1H NMR (400 MHz, CDCl3): 11.9 (br s, 1H, HN+(CH2CH3)3), 5.41 (dd, 3JH1–H2 = <1.0 Hz and 3JH1–P = 7.9 Hz, H-1), 5.31 (dd, J = 3.5 and 9.4 Hz, H-3), 5.28 (d, J = 3.3 Hz, H-4′), 5.24 (s, H-2), 5.07 (dd, J = 8.2 and 10.4 Hz, H-2′), 4.89 (dd, J = 3.2 and 10.4 Hz, H-3′), 4.47 (d, J = 8.0 Hz, H-1′), 4.36 (d, J = 10.9 Hz, H-6Man), 4.12 (m, 3H, H-5, H-6′Man, H-6Gal), 3.95 (dd, J = 7.9 and 11.0 Hz, H-6′Gal), 3.92 (m, 2H, OC
2CH2CH2N3), 3.87 (t, J = 9.7 Hz, H-4), 3.81 (dd, J = 6.8 and 8.0 Hz, H-5′), 3.37 (t, J = 6.9 Hz, OCH2CH2C
2N3), 3.04 (m, 6H, HN+(CH2CH3)3), 2.09 (s, 3H), 2.06 (s, 3H), 2.06 (s, 3H), 1.99 (s, 3H), 1.98 (s, 3H), 1.94 (s, 3H), 1.91 (s, 3H), 1.83 (m, 2H, OCH2C
2CH2N3), 1.33 (t, J = 7.3 Hz, 9H, HN+(CH2CH3)3). APT 13C{1H} (100 MHz, CDCl3): 170.5, 170.4, 170.2, 170.1, 169.8, 169.6, 169.1, 101.1 (C-1′), 93.4 (d, 2JC1–P = 6.0 Hz, C-1), 73.8 (C-5′), 70.9 (C-3′), 70.4 (C-4), 69.8 (d, 2JC2–P = 6.8 Hz, C-2), 69.8 (C-5), 69.5 (C-3), 69.1 (C-2), 66.6 (C-4′), 62.7 (d, 2JC1–P = 5.3 Hz, OCH2CH2CH2N3), 62.5 (C-6), 60.8 (C-6′), 48.2 (OCH2CH2CH2N3), 45.8 (HN+(CH2CH3)3), 30.1 (d, 2JC–P = 7.4 Hz, OCH2CH2CH2N3), 20.9, 20.9, 20.8, 20.6, 20.6, 20.6, 20.5, 8.6 (HN+(CH2CH3)3); 31P NMR (162 MHz, CDCl3): −3.09 (d, 3JP–H1 = 6.9 Hz); HRMS (ESI/Q-TOF) m/z: [M − H]− calcd for C29H41N3O21P 798.1976, found 798.1972.
3-Azidopropyl 4-O-β-D-galactopyranosyl-α-D-mannopyranosyl phosphate (2). In a round-bottomed flask provided with a magnetic bar, phosphodiester 13 (83.0 mg, 0.09 mmol) and NaOMe (14.6 mg, 0.27 mmol) were suspended in CH3OH (5.0 mL) and stirred at room temperature for 48 hours. Then, the reaction was diluted with CH3OH (5.0 mL) and NH4Cl was added dropwise until reaching pH ∼7. The volatiles were then removed in rotary evaporator and the crude was subjected to purification by flash reverse phase chromatography using C-18 as stationary phase and MeCN/H2O gradient (1
:
99 to 1
:
1) as eluent system. This procedure afforded the deprotected azido-derived Galβ(1→4)αMan phospho-glycoside 2 (33.7 mg, 0.06 mmol, 67%) as a white solid. 1H NMR (400 MHz, CD3OD): 5.34 (dd, 3JH1–H2 = 1.5 Hz, 3JH1–P = 7.9 Hz, H-1), 4.25 (d, J = 7.5 Hz, H-1′), 3.85 (m, 2H), 3.83 (m, 2H), 3.79 (m, 5H), 3.70 (m, 3H), 3.61 (dd, J = 4.1 and 11.5 Hz, H-6), 3.50 (dd, J = 4.1 and 7.3 Hz, 1H), 3.45 (dd, J = 7.6 and 9.7 Hz, 1H), 3.40 (d, J = 2.6 Hz, H-4′), 3.36 (m, 4H), 1.79 (m, 2H), 1.26 (t, J = 7.2 Hz, 3H); APT 13C{1H} (100 MHz, CD3OD): 103.9 (C-1′), 96.1 (d, 2JC1–P = 5.5 Hz, C-1), 76.6, 75.7, 73.3, 72.6, 71.1, 70.5 (d, JC–P = 8.6 Hz), 69.1, 69.0, 62.3 (d, JC–P = 5.8 Hz, OCH2CH2CH2N3), 61.3, 60.4, 52.2, 29.7 (d, JC–P = 7.6 Hz, OCH2CH2CH2N3); 31P NMR (162 MHz, CD3OD): −1.82 (q, J = 7.1 Hz); HRMS (ESI/Q-TOF) m/z: [M − H]− calcd for C15H27N3O14P 504.1236; found 504.1239.
Conflicts of interest
The authors declare no competing financial interests or personal relationships that could have influenced the performance of this work.
Acknowledgements
C. A. S. thanks the SJU's Office of Grants and Sponsored Research for Seed Grant FY23 and the Department of Pharmaceutical Sciences for generous starting funds. D. B. and B. D. thanks the Department of Pharmaceutical Sciences for graduate assistantship. We thank Dr Fereshteh Zandkarimi, director of the Mass Spectrometry Core Facilities at Columbia University, for facilitating access to HRMS.
References
- CDC, Epidemiology and Risk factors, https://www.cdc.gov/parasites/leishmaniasis/epi.html, accessed on May 8th, 2022 Search PubMed.
- M. Boelaert and S. Sundar, 47 – Leishmaniasis, in Manson's Tropical Infectious Diseases (Twenty-third Edition), ed. J. Farrar, P. J. Hotez, T. Junghanss, G. Kang, D. Lalloo and N. J. White, W.B. Saunders, London, 2014, pp 631–651 Search PubMed.
- S. Mann, K. Frasca, S. Scherrer, A. F. Henao-Martínez, S. Newman, P. Ramanan and J. A. Suarez, A Review of Leishmaniasis: Current Knowledge and Future Directions, Current Tropical Medicine Reports, 2021, 8(2), 121–132 CrossRef.
-
(a) J. B., B. M. and K. Chanda, An Overview on the Therapeutics of Neglected Infectious Diseases—Leishmaniasis and Chagas Diseases, Front. Chem., 2021, 9(37), 622286 Search PubMed;
(b) N. K. Copeland and N. E. Aronson, Leishmaniasis: treatment updates and clinical practice guidelines review, Curr. Opin. Infect. Dis., 2015, 28(5), 426–437 CrossRef CAS;
(c) B. Monge-Maillo and R. López-Vélez, Therapeutic Options for Visceral Leishmaniasis, Drugs, 2013, 73(17), 1863–1888 CrossRef CAS PubMed;
(d) N. Singh, M. Kumar and R. K. Singh, Leishmaniasis: current status of available drugs and new potential drug targets, Asian Pac. J. Trop. Med., 2012, 5(6), 485–497 CrossRef CAS.
-
(a) M. J. McConville and M. A. J. Ferguson, The structure, biosynthesis and function of glycosylated phosphatidylinositols in the parasitic protozoa and higher eukaryotes, Biochem. J., 1993, 294(2), 305–324 CrossRef CAS PubMed;
(b) R. R. de Assis, I. C. Ibraim, P. M. Nogueira, R. P. Soares and S. J. Turco, Glycoconjugates in New World species of Leishmania: polymorphisms in lipophosphoglycan and glycoinositolphospholipids and interaction with hosts, Biochim. Biophys. Acta Gen. Subj., 2012, 1820(9), 1354–1365 CrossRef PubMed;
(c) J. M. Coelho-Finamore, V. C. Freitas, R. R. Assis, M. N. Melo, N. Novozhilova, N. F. Secundino, P. F. Pimenta, S. J. Turco and R. P. Soares, Leishmania infantum: Lipophosphoglycan intraspecific variation and interaction with vertebrate and invertebrate hosts, Int. J. Parasitol., 2011, 41(3), 333–342 CrossRef CAS PubMed;
(d) M. J. McConville, L. F. Schnur, C. Jaffe and P. Schneider, Structure of Leishmania lipophosphoglycan: inter- and intra-specific polymorphism in Old World species, Biochem. J., 1995, 310(3), 807–818 CrossRef CAS PubMed.
-
(a) M. J. McConville, S. J. Turco, M. A. Ferguson and D. L. Sacks, Developmental modification of lipophosphoglycan during the differentiation of Leishmania major promastigotes to an infectious stage, EMBO J., 1992, 11(10), 3593–3600 CrossRef CAS PubMed;
(b) S. J. Turco and A. Descoteaux, The Lipophosphoglycan of Leishmania Parasites, Annu. Rev. Microbiol., 1992, 46(1), 65–92 CrossRef CAS PubMed.
- D. L. Sacks, P. F. Pimenta, M. J. McConville, P. Schneider and S. J. Turco, Stage-specific binding of Leishmania donovani to the sand fly vector midgut is regulated by conformational changes in the abundant surface lipophosphoglycan, J. Exp. Med., 1995, 181(2), 685–697 CrossRef CAS.
- R. P. P. Soares, M. E. Macedo, C. Ropert, N. F. Gontijo, I. C. Almeida, R. T. Gazzinelli, P. F. P. Pimenta and S. J. Turco, Leishmania chagasi: lipophosphoglycan characterization and binding to the midgut of the sand fly vector Lutzomyia longipalpis, Mol. Biochem. Parasitol., 2002, 121(2), 213–224 CrossRef CAS.
-
(a) G. F. Späth, L. Epstein, B. Leader, S. M. Singer, H. A. Avila, S. J. Turco and S. M. Beverley, Lipophosphoglycan is a virulence factor distinct from related glycoconjugates in the protozoan parasite Leishmania major, Proc. Natl. Acad. Sci. U.S.A., 2000, 97(16), 9258–9263 CrossRef PubMed;
(b) F. H. Routier, A. V. Nikolaev and M. A. J. Ferguson, The preparation of neoglycoconjugates containing inter-saccharide
phosphodiester linkages as potential anti-Leishmania vaccines, Glycoconjugate J., 1999, 16(12), 773–780 CrossRef CAS PubMed;
(c) H. Moll, G. F. Mitchell, M. J. McConville and E. Handman, Evidence of T-cell recognition in mice of a purified lipophosphoglycan from Leishmania major, Infect. Immun., 1989, 57(11), 3349–3356 CrossRef CAS;
(d) D. G. Russell and J. Alexander, Effective immunization against cutaneous leishmaniasis with defined membrane antigens reconstituted into liposomes, J. Immunol., 1988, 140(4), 1274 CAS;
(e) M. J. McConville, A. Bacic, G. F. Mitchell and E. Handman, Lipophosphoglycan of Leishmania major that vaccinates against cutaneous leishmaniasis contains an alkylglycerophosphoinositol lipid anchor, Proc. Natl. Acad. Sci. U.S.A., 1987, 84(24), 8941–8945 CrossRef CAS PubMed.
-
(a) G. Sureshkumar and S. Hotha, AuBr3 mediated glycosidations: synthesis of tetrasaccharide motif of the Leishmania donovani lipophosphoglycan, Glycoconjugate J., 2012, 29(4), 221–230 CrossRef CAS PubMed;
(b) A. J. Ross, O. V. Sizova and A. V. Nikolaev, Parasite glycoconjugates. Part 16: synthesis of a disaccharide and phosphorylated di- and tri-saccharides from Leishmania lipophosphoglycan, Carbohydr. Res., 2006, 341(11), 1954–1964 CrossRef CAS PubMed;
(c) D. Ruhela and R. A. Vishwakarma, A facile and novel route to the antigenic branched phosphoglycan of the protozoan Leishmania major parasite, Tetrahedron Lett., 2004, 45(12), 2589–2592 CrossRef CAS;
(d) D. Ruhela and R. A. Vishwakarma, Efficient synthesis of the antigenic phosphoglycans of the parasite, Chem. Commun., 2001,(19), 2024–2025 RSC;
(e) M. C. Hewitt and P. H. Seeberger, Solution and Solid-Support Synthesis of a Potential Leishmaniasis Carbohydrate Vaccine, J. Org. Chem., 2001, 66(12), 4233–4243 CrossRef CAS;
(f) M. Upreti and R. A. Vishwakarma, Synthesis of the phosphodisaccharide repeat of antigenic lipophosphoglycan of Leishmania donovani parasite, Tetrahedron Lett., 1999, 40(13), 2619–2622 CrossRef CAS;
(g) A. P. Higson, Y. E. Tsvetkov, M. A. J. Ferguson and A. V. Nikolaev, The synthesis of Leishmania major phosphoglycan fragments, Tetrahedron Lett., 1999, 40(52), 9281–9284 CrossRef CAS;
(h) P. Higson, A. E. Tsvetkov, Y. A. J. Ferguson and M. V. Nikolaev, Parasite glycoconjugates. Part 8.1 chemical synthesis of a heptaglycosyl triphosphate fragment of Leishmania mexicana lipo- and proteo-phosphoglycan and of a phosphorylated trisaccharide fragment of Leishmania donovani surface lipophosphoglycan, J. Chem. Soc., Perkin Trans. 1, 1998, 16, 2587–2596 RSC;
(i) V. Nikolaev, A. M. Watt, G. A. J. Ferguson and M. S. Brimacombe, Parasite glycoconjugates. Part 6.1 chemical synthesis of phosphorylated penta- and hepta-saccharide fragments of Leishmania major antigenic lipophosphoglycan, J. Chem. Soc., Perkin Trans. 1, 1997,(6), 969–980 RSC;
(j) A. V. Nikolaev, T. J. Rutherford, M. A. J. Ferguson and J. S. Brimacombe, Parasite glycoconjugates. Part 4. Chemical synthesis of disaccharide and phosphorylated oligosaccharide fragments of Leishmania donovani antigenic lipophosphoglycan, J. Chem. Soc., Perkin Trans. 1, 1995, 16, 1977–1987 RSC.
-
(a) C. Anish, C. E. Martin, A. Wahlbrink, C. Bogdan, P. Ntais, M. Antoniou and P. H. Seeberger, Immunogenicity and Diagnostic Potential of Synthetic Antigenic Cell Surface Glycans of Leishmania, ACS Chem. Biol., 2013, 8(11), 2412–2422 CrossRef CAS PubMed;
(b) M. E. Rogers, O. V. Sizova, M. A. J. Ferguson, A. V. Nikolaev and P. A. Bates, Synthetic Glycovaccine Protects against the Bite of Leishmania-Infected Sand Flies, J. Infect. Dis., 2006, 194(4), 512–518 CrossRef;
(c) X. Liu, S. Siegrist, M. Amacker, R. Zurbriggen, G. Pluschke and P. H. Seeberger, Enhancement of the Immunogenicity of Synthetic Carbohydrates by Conjugation to Virosomes: A Leishmaniasis Vaccine Candidate, ACS Chem. Biol., 2006, 1(3), 161–164 CrossRef CAS.
-
(a) G. M. Rodrigues da Cunha, M. A. Azevedo, D. S. Nogueira, M. d. C. Clímaco, E. Valencia Ayala, J. A. Jimenez Chunga, R. J. Y. La Valle, L. M. da Cunha Galvão, E. Chiari, C. R. N. Brito, R. P. Soares, P. M. Nogueira, R. T. Fujiwara, R. Gazzinelli, R. Hincapie, C.-S. Chaves, F. M. S. Oliveira, M. G. Finn and A. F. Marques, α-Gal immunization positively impacts Trypanosoma cruzi colonization of heart tissue in a mouse model, PLoS Neglected Trop. Dis., 2021, 15(7), e0009613 CrossRef CAS PubMed;
(b) A. P. V. Moura, L. C. B. Santos, C. R. N. Brito, E. Valencia, C. Junqueira, A. A. P. Filho, M. R. V. Sant'Anna, N. F. Gontijo, D. C. Bartholomeu, R. T. Fujiwara, R. T. Gazzinelli, C. S. McKay, C. A. Sanhueza, M. G. Finn and A. F. Marques, Virus-like Particle Display of the α-Gal Carbohydrate for Vaccination against Leishmania Infection, ACS Cent. Sci., 2017, 3(9), 1026–1031 CrossRef CAS.
-
(a) M. M. Alam, C. M. Jarvis, R. Hincapie, C. S. McKay, J. Schimer, C. A. Sanhueza, K. Xu, R. C. Diehl, M. G. Finn and L. L. Kiessling, Glycan-Modified Virus-like Particles Evoke T Helper Type 1-like Immune Responses, ACS Nano, 2021, 15(1), 309–321 CrossRef CAS PubMed;
(b) Z. Yin, M. Comellas-Aragones, S. Chowdhury, P. Bentley, K. Kaczanowska, L. BenMohamed, J. C. Gildersleeve, M. G. Finn and X. Huang, Boosting Immunity to Small Tumor-Associated Carbohydrates with Bacteriophage Qβ Capsids, ACS Chem. Biol., 2013, 8(6), 1253–1262 CrossRef CAS PubMed.
- R. J. Ferrier, W. G. Overend and A. E. Ryan, The reaction between 3,4,6-tri-O-acetyl-D-glucal and p-nitrophenol, J. Chem. Soc., 1962, 3667–3670 RSC.
-
(a) H. C. Kolb, M. S. VanNieuwenhze and K. B. Sharpless, Catalytic Asymmetric Dihydroxylation, Chem. Rev., 1994, 94(8), 2483–2547 CrossRef CAS;
(b) K. B. Sharpless, W. Amberg, Y. L. Bennani, G. A. Crispino, J. Hartung, K. S. Jeong, H. L. Kwong, K. Morikawa and Z. M. Wang, The osmium-catalyzed asymmetric dihydroxylation: a new ligand class and a process improvement, J. Org. Chem., 1992, 57(10), 2768–2771 CrossRef CAS.
- J. Zhao, S. Wei, X. Ma and H. Shao, A simple and convenient method for the synthesis of pyranoid glycals, Carbohydr. Res., 2010, 345(1), 168–171 CrossRef CAS.
-
(a) R. J. Ferrier and N. Prasad, Unsaturated carbohydrates. Part IX. Synthesis of 2,3-dideoxy-α-D-erythro-hex-2-enopyranosides from tri-O-acetyl-D-glucal, J. Chem. Soc. C, 1969, 4, 570–575 RSC;
(b) A. M. Gómez, F. Lobo, C. Uriel and J. C. López, Recent Developments in the Ferrier Rearrangement, Eur. J. Org. Chem., 2013, 32, 7221–7262 CrossRef;
(c) G. Descotes and J.-C. Martin, Sur l'isomérisation du 1,5-anhydro-3,4,6-tri-O-benzyl-1,2-didésoxy-d-arabino-hex-1-énitol on présence d'acides de Lewis, Carbohydr. Res., 1977, 56(1), 168–172 CrossRef CAS.
- J. Ø. Duus, C. H. Gotfredsen and K. Bock, Carbohydrate Structural Determination by NMR Spectroscopy: Modern Methods and Limitations, Chem. Rev., 2000, 100(12), 4589–4614 CrossRef CAS.
-
(a) I. Fukuhara, R. Matsubara and M. Hayashi, Selective Synthesis of Some Aminosugars via Catalytic Aminohydroxylation of Protected 2,3-Unsaturated d-Gluco- and d-Galacto-2-hexenopyranosides, J. Org. Chem., 2020, 85(14), 9179–9189 CrossRef CAS;
(b) P. V. Murphy, J. L. O'Brien and A. B. Smith, Stereospecific synthesis of β-d-allopyranosides by dihydroxylation of β-d-erythro-2,3-dideoxyhex-2-enopyranosides, Carbohydr. Res., 2001, 334(4), 327–335 CrossRef CAS PubMed;
(c) Z. J. Liu, M. Zhou, J. M. Min and L. H. Zhang, Syntheses of 5′-O-glycosylnucleosides, Tetrahedron: Asymmetry, 1999, 10(11), 2119–2127 CrossRef CAS;
(d) T. M. B. de Brito, L. P. da Silva, V. L. Siqueira and R. M. Srivastava, Synthesis of 2,3-unsaturated 4-Amino Sugars and Cyclohexyl 2,3-Di-O-Acetyl-4,6-Di-O-Methyl-α-D-Manno-Pyranoside from Cyclohexyl 4,6-Di-O-Acetyl-2,3-Dideoxy-α-D-erythro-Hex-2-Enopyranoside, J. Carbohydr. Chem., 1999, 18(6), 609–616 CrossRef CAS;
(e) R. W. Friesen and S. J. Danishefsky, On the use of the haloetherification method to synthesize fully functionalized disaccharides, Tetrahedron, 1990, 46(1), 103–112 CrossRef CAS;
(f) H. H. Baer and L. Siemsen, Synthesis of 2-amino-2-deoxy- and 3-amino-3-deoxy-α-d-mannopyranosyl α-d-mannopyranoside by sequential osmylations of a dienic disaccharide, Carbohydr. Res., 1986, 146(1), 63–72 CrossRef CAS;
(g) A. M. Gomez, F. Lobo, S. Miranda and J. C. Lopez, A Survey of Recent Synthetic Applications of 2,3-Dideoxy-Hex-2-enopyranosides, Molecules, 2015, 20(5), 8357–8394 CrossRef CAS;
(h) M. Fukudome, T. Shiratani, Y. Nogami, D.-Q. Yuan and K. Fujita, Shortcut Synthesis of β-Cyclomannin from β-Cyclodextrin, Org. Lett., 2006, 8(25), 5733–5736 CrossRef CAS.
- C. Masson, J. Soto and M. Bessodes, Ferric Chloride: a New and Very Efficient Catalyst for the Ferrier Glycosylation Reaction, Synlett, 2000, 9, 1281–1282 Search PubMed.
- R. D. Dawe and B. Fraser-Reid, α-C-Glycopyranosides from Lewis acid catalysed condensations of acetylated glycals and enol silanes, J. Chem. Soc., Chem. Commun., 1981, 22, 1180–1181 RSC.
- M. Koreeda, T. A. Houston, B. K. Shull, E. Klemke and R. J. Tuinman, Iodine-catalyzed Ferrier Reaction 1. A Mild and Highly Versatile Glycosylation of Hydroxyl and Phenolic Groups1, Synlett, 1995, 1, 90–92 CrossRef.
- P. Chen and S. Li, Y(OTf)3 as a highly efficient catalyst in Ferrier Rearrangement for the synthesis of O- and S-2,3-unsaturated glycopyranosides, Tetrahedron Lett., 2014, 55(42), 5813–5816 CrossRef CAS.
- P. Chen and J. Su, Gd(OTf)3 catalyzed preparation of 2,3-unsaturated O-, S-, N-, and C-pyranosides from glycals by Ferrier Rearrangement, Tetrahedron, 2016, 72(1), 84–94 CrossRef CAS.
-
(a) Q. Wang, T. R. Chan, R. Hilgraf, V. V. Fokin, K. B. Sharpless and M. G. Finn, Bioconjugation by Copper(I)-Catalyzed Azide-Alkyne [3 + 2] Cycloaddition, J. Am. Chem. Soc., 2003, 125(11), 3192–3193 CrossRef CAS;
(b) V. V. Rostovtsev, L. G. Green, V. V. Fokin and K. B. Sharpless, A Stepwise Huisgen Cycloaddition Process: Copper(I)-Catalyzed Regioselective “Ligation” of Azides and Terminal Alkynes, Angew. Chem., Int. Ed., 2002, 41(14), 2596–2599 CrossRef CAS;
(c) H. C. Kolb, M. G. Finn and K. B. Sharpless, Click Chemistry: Diverse Chemical Function from a Few Good Reactions, Angew. Chem., Int. Ed., 2001, 40(11), 2004–2021 CrossRef CAS;
(d) R. Huisgen, 1,3-Dipolar Cycloadditions. Past and Future, Angew. Chem., Int. Ed., 1963, 2(10), 565–598 CrossRef.
- G. Zemplén and E. Pacsu, Über die Verseifung acetylierter Zucker und verwandter Substanzen, Ber. Dtsch. Chem. Ges., 1929, 62(6), 1613–1614 CrossRef.
- D. V. Yashunsky and A. V. Nikolaev, Hydrogenphosphonate synthesis of sugar phosphomonoesters, J. Chem. Soc., Perkin Trans. 1, 2000,(8), 1195–1198 RSC.
- H. E. Gottlieb, V. Kotlyar and A. Nudelman, NMR Chemical Shifts of Common Laboratory Solvents as Trace Impurities, J. Org. Chem., 1997, 62(21), 7512–7515 CrossRef CAS PubMed.
- S. Kopitzki, K. J. Jensen and J. Thiem, Synthesis of Benzaldehyde-Functionalized Glycans: A Novel Approach Towards Glyco-SAMs as a Tool for Surface Plasmon Resonance Studies, Chem.–Eur. J., 2010, 16(23), 7017–7029 CrossRef CAS PubMed.
|
This journal is © The Royal Society of Chemistry 2022 |