DOI:
10.1039/D2RA04589C
(Review Article)
RSC Adv., 2022,
12, 29253-29290
1,4-Dihydropyridine: synthetic advances, medicinal and insecticidal properties
Received
23rd July 2022
, Accepted 21st September 2022
First published on 12th October 2022
Abstract
1,4-Dihydropyridine (1,4-DHP) is one of the foremost notable organic scaffolds with diverse pharmaceutical applications. This study will highlight recent accomplishments in the construction of 1,4-DHP with structural and functional modifications using multi-component one-pot and green synthetic methodologies. The various intrinsic therapeutic applications, ranging from calcium channel blocker, anti-oxidative, anticancer, anti-inflammatory, anti-microbial, anti-hypertensive, anti-diabetic, anticoagulants, anti-cholinesterase, neuro-protective, and other miscellaneous activities, have been summarized with a focus on their structure–activity relationship (SAR) investigations. In addition, the insecticidal properties have been collated and discussed. Researchers in the fields of medicinal chemistry and drug development will find the summarized conclusions of this study incredibly informative, instructional, and valuable.
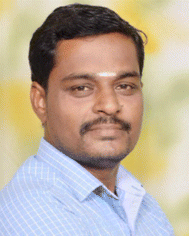 Parthiban A. | Dr A. Parthiban is currently working as a Research Associate Scientist-III in the Centre for Excellence on GMP Extraction Facility, National Institute of Pharmaceutical Education and Research, (NIPER)-Guwahati, Assam, India. He was born in the village of Marudadu, Thiruvannamalai district in Tamil Nadu, India, and his main research interest is in the areas of Synthetic Organic and Medicinal Chemistry. He received his BSc degree from the Department of Chemistry, Arignar Anna Govt. Arts College, Cheyyar, affiliated with the University of Madras in 2005, and MSc degree from the Department of Chemistry, Annamalai University, Tamil Nadu in 2007. He received his PhD degree in Medicinal Chemistry from the Department of Chemistry, Pondicherry University, Puducherry in 2015. He was selected as a postdoctoral fellow at the Orchid Pharmaceutical Company, Chennai from 06.05.2015 to 31.05.2016, and he subsequently worked as an Assistant Professor of Chemistry at Karpagam University, Coimbatore and Vinayaka Missions Research Foundations, Chennai. He also worked as a scientist from 2018 to 2021 at NCSCM, Anna University campus, Chennai. He was awarded the honor of CSIR-NET, GATE and Young Scientist by the Government of India. He served as a reviewer for various international journals published by Springer, Taylor Francis and Elsevier. He has published more than 30 research articles, and also delivered several invited scientific lectures in various institutions. |
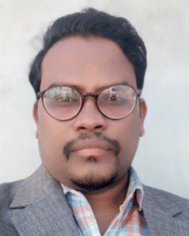 Parameshwar Makam | Dr Parameshwar Makam is a native of Achampet, Nagar Kurnool district in Telangana, India. His main research interest is in the areas of Synthetic Organic and Medicinal Chemistry. He received his MSc from JNT University, Hyderabad, Telangana in 2007, and PhD in Medicinal Chemistry from the Department of Chemistry, Pondicherry University, Pondicherry in 2015. He did his postdoctoral research under the guidance of Professor Per I. Arvidsson in UKZN, South Africa. He subsequently worked as an Assistant Professor of Chemistry at Lovely Professional University and Uttaranchal University, India. He also worked at Mylan Laboratories Limited as a scientist. His scientific achievements include several invited talks at different institutions and serving as a reviewer for international journals published by Springer, Nature Research Group, Taylor Francis, and Elsevier. He has also published fifteen research papers, received three patents, and received six awards for research presentations. He is also a Member of the Royal Society of Chemistry (MRSC), London, UK and a Life-time Member of Indian Science Congress (ISCA). |
1. Introduction
Pyridine has remained one of the most remarkable scaffolds in chemistry since its discovery owing to its broad range of uses in fields ranging from medicine to materials.1,2 The reduction of the unsaturated double bond(s) forms major scaffolds, which facilitates their wide variety of applications, among the several successful pyridine chemical transformations. Different scaffolds have been created based on the number of double bonds that are decreased. Piperidine (three double bonds), tetrahydropyridines (two double bonds), and dihydropyridines (DHPs) are examples of these compounds (one double bond). These scaffolds have been found in a variety of natural and synthetic substances, with a proclivity for inducing molecules with striking and unusual pharmacological effects. The semi-saturation of one pyridine double bond with two substituents can theoretically generate five isomeric structures of nitrogen heterocyclic DHPs, although the 1,2-DHPs and the 1,4-DHPs are the most prevalent and significant.3 Scheme 1 depicts the reductive chemical transformation of pyridine, and the structural characteristics of the 1,2-DHP and 1,4-DHP scaffolds.
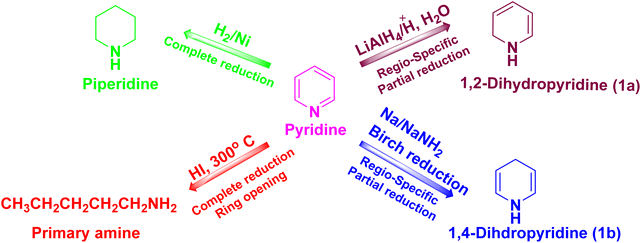 |
| Scheme 1 The possible reductions of pyridine and generation of the 1,2-DHP (1a) and 1,4-DHP (1b) scaffolds. | |
The 1,4-DHP scaffold has gained traction as a preferred choice of medicinal chemistry researchers for developing novel molecules with therapeutic significance, and it is regarded as one of the privileged structures in the drug discovery process due to its ability to import valuable medicinal properties into molecules.4–10 Privilege structures, according to the IUPAC definition, exhibit “substructural diversity, which reveal required (often drug-like) activities on molecules comprising that feature. Mostly include a semi-rigid skeleton, on which can exist numerous hydrophobic residues without undertaking hydrophobic collapse”.11 Research data on 1,4-DHP derivatives have been collected from PubMed, an open-access database, and the statistics of the data are depicted in Fig. 1. The data indicate that researchers have been working on the development of newer 1,4-DHP derivatives since 1969. From 1970 to the 1990s, publications grew dramatically; after that, they began to decline. The largest number of research publications published in a year in the previous ten years was 60, which is somewhat disappointing. Having accessible, thoroughly evaluated information on this research area is one approach to enhancing the research in this field. There have been few attempts at a complete study of the 1,4-DHPs,12 and only a handful have been discovered with a specific focus on therapeutic regions.4,13 There is no study that we are aware of that focuses on the insecticidal agrochemical applications of 1,4-DHP derivatives. To draw researchers' attention to this field, a complete evaluation of the literature on synthetic advances in producing different 1,4-DHP derivatives and their medicinal and agrochemical applications are necessary. This review provides a summary of the current advances in the development of synthetic techniques for 1,4-DHP derivatives and the numerous intrinsic medicinal applications, with an emphasis on their structure–activity relationship (SAR) studies. Additionally, the insecticidal applications as agrochemicals have been compiled and reviewed.
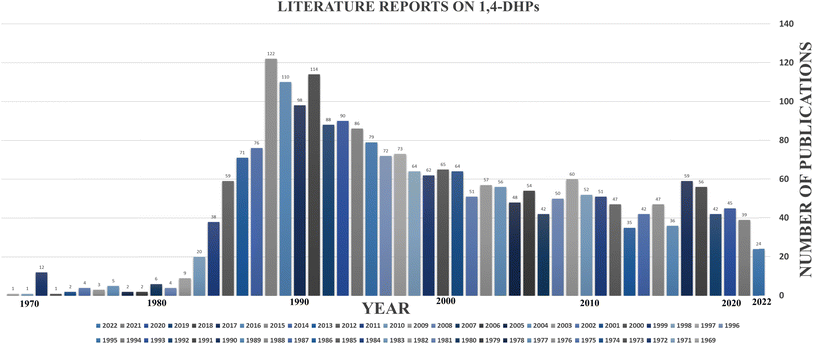 |
| Fig. 1 The number of publications published on 1,4-DHP scaffolds since 1969 (https://pubmed.ncbi.nlm.nih.gov/?term=1%2C4-dihydropyridine). | |
2. Synthetic advances
2.1. The classic reaction: the Hantzsch reaction
A multi-component reaction (MCR) is used in this process, in which two or more starting elements react together to produce pure results.14–16 The key benefits of MCRs are that they are environmentally benign, green, and long-lasting reactions for the synthesis of biomolecules from readily available starting materials. Bond breaking and making occur concurrently in a single reaction in MCRs, resulting in the formation of multiple bonds.17,18 That reaction produces selected products with a high yield and purity according to MCR's technique. MCRs are becoming increasingly attractive in the production of bioactive chemicals. The Hantzsch reaction, a classic and iconic MCR established for the synthesis of physiologically active heterocyclic (N, S, and O) compounds,19 was first used to make 1,4-DHP (1) (Scheme 2), in which one mole of an aldehyde 1a reacts with two moles of acetoacetic ester 1b and an amine 1c to undergo a four-component, one-pot reaction.
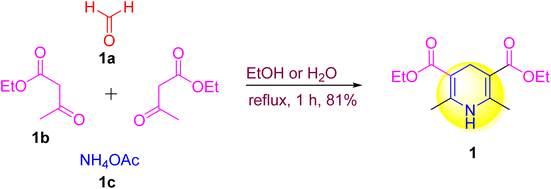 |
| Scheme 2 The Hantzsch synthetic method, a classical 1,4-DHP synthesis. | |
2.2. A decade of advancements in 1,4-DHP synthetic protocols
Owing to the broad range of possible options in synthetic methods development and the construction of structurally varied 1,4-DHP compounds, the Hantzsch method for the synthesis of 1,4-DHPs has swiftly acquired popularity. To maximize its value, several researchers have been working on future advancements. By desymmetrizing enantio-selective bromination, Han et al. (2022) discovered an effective approach for the synthesis of enantio-enrich Hantzsch type 1,4-DHPs 2 starting with symmetrical 1,4-DHPs 2a with the methyl group at the second or sixth position. The inert C–H bond was changed into the adaptable C–Br bond, ensuring high efficiency in the alteration of the chiral 1,4-DHP derivatives (Scheme 3).20 According to the location of the azinium ion that is attacked, Brien et al. (2022) detailed the gold(I)-catalyzed nucleophilic allylations of pyridinium and quinolinium ions 3a with various allyl pinacol boronates 3b to yield diverse functionalized 1,4-DHPs 3. Allyl gold(I) intermediates created by transmetalation from allyl boronates are entirely selective for the reactions. The reactions have unique regioselectivity for attacking the substrates' fourth position, and do not require any additional safety measures to keep out air or moisture (Scheme 4).21 A fluorescent hydrazine hydrate probe (DMA) was created by Xue et al. (2021) using 1,4-DHP derivatives 4 that were produced from a variety of aldehydes 4a, malonaldehyde 4b in the presence of hydrazine hydrate 4c. This probe's fluorescence emission peak is in the near-infrared (667 nm) range, which exhibits strong hydrazine hydrate selectivity. The probe demonstrates aggregation-induced emission (AIE) properties, which is significant. To identify hydrazine hydrate in the solution and achieve the quantitative detection of hydrazine hydrate gas, the probe is also equipped with a portable test paper. This probe is capable of quickly and accurately detecting hydrazine hydrate on both a qualitative and quantitative level (Scheme 5).22 In the presence of benzene sulphonic acid 5b and pyridines, Schiller et al. (2022) reported the amlodipine-based acetylated DHP 5A using acetyl chloride and benzylated 1,4-DHP 5B using phenyl acetyl chloride. Amlodipine 5a, at a high dose of 300 μM, showed an inhibitory impact on the four newly synthesized 1,4-DHPs evaluated at hP2X5FL. Low micromolar ATP-induced currents were increased by isradipine and even more nimodipine. The 1,4-DHP derivatives of amlodipine are not effective hP2X5 antagonists, but amlodipine itself may be used as a starting material for future synthesis to improve its affinity. A stimulatory effect on inflammatory processes may also be a side effect of nimodipine therapy (Scheme 6).23
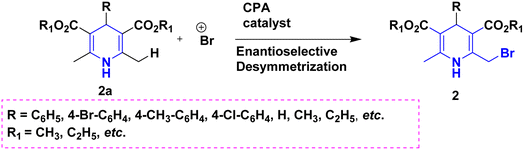 |
| Scheme 3 Hyphothesis for preparation of chiral Hantzsch type 1,4-DHPs. | |
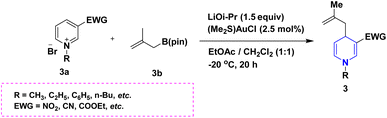 |
| Scheme 4 Nucleophilic allylation of azinium ions. | |
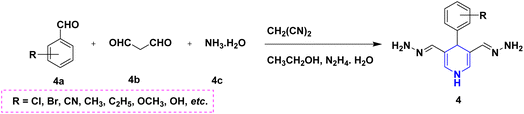 |
| Scheme 5 Synthesis of 1,4-DHP using the fluorescent probe. | |
 |
| Scheme 6 Synthesis of amlodipine 1,4-DHP derivatives. | |
Using alkyne 1,4-DHPs 6a and substituted azides 6b for the synthesis of functionalized triazoles, Bijani et al. (2022) described a copper-catalyzed azide–alkyne cyclization (CuAAC) reaction for the synthesis of a library of 1,4-DHP-based 1,2,3-triazole derivatives 6 and assessed their cytotoxic potential on colorectal adenocarcinoma. The findings suggested that the majority of these drugs exhibit substantial antiproliferative action, with IC50 values ranging from 0.63 ± 0.05 to 5.68 ± 0.14 μM. The available findings suggest that powerful anticancer compounds can be made from 1,2,3-triazole conjugates coupled with DHP (Scheme 7).24 Malhi et al. (2022) described a series of 1,4-DHPs with a carboxylic moiety to synthesize 2,2′-[3,5-bis(ethoxycarbonyl)-4-phenyl-1,4-dihydropyridine-2,6-diyl]diacetic acid 7, the unique dicarboxylic derivatives of 1,4-DHP obtained through the electrocarboxylation of tetrasubstituted-1,4-DHP derivatives 7a. Additionally, the synthesized compounds exhibit superb activity in a variety of bacteria. Their activity against E. coli, S. aureus, B. subtilis, A. niger, and P. glabrum was found to be very effective at 4 μg mL−1 in comparison to conventional doses of amoxicillin and fluconazole (Scheme 8).25 In a straightforward one-pot synthesis involving three components of aromatic aldehydes 8a, methyl propiolate 8b, and a benzylamine 8c, Döring et al. (2021) reported that the novel 1,4-DHPs 8 are accessible. While those generated with the dicarbonyl molecule have alkyl substituents at the corresponding 1,4-DHP positions, their target was to generate compounds that have no substituents in the second and sixth positions. In an MRP4-overexpressing cell line experiment, all compounds displayed superior activities to the most well-known MRP4 inhibitor MK571, and the activities may be attributed to the varied aromatic residue substitution patterns within the symmetric molecular framework (Scheme 9).26 In a further reaction involving 2-chlorobenzaldehyde 9a and cyanothioacetamide 9b in the presence of an excess of N-methylmorpholine, Kurskova et al. (2022) reported on the production of 2-amino-4-(2-chlorophenyl)-6-(dicyanomethyl)-1,4-dihydropyridine-3,5-dicarbonitrile 9 (Scheme 10).27
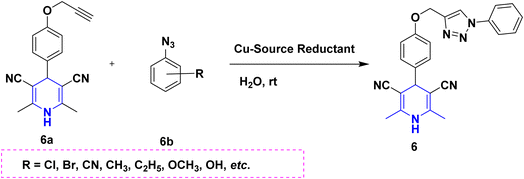 |
| Scheme 7 Synthesis of 1,4-DHPs based triazole derivatives. | |
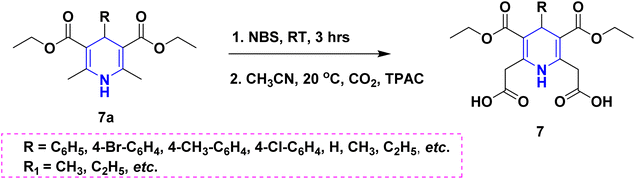 |
| Scheme 8 Synthesis of dicarboxlyic-1,4-DHP derivatives. | |
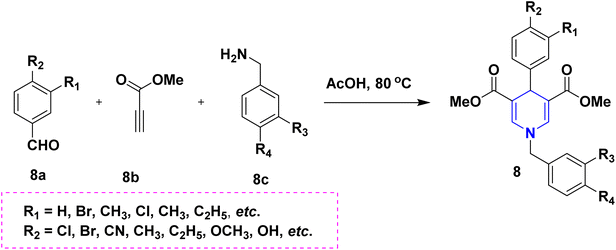 |
| Scheme 9 Synthesis of symmetrical 1,4-DHPs. | |
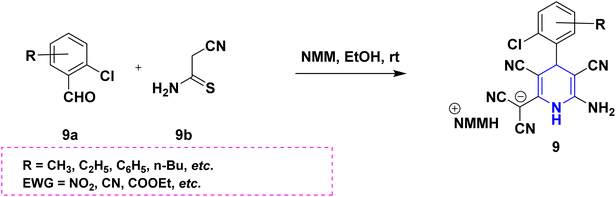 |
| Scheme 10 Synthesis of 1,4-DHPs using NMO. | |
In a condensation procedure involving certain aldehyde derivatives 10a, ethyl acetoacetate 10b, and ammonium acetate 10c in the presence of superparamagnetic manganese ferrite nanoparticles at 80 °C, Moradi et al. (2022) described the effective one-pot synthesis of several 1,4-DHPs 10. The advantages of this method for synthesizing 1,4-DHPs include selectivity, high product purity, outstanding yields, quick reaction times, ease of processing, and environmentally favorable settings. Furthermore, the product yield can be slightly decreased by recovering and reusing the catalyst across several runs (Scheme 11).28
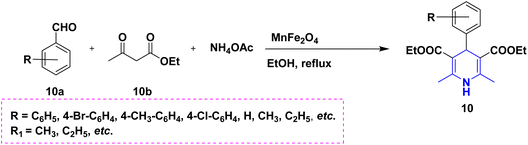 |
| Scheme 11 Synthesis of 1,4-DHPs by MnFe204 nanoparticles. | |
For the first time, Patra et al. (2022) took advantage of the activated pyridinium salts with, -difluorinated gem-diols 11a, creating a highly regioselective and simple synthesis of 1,4-DHPs ornamented with a high-value gem-difluoro methylene motif 11 in high yields. The protocol has a wide substrate scope, is scalable, and is compatible with many different functional groups. The DHP and pyridone compound 11B that were thus produced were particularly noteworthy because they could be used to create densely functionalized oxaazabicyclo[3.3.1]nonane scaffolds (Scheme 12).29 The Hantzsch-like process was used to synthesize a novel series of diethyl 3,5-dicarboxylate derivatives of 1,4-DHPs 12A. As raw materials, aromatic aldehydes 12a, aromatic amines 12c, and ethyl propiolate 12b were used to construct and refine the synthetic methodology shown here. The diethyl-3,5-dicarboxylate of 1,2-DHP, 12B, was discovered as an unanticipated by-product in this synthesis process (Scheme 13).30 The novel compounds, diethyl 3,5-dicarboxylate derivatives of 1,4-DHPs 13, have been reported using an array of aromatic aldehydes 13a, ethylaceto acetate 13b, and urea 13c in the presence of sub-critical (SBC) and ethanol as a solvent medium (Scheme 14). This study is significant since it takes less time, uses a traditional work-up, and does not use column chromatography.31
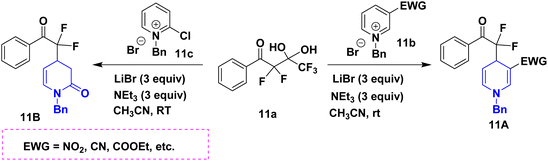 |
| Scheme 12 Synthesis of 1,4-DHPs adorned with gem-difluoromethylene motif. | |
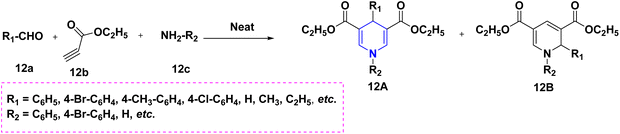 |
| Scheme 13 Synthesis of 1,4-DHPs using neat conditions. | |
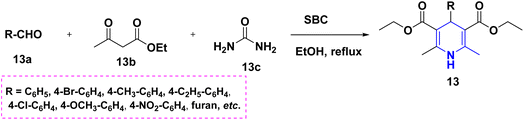 |
| Scheme 14 Synthesis of 1,4-DHPs using SBC conditions. | |
Radioactive 13N-labeling experiments were carried out by Blower et al. (2021). The findings were interesting and are quite useful for the radiochemical synthesis of [13N] 1,4-DHP 14 derivatives using several benzaldehydes 14a and β-keto ester 14b in the presence of radiolabeling ammonia 14c (Scheme 15). The 1,2,4-triazole-tagged 1,4-DHP derivatives 15 were developed with a highly efficient, green MCR protocol by the multicomponent reaction, viz., aldehydes 15a, 1H-1,2,4-triazol-3-amine 15d, diethyl acetylene dicarboxylate 15b and active methylene compounds 15c. Interestingly, the reactions took only 12 minutes to complete in a green water solvent under microwave (MW) conditions (Scheme 16). Excellent yields (94–97%), functional group tolerance, and selectivity were all achieved throughout the reaction.32 Similarly, the condensation of benzaldehyde 16a, ethyl acetoacetate 16b, and ammonia 16c in the presence of aqueous ethanol at room temperature was described as a visible light-mediated technique for the production of poly substituted 1,4-DHPs, 16. When compared to thermal procedures, this approach has the benefit of minimizing byproduct generation and having quicker reaction times (Scheme 17).33 By using the same starting ingredients, such as benzaldehyde and ethyl acetoacetate, 1,4-DHPs, 16 are synthesized in the presence of Fe nanoparticles34 and magnesium nitride.35 By using ionic liquid and MW mediation, the catalytic efficiency of glycine nitrate (GlyNO3) was investigated and developed into a multicomponent reaction of 1,4-DHPs 17 starting from aromatic or heteroaromatic symmetrical or unsymmetrical aldehydes 17a, methyl acetoacetate 17b, and ammonium carbonate 17c (Scheme 18). The use of ionic liquid glycine nitrate to boost product yields was a key feature of this research.36
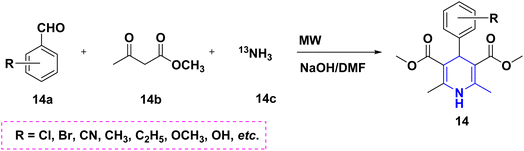 |
| Scheme 15 Synthesis of 1,4-DHP using microwave conditions. | |
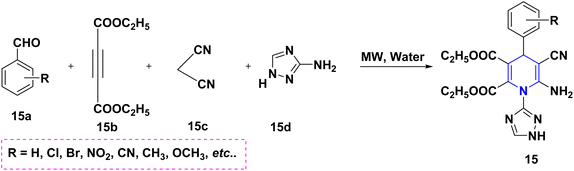 |
| Scheme 16 Synthesis of 1,4-DHP using microwave in water conditions. | |
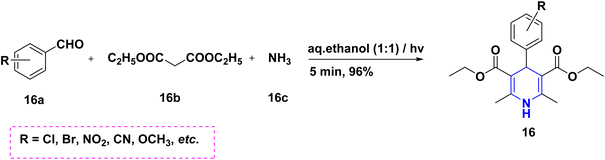 |
| Scheme 17 Synthesis of 1,4-dihydropyridine in visible light. | |
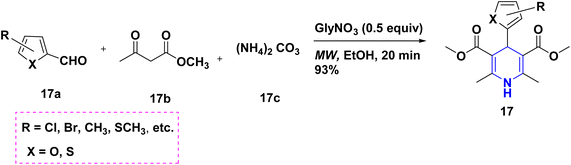 |
| Scheme 18 Glycine nitrate ionic liquid catalyzed synthesis of 1,4-dihydropyridines. | |
In 2011, Gestwicki and coworkers devised a synthetic technique for highly functionalized 1,4-DHPs. In the MCR of benzaldehyde 18a, ethyl acetoacetate 18b, dimedone 18c, and ammonium acetate 18d, Yb(OTf)3 is utilized as a Lewis acid catalyst. With good yields, this approach has been tested with a variety of aldehydes, including aliphatic, aromatic, and bulky aldehydes. This research is significant since it confirms the use of Yb(OTf)3, a stable rare-earth metal, as a catalyst (Scheme 19).37 A solid support biodegradable cellulose sulphuric acid (CSA) was applied in the non-toxic and reusable catalyzed water-mediated one-pot synthesis of C5-unsubstituted 1,4-DHPs, 19 with excellent yields using chalcones 19a, ethyl acetoacetate 19b and ammonium acetate 19c (Scheme 20).38 The development of highly economical cellulose sulphuric acid as a catalyst and C5-unsubstituted 1,4-DHPs are the high points of this work. The palladium(II) catalyzed cascade reaction of 1,4-DHPs 20 started from substituted benzaldehyde, 20a, aniline 20b and electron-deficient alkenes 20c in the presence of O2 atmosphere (Scheme 21).39 The highlight of the method is an exploration of an effective Pd-catalyst to provide anti-Markovnikov products using primary anilines and alkenes. The unsymmetrical 1,4-DHPs 21 has been investigated from enaminones 21a, benzaldehyde 21b, and 1,3-cyclohexanedione 21c in the presence of neat conditions (Scheme 22).40 The access to a complex product, green solvent and no column chromatography method are some of the advantages of this technique.
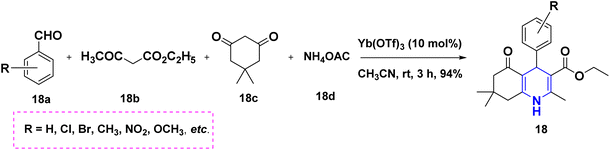 |
| Scheme 19 Yb(OTf)3 Lewis acid-catalyzed synthesis of 1,4-DHPs. | |
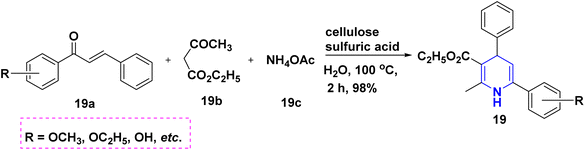 |
| Scheme 20 The cellulose sulphuric acid medicated synthesis of C5-unsubstituted 1,4-DHPs. | |
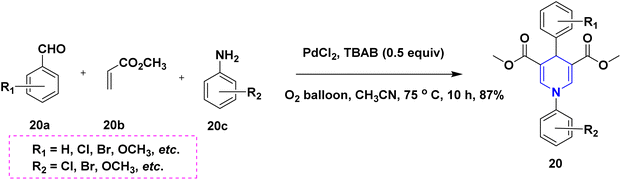 |
| Scheme 21 Palladium-assisted synthesis of 1,4-DHPs. | |
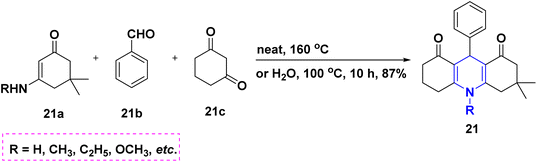 |
| Scheme 22 Synthesis of unsymmetrical 4-arylacridinediones under neat condition. | |
Benzaldehyde 22a, quaternary ammonium hydrochloride salt 22b, and acetals 22c react to form 1,4-DHP 22 with an 80% yield without the aid of a catalyst. The synthesized 1,4-DHPs also displayed a diversity of fluorescence wavelengths as a result of various derivatives at the third and fifth positions (Scheme 23).41 The one-pot cascade reaction of benzaldehyde 23a, malononitrile 23b, arylamine 23c, and acetylene dicarboxylate 23d provide highly substituted 1,4-DHPs 23 with excellent yields, as shown in (Scheme 24).42 Trifluoroacetic acid (TFA) catalyzed the synthesis of 1,4-DHPs 24 via domino reaction of p-nitrobenzaldehyde 24b, enaminones 24a (Scheme 25).43
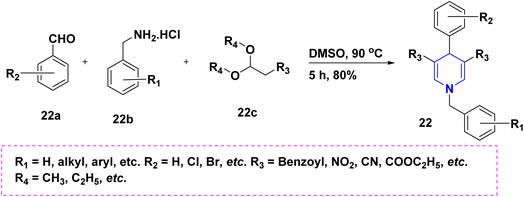 |
| Scheme 23 Three-component reaction for the generation of 1,4-DHPs. | |
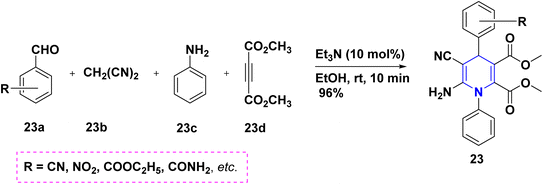 |
| Scheme 24 Et3N catalysed synthesis of polysubstituted 1,4-DHPs. | |
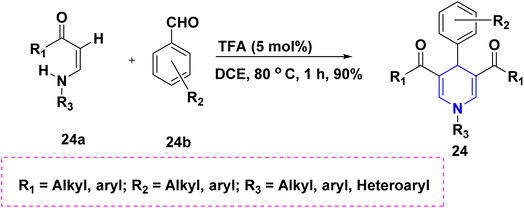 |
| Scheme 25 TFA catalyzed synthesis of 1,4-DHPs. | |
By reacting different benzaldehydes 25a, malononitrile 25b, and cyclic nitroketene N,S-acetals 25c with a triethylamine (Et3N) base, it is possible to create the highly substituted thiazolo[3,2-a]pyridines 25. The focus of this work is the investigation of N,S-acetals and their derivatives (Scheme 26).44 An Et3N-mediated one-pot cascade ring formation reaction towards the synthesis of imidazo-[1,2-a]thiochromeno[3,2-e]pyridines 26 started from substituted N,S-acetals 26a, ethyl cyanoacetate 26b and benzaldehydes 26c under neat conditions (Scheme 27).45 p-Toluenesulfonic acid (PTSA) catalyzed the condensation reaction of two moles of 2-formyl-2-nitroenamine 27a and one mole of electron-rich aromatic compound 27b towards the synthesis of C4-substituted 3,5-dinitro-1,4-DHPs 27 (Scheme 28).46
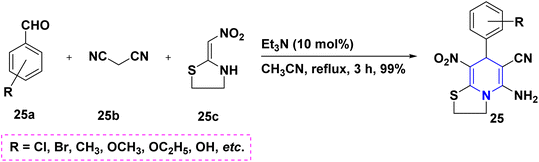 |
| Scheme 26 Synthesis of 3-cyano-5-nitro-substituted 1,4-DHPs. | |
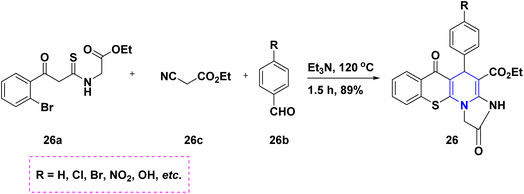 |
| Scheme 27 Synthesis of imidazo[1,2-a]thiochromeno[3,2-e]pyridine. | |
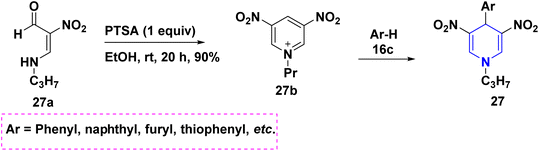 |
| Scheme 28 Synthesis of 3,5-dinitrosubstituted 1,4-DHPs. | |
The one-pot multicomponent reaction of benzaldehyde 28a with ethyl acetoacetate 28b in the presence of metal–organic catalyst HKUST-1 (a type of MOFs with a molecular formula of [Cu3(BTC)2(H2O)n]) towards the synthesis of 1,4-DHPs 28 has been carried out in neat conditions. This protocol affords numerous advantages such as good yields with high purity, no column chromatography, short reaction time and recyclability of the catalyst (Scheme 29).47 A similar synthesis of 1,4-DHPs was obtained by using a recyclable biocatalyst, nicotinic acid48 and alginic acid.49 Similar single pot cascade reactions for the synthesis of 1,4-DHPs have been reported by applying other conditions such as tetrabutylammonium hydrogen sulfate (TBAHS),50 Brønsted acid MIL-101-SO3H51 and MW irradiation.52 A new one-pot cascade reaction of aldehydes 29a, ethyl acetoacetate 29b, and dimedone 29c was carried out to give 1,4-DHPs 29A and 29B using aminated multi-walled carbon nanotubes under heat conditions. The benefits of this method include the recyclable catalyst, easy workup and good yield of products (Scheme 30).53 Ceric ammonium nitrate (CAN) catalyzed the tandem multicomponent reaction of various 1,3-diones and 5-bromothiophene-2-carboxaldehyde 30a, ethyl acetoacetate 30b, for the synthesis of 1,4-DHPs 30 with significant yields (Scheme 31).54
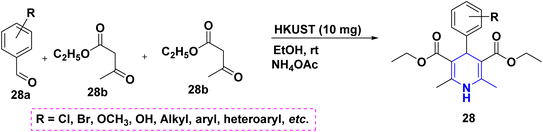 |
| Scheme 29 HKUST-1 catalyzed synthesis of 1,4-DHPs under solvent-free conditions. | |
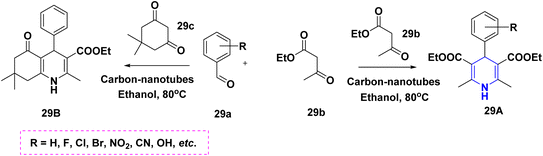 |
| Scheme 30 Preparation of 1,4-DHPs in the presence of aminated MWCNTs. | |
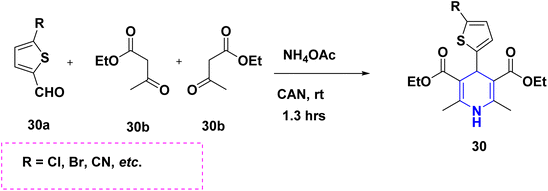 |
| Scheme 31 Synthesis of 1,4-DHP using CAN catalyst. | |
According to Dharma Rao et al. (2018), an effective synthesis of symmetrical 1,4-DHP derivatives 31 was initiated from tert-butyl-β-ketoester 31a, aryl aldehyde 31b, benzyl alcohol 31c, and ammonium carbonate 31d at 70 °C in the presence of a green solvent and under neat conditions (Scheme 32).55 The one-pot domino methodology was established for the synthesis of 4-DHPs carrying a C4 acetate moiety 32. They were made from alkyl propiolates 32a and primary amines 32b in DMSO solvent with piperazine as a base. The application has been realized with ease, and allows for the efficient and high-yield production of 1,4-DHPs (Scheme 33).56 Jieping Wan et al. (2014) described the symmetrical 1,4-DHPs 33 produced in the presence of TMSCl from electron-deficient alkynes 33a, aldehydes 33b, and amines 33c by utilizing a secondary amine for the preparation of N-substituted 1,4-DHPs started from aldehyde 33b with acetylene derivative 33a via enaminoester intermediates (Scheme 34).57
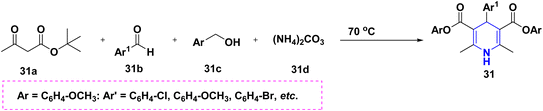 |
| Scheme 32 Synthesis of 1,4-DHP under catalyst-free conditions. | |
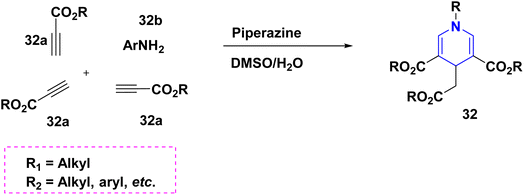 |
| Scheme 33 Synthesis of 1,4-DHP under piperazine catalyst. | |
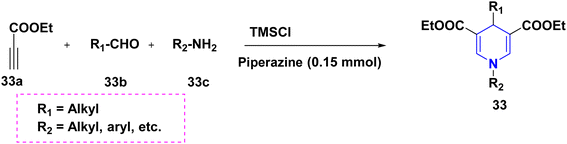 |
| Scheme 34 Synthesis of 1,4-DHP using piperazine (0.15 mmol) and TMSCl catalyst. | |
Zhu et al. (2016) used copper as a less hazardous catalyst and molecular oxygen as the terminal oxidant to synthesize highly substituted 1,4-DHPs 34 generated from N-arylglycine esters 34a and 1,3-dicarbonyl compounds 34b. The key advantage of this one-pot tandem reaction was the excellent product yield (Scheme 35).58 Perumal et al. (2014) described a cascade process towards the synthesis of DHPs 35 generated from aldehydes 35a, malononitrile 35b, amines 35c, and activated alkynes 35d under neat conditions. Huisgen dipolar additions are used in this cascade technique to produce 1,4-DHPs as a single diastereomer (Scheme 36).59 Using PTSA in ethanol and acetonitrile as solvents, Liu et al. (2018) reported an atom economy reaction of enaminones 36b with aldehydes 36a to give symmetrical 1,4-DHPs 36A and 36B. The production of four carbon–carbon bonds and two carbon–nitrogen bonds in a single pot procedure are the highlights of this reaction (Scheme 37).60 Chang-Sheng Yao et al. (2010) developed a one-pot multicomponent process using active methylene 37a, aldehyde 37b, and ketoester 37c for the synthesis of libraries of thiopyrano[3,4-b]pyridine derivatives 37 with shortened reaction times with excellent yields (Scheme 38).61 Li et al. (2013) described a study towards the preparation of a chemo selectivity product of 1,4-DHPs in higher yields 38 by using the simple starting materials such as β-ketothioamides (KTAs), aldehydes 38a and β-enaminonitriles 38b in the presence of AcOH under a neat protocol (Scheme 39).62
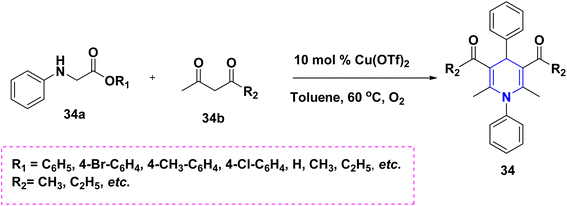 |
| Scheme 35 Synthesis of 1,4-DHPs using Cu(OTf)2 catalyst. | |
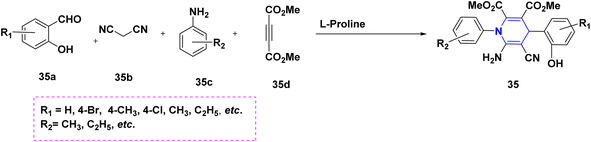 |
| Scheme 36 Synthesis of 1,4-DHP using L-proline catalyst. | |
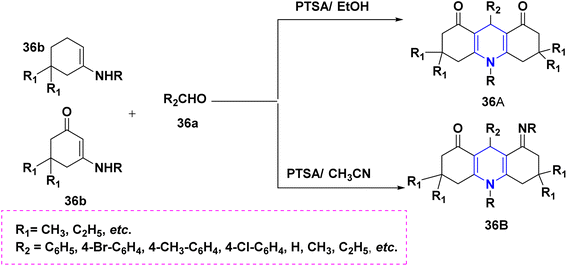 |
| Scheme 37 Synthesis of 1,4-DHP using PTSA. | |
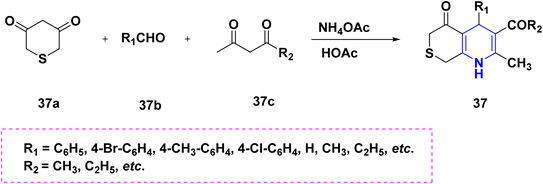 |
| Scheme 38 Synthesis of 1,4-DHP using NH4OAc. | |
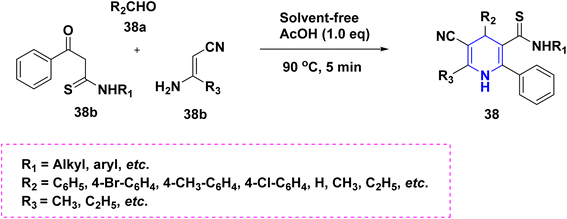 |
| Scheme 39 Synthesis of 1,4-DHP neat conditions. | |
2.3. Limitations of Hantzsch 1,4-DHPs synthesis
Even though the Hantzsch 1,4-DHP synthesis is a well-known reaction, it has a few disadvantages, including the use of only alkyl acetoacetates as substituents, the difficulty of adding an aryl group in the -keto ester, and the need for a distinct nitrogen supply (Scheme 40). Exploration of innovative techniques toward the synthesis of highly functionalized 1,4-DHPs by overcoming these challenges will now receive a lot of attention.
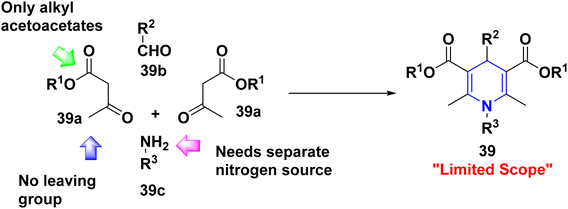 |
| Scheme 40 1,4-DHPs Hantzsch synthesis and limitations. | |
Surya Prakash Rao and Parthiban (2014) investigated the chemistry of N-methyl-1-(methylthio)-2-nitroethenamine (NMSM) 40b as a nitrogen source, and used one-pot MCR to synthesize poly substituted 1,4-DHPs 40 from aldehydes 40a and NMSM 40b with 2-amino pyridine (2-AP) as a base (Scheme 41).63 As illustrated in Scheme 41, a plausible mechanism has been presented. The iminium ion A is formed in the first step when benzaldehyde 40a combines with 2-AP. The iminium ion which interacts with NMSM 40b provides the intermediate B, and swiftly changes to give the unstable intermediate C, which has a more stable nitroketene-N,S-acetal substructure. Intermediate D and 2-AP are formed when intermediate C interacts with one additional unit of NMSM 40b. Finally, methanethiol is eliminated intramolecularly, yielding 1,4-DHP 40. The fact that primary amines stimulate the MCR suggests that iminium ion A is formed as the first intermediate.
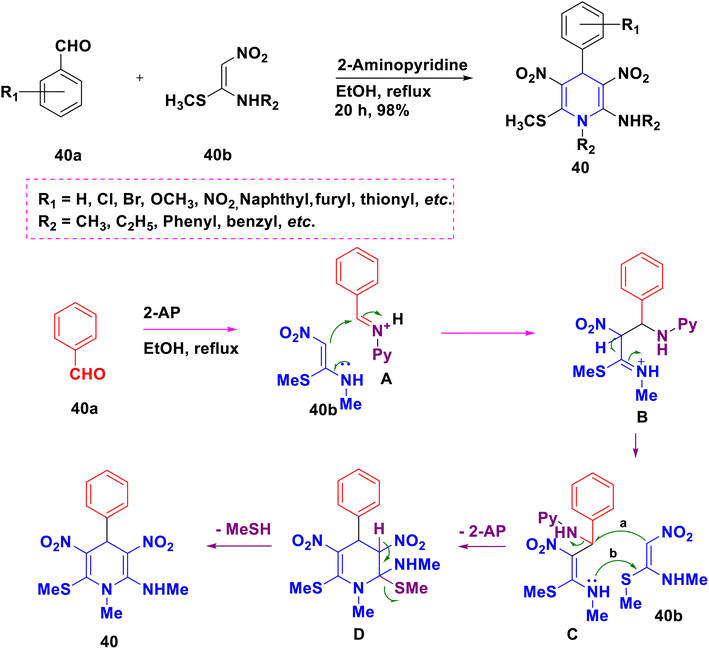 |
| Scheme 41 The pseudo three components for the synthesis of 1,4-DHP and plausible mechanism. | |
2.4. Medicinal importance of 1,4-DHPs
For nearly a century, the 1,4-DHPs have been known for their enormous biological value and uses. Both NADH and NADPH have reactive portions that function as cofactors in enzymes that perform oxidation–reduction processes, while keeping 1,4-DHP in their structures (Fig. 2).64,65 Synthetic 1,4-DHPs have a wide range of therapeutic uses.66,67 As a result of their wide range of applications and vast structural variety, 1,4-DHPs have become a favored scaffold among synthetic and medicinal chemists (Fig. 2).68–72 To aid medicinal chemists and biologists in identifying and rationalizing the molecule's structural and functional importance in inducing the particular activity, some of the most notable medicinally important molecules of 1,4-DHPs, as well as the synthetic protocols used to achieve them, are discussed herein.
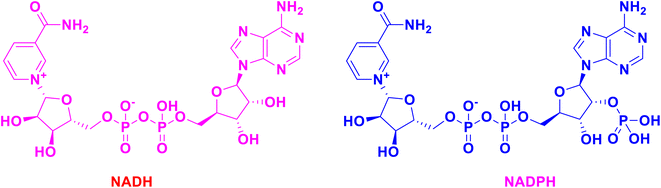 |
| Fig. 2 Structure of NADH and NADPH. | |
2.4.1. Antihypertensive activity. The role of nitrooxyalkylester insertion at the 3- and 5-positions of 1,4-DHPs 41 in exerting antihypertensive action was discovered by Ogawa et al. (1993). As indicated in the scheme, the synthesis involves an MCR reaction between the corresponding aldehyde 41a and the nitrooxyalkylester 41b and amine source 41c. The study discovered CD-349, a chemical compound that is more powerful than the antihypertensive medication, nifedipine (Scheme 42).73 Nekooeian et al. (2009) reported on novel nitroxyalkyl-containing 1,4-DHP derivatives 42 in a similar way. The compounds were tested in two-kidney and one-clip hypertension rat models. In comparison to nifedipine, a commonly used medicine, the two nitroxy group-containing compounds have shown a weak lowering impact on the mean arterial pressure. Because of its putative interaction with Ca channels, the substituent at the fifth position of 1,4-DHP had a significant influence on inducing the antihypertensive ability, according to the SAR. Furthermore, it is established that the presence of the nitroxy group is not required for the action. When compared to nifedipine, a medication with no nitroxy group, there is no indication of a drop in MAP (Scheme 43).74 Hadizadeh et al. (2009) developed and synthesized a new class of imidazolyl comprising 1,4-DHPs 43. DOCA-induced hypertension in rats was used to test the drug's antihypertensive efficacy. The influence of the benzyl ring, particularly the para position replacement with electron-withdrawing groups, was shown in SAR analyses. All of the examined substances improved the antihypertensive properties. The molecule with the fluorine substituent (X = F) was shown to be the most active, and even more effective than the common medication amlodipine (Scheme 44).75 Novel 3-[4-(substituted amino)phenyl]esters containing 1,4-DHP derivatives 44 were developed, produced, and evaluated for antihypertensive efficacy by Ashimori et al. (1991). The results are encouraging, with a longer duration and stronger impact than nicardipine, the usual medicine. Researchers were encouraged by the positive results to pursue the compounds for further pharmacological exploration (Scheme 45).76
 |
| Scheme 42 The antihypertensive activity of CD-349. | |
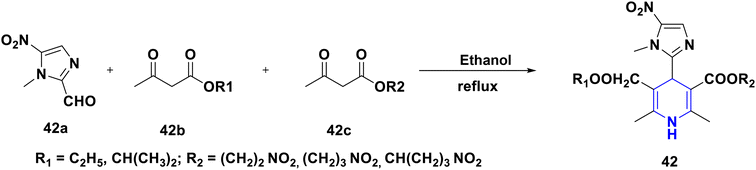 |
| Scheme 43 Antihypertensive activity of nitroxyalkyl 1,4-DHP derivatives. | |
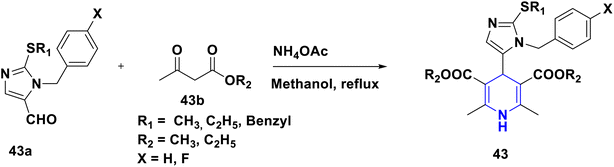 |
| Scheme 44 Antihypertensive activity of 5-imidazolyl DHP derivatives. | |
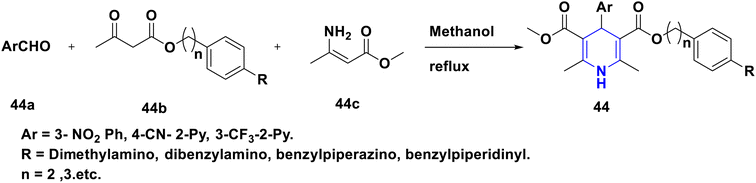 |
| Scheme 45 Antihypertensive acivity of 1,4-DHPs bearing 3-[4-(substituted amino)phenylalkyl]ester derivatives. | |
Kanno et al. (1992) developed and synthesized a variety of novel 1,4-DHPs with amino-2,2-dimethyl-propyl ester groups or 4-disubstituted phenyl and an aminoethyl ester 45, and tested their antihypertensive efficacy in normotensive and spontaneously hypertensive rats. In comparison to nifedipine and nicardipine, several have shown strong activity and a longer duration of their effect. The 2-fluoro-5-nitrophenyl at the fourth position and 2,2-dimethylaminopropylester at the third position in 45 make it the most active compound in this class (Scheme 46).77 For the screening of the antihypertensive activity of their synthesized novel 2-amino-1,4-DHP compounds with nitroxy-alkoxycarbonyl group substitutions at the third or fifth position in 46, Koyabhashi et al. (1995) utilized spontaneously hypertensive rats. Compounds having tert-amino groups on each side of an ester chain have been shown to have a powerful and long-lasting effect, and have been discovered to be more powerful than nifedipine (Scheme 47). Fassihi et al. (2004) described the analogues of nifedipine alkyl ester 47. The 2-methylthio-1-phenylamino-5-imidazolyl group has been substituted for the o-nitrophenyl group at the fourth position in the design. The calcium-channel antagonist properties of the synthesized derivatives were tested utilizing a higher-potassium contraction of guinea-pig ileal longitudinal smooth muscle. More than two methylene chains in the position of the third and fifth ester substituents lowered the activity of the symmetrical aliphatic esters and phenyl alkyl ester series, according to SAR analyses. Overall, the compounds with an isopropyl ester substitution at the third and fifth positions exhibited promising activity in comparison to the standard drug, nifedipine. Amazingly, 47 derivatives were shown to be more effective than nifedipine (Scheme 48).78
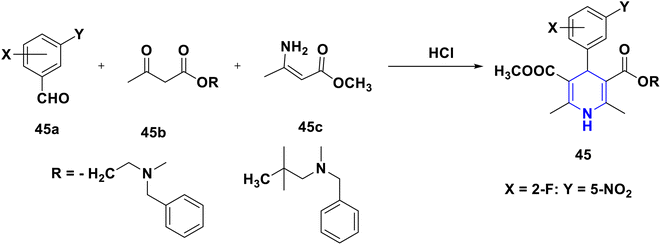 |
| Scheme 46 Antihypertensive activity of 1,4-DHPs bearing 3-aminoethylester derivatives. | |
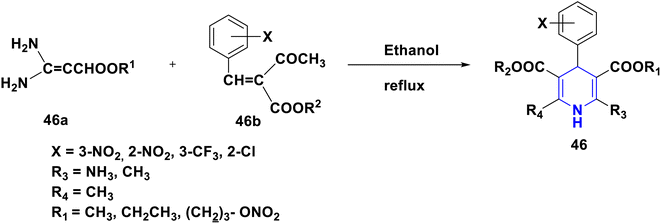 |
| Scheme 47 Antihypertensive activity of 1,4-DHPs bearing nitroxy-alkoxycarbonyl derivatives. | |
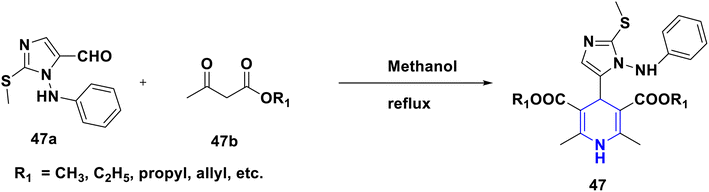 |
| Scheme 48 Antihypertensive activity of 1,4-DHPs bearing 2-methylthio-1-phenylamino-5-imidazolyl derivatives. | |
SAR has been established, and is graphically depicted in Fig. 3 based on the interpretation of the results. 1,4-DHP's central core is a necessary prerequisite for performing the activity. The common core requirement to execute the activity was discovered to be the methyl group substitutions at the second and sixth positions, and the ester substitutions at the third and fifth positions. It was found that 1,4-DHPs with a nitrooxy ester in the third position are more potent than nifedipine. When compared to other compounds that induce activity by electron-withdrawing groups, compounds having a fluoro group at the fourth position of the phenyl ring demonstrated superior activity. Similarly, the substitution of phenyl, 4-(substituted pyridyl) and imidazole at the fourth position have significantly improved activity.
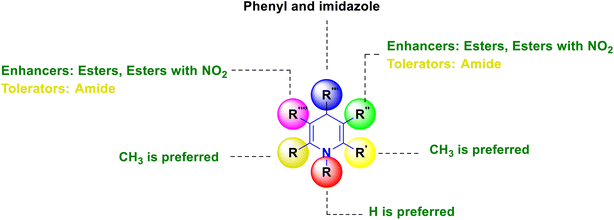 |
| Fig. 3 SAR studies of antihypertensive acitivity of 1,4-DHPs. | |
2.4.2. Calcium channel blockers. Ozer et al. (2018) synthesized cyclohexane ring fused 1,4-DHPs 48, and investigated the effects on the calcium channel blocking activities by substituents with different ester moieties and the benzoyl inserted in the 4-phenyl ring. The observed pharmacological data revealed that, while all compounds are powerful relaxants on the isolated rat aortic smooth muscle, adding a benzyloxy substituent to the phenyl ring reduced their relaxant activity (Scheme 49).79 Bladen et al. (2014) synthesized libraries of a new 1,4-DHP ring structure (hexahydroquinoline) 49, and showed that they can block both L- and T-type calcium channels. Modification of a crucial ester group within this class of compounds not only adjusts the blocking affinity for both L- and T-type channels, but also permits the synthesis of DHPs with 30-fold selectivity for T-type channels over L-type channels. These results indicate that a pharmacophore based on a condensed DHP-based scaffold might be used to develop a novel class of T-type selective inhibitors (Scheme 50) (Fig. 4).80 The SAR investigation on the fourth, fifth, and sixth positions of the series of 1,4-DHPs APJ2708 (50A) published by Yamamoto et al. (2006) identified a new class of N-type calcium channel blockers with fewer cardiovascular side effects than the standards, cilnidipine and APJ2708. The compounds, 4-(3-chloro-phenyl)-2-methyl-6-trifluoromethyl-1,4-dihydropyridine-3,5-dicarboxylic acid 5-(3,3-diphenylpropyl)ester 50B, which exhibited almost identical activity against N-type calcium channels but 1600-fold less activity for L-type than cilnidipine, was among them. The relevance of the inhibitory action against N-type channels in the action of neuropathic pain was reemphasized by this finding (Scheme 51).81 Lipophilic nitroimidazolyl-1,4-DHPs 51 were explored by Miri et al. (2006). It claims that a lipophilic aromatic substitution at the C-6 position of the DHP core improves penetration into the organs, but that replacing both methyl groups in the aromatic ring at both the C-2 and C-6 positions of DHP results in negligible calcium antagonistic activity owing to increased steric hindrance. Due to an extended alkyl chain at the C-3 position, the product 5-ethyl-3-n-propyl-1,4-dihydro-2-methyl-6-phenyl-4-(1-methyl-5-nitro-2-imidazolyl)-3,5-pyridine dicarboxylate 51 demonstrated better activity (Scheme 52).82 Rucins et al. (2014) developed, synthesized, and tested new 1,4-DHP derivatives featuring the cationic pyridine group at the fourth portion and the N-propargyl moiety as a substituent at the first position of the 1,4-DHP 52. The 4-(N-dodecyl)pyridinium group bearing compounds (without the N-propargyl group) and (with the N-propargyl group) had the strongest calcium antagonistic activities with an IC50 value of almost 5–14 μM against neuroblastoma SH-SY5Y and vascular smooth muscle A7r5 cell with an IC50 of 0.6–0.7 μM. This indicated that they primarily targeted L-type calcium channels, and also showed a little free radical scavenging activity overall. 4-(N-Dodecyl)pyridinium-moiety containing molecules might act as prototype molecules for cardio-protective or neuro-protective drugs (Scheme 53).83
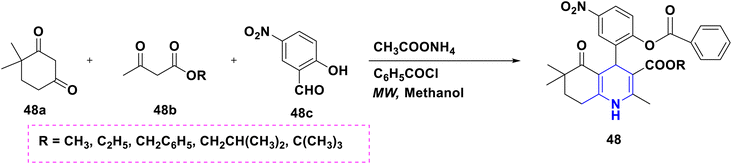 |
| Scheme 49 Cyclohexane ring fused 1,4-DHPs as potential calcium channel blockers. | |
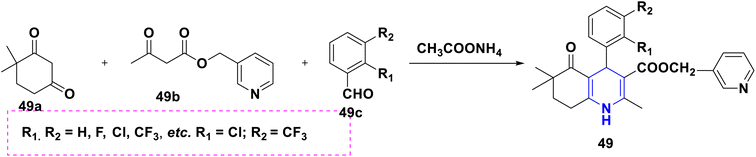 |
| Scheme 50 The condensed 1,4-DHP ring system act as potential calcium channel blockers. | |
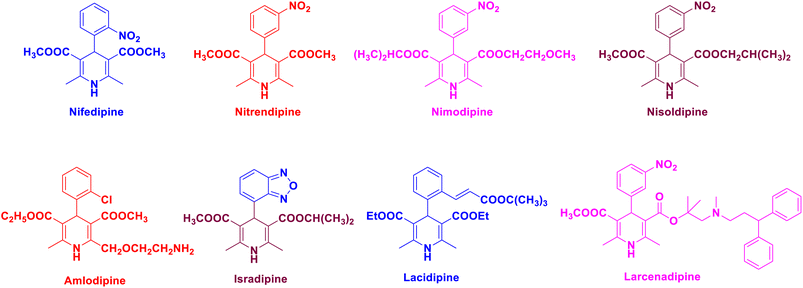 |
| Fig. 4 Structure of 1,4-DHP based channel blockers. | |
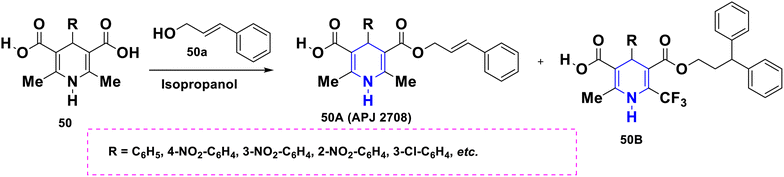 |
| Scheme 51 1,4-DHPs act as potential calcium channel blockers. | |
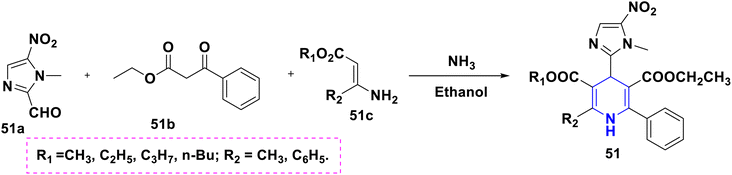 |
| Scheme 52 The nitroimidazoly1-1,4-DHPs as potential calcium channel blockers. | |
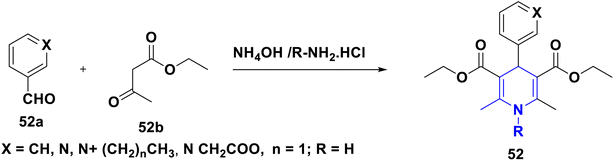 |
| Scheme 53 1,4-DHPs cationic pyridine moiety act as calcium channel blockers. | |
Based on the interpretation of the findings, the SAR has been constructed and is graphically represented in Fig. 5. The central core of 1,4-DHP is a necessity for carrying out the activity. The methyl group substitutions at the second and sixth positions were found to be the collective core requirement to exert the activity. The activity has been reduced as a result of replacing the methyl group with a trifluoromethyl group and phenyl systems. Therefore, the recommended functional group at the second and sixth positions is the methyl group. The required functional group is the ester functional group at the third and fifth positions. The activity has been decreased by using an ester instead of a keto functional group. As a result, the ester functional group is preferred. A considerable impact on the activity has been shown when the phenyl group, a six-membered aromatic system, is favored over imidazole, a five-membered aromatic heterocyclic system, at the fourth position. The relaxant activity of 1,4-DHP was decreased by adding a benzyloxy group to the phenyl ring found in the fourth position. This implies that the molecules become significantly larger when a large ring is added to the phenyl ring, which may reduce their capacity to block calcium channels. The results for the asymmetrical esters showed that lengthening the substituent in the three carbons of the ester substituent increased activity in the alkyl ester analogues of the new derivatives of nifedipine, in which the o-nitrophenyl group at the fourth position is replaced by a 1-methyl-5-nitro-2-imidazolyl substituent and the methyl group at the sixth position is replaced by a phenyl substituent. The activity dropped when the length increased, and while the steric hindrance also increased.
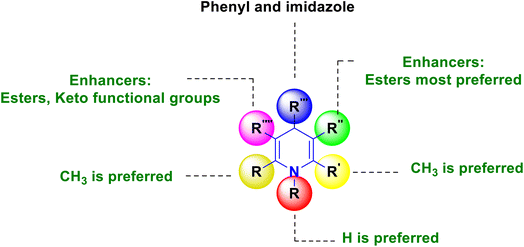 |
| Fig. 5 SAR studies of calcium channel blockers acitivity of 1,4-DHPs. | |
2.4.3. Anticancer agents. Parthiban et al. (2020) designed and synthesized 1,4-DHPs with various structural and functional group changes. SWISSADME has been used to evaluate the physicochemical characteristics and drug-like molecular nature of the compounds. The PTSA (para-toluene sulfonic acid) catalyzed a tandem one-pot synthesis approach from NMSM 53b and related aldehydes 53a using a simple, inexpensive, and environmentally friendly water-mediated process. All of the substances were tested in vitro against lung adenocarcinoma (A549) and laryngeal carcinoma (Hep2) cancer cell lines. The antioxidant capabilities were assessed using the DPPH decrease level (%). According to the SAR studies (Fig. 6), the furan substituted 1,4-DHPs displayed the most potent anti-cancer activity and was almost equal to doxorubicin, a commonly used cancer medication (Scheme 54).84
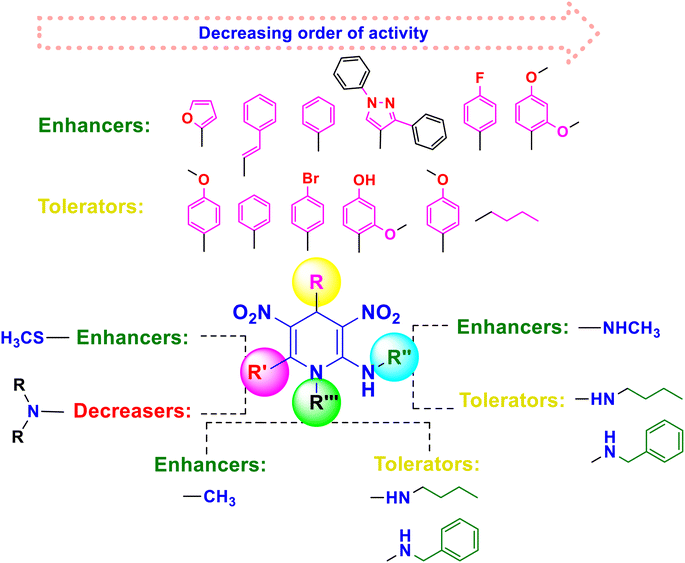 |
| Fig. 6 SAR studies of nitro group containing 1,4-DHPs. | |
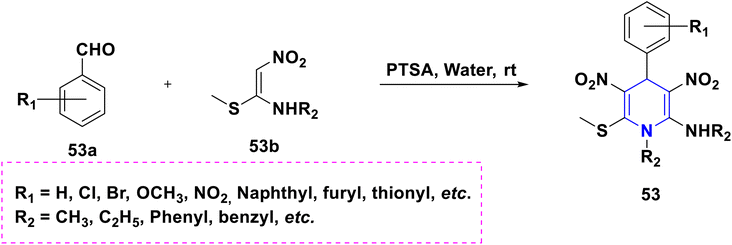 |
| Scheme 54 The nitro substituted 1,4-DHPs as anticancer agents. | |
Gomha et al. (2020) reported a new series of 2,6-dimethyl-4-phenyl-1,4-dihydropyridine-3,5-dicarbohydrazone derivatives (54A–C) started from 2,6-dimethyl-4-phenyl-1,4-dihydropyridine-3,5-dicarbohydrazide (54a) with various benzaldehydes (54b–d) in the presence of catalytic drops of acetic acid without any catalyst and solvent at ambient temperature, which resulted in moderate to higher yields. The majority of the newly synthesized compounds were screened for anticancer activity against the HepG2 cancer cell line, with several showing good IC50 values. Additionally, using Autodock Vina, in silico molecular docking of the synthesized molecules revealed their binding mechanisms inside the active site of DYRK1A (Scheme 55).85
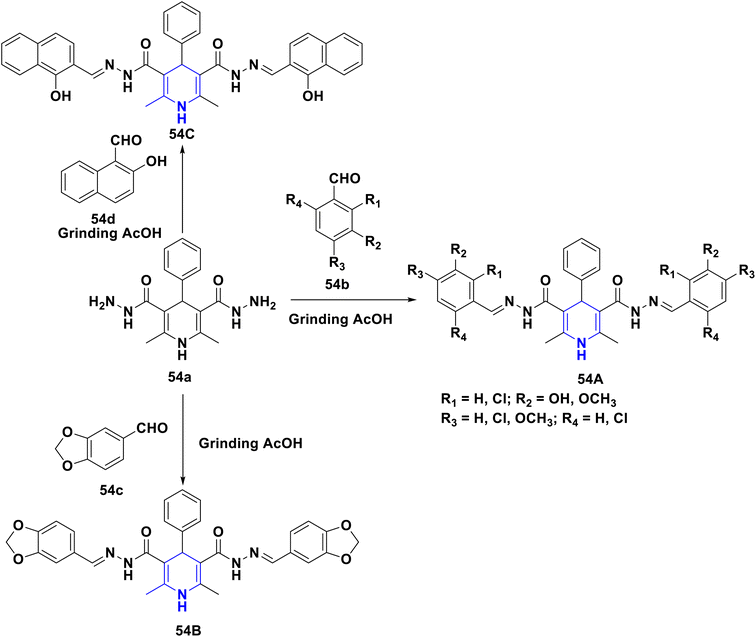 |
| Scheme 55 The 1,4-DHPs-3,5-dicarbohydrazide derivatives as anticancer agents. | |
Sabakhi et al. (2015) conducted research into novel COX-2 inhibitors, a novel series of 1,4-DHPs that were synthesized and studied in vitro and in silico to understand their biological properties. Novel synthesized compounds at the para (p) position of the C-4 phenyl ring with a COX-2 SO2Me pharmacophore, distinct alkoxycarbonyl groups (COOR2) at the C-3 position and hydrophobic groups (R1) at the C-2 position of 1,4-DHP showed specific inhibition of the COX-2 isozyme. Compound 55 exhibited the most powerful and selective COX-2 inhibitor with an IC50 of 0.30 μM and a COX-2 selectivity index of 92. To establish possible binding models for chemical 55, molecular docking research was conducted. The p-SO2Me-phenyl fragment of 55 was found to be placed into the secondary COX-2 binding sites Phe (518), Arg (513), His (90) and Gly (519) in the research study. The SAR discovery shows that compound 55, ethoxycarbonyl and methyl as R1 and COOR2 replacements, has the required molecular geometry as a result of selective inhibition of the COX-2 isozyme, and might be a viable starting point for discovering novel drugs (Scheme 56).86 Denish et al. (2017) have reported on the 1,4-DHP derivatives having the benzylpyridinium moiety 56, and evaluated its anticancer properties using three human cancer lines: lung cancer A549, colorectal adenocarcinoma Caco-2 and glioblastoma U87MG. Among them, compound 56 had strong anticancer properties. The anticancer activity of the synthesized compounds was similar to that of common pharmaceuticals (carboplatin, gemcitabine, and daunorubicin). As a result, 1,4-DHPs that have been synthesized might be regarded as promising compounds for further drug development as anticancer medicines (Scheme 57).87 Sivaramakarthikeyan et al. (2019) reported the synthesis of a series of new phenothiazinyldihydropyridine dicarboxamides 57 using a multi-step synthetic method. The molecules containing o-bromo/fluoro, p-fluoro, o/p-methyl, and m/p-methoxy substituents have anti-inflammatory efficacy that was equivalent to or superior to that of diclofenac sodium. Furthermore, as compared to conventional ascorbic acid, the molecules having o-bromo, m-nitro and fluoro substituents demonstrated higher radical scavenging capabilities. Anticancer research also found that p and m-chloro-derivatives had the highest anticancer efficacy against all pancreatic cancer cells examined. Molecular dynamics studies were conducted on B-cell lymphoma and B-cell lymphoma, and revealed a promising binding affinity in docking (8.10 kcal mol) (Scheme 58).88 Singh et al. (2014) developed and synthesized novel nitrogen mustard pharmacophore hybrids such as DHP-M 58A without a spacer and DHP-L-M 58B with an ethyl spacer. They were evaluated in vitro for their cytotoxicity properties against the COLO 205 (colon), A 549 (lung), IMR-32 (neuroblastoma) and U 87 (glioblastoma) cancer cell lines by MTT assay with chlorambucil and docetaxel standard drugs. Most of the derivatives tested and exhibited cytotoxic activity that ranged from mild to substantial. DHP-M had the greatest anticancer properties in the four cancer cells studied (58A). This might be because 58B provides less steric impediment (Scheme 59).89
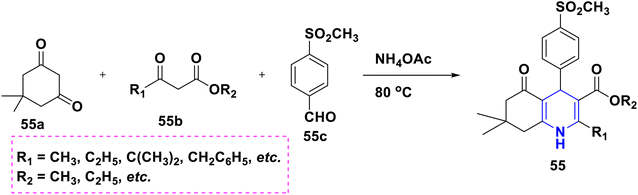 |
| Scheme 56 The sulphoxide 1,4-DHPs as anticancer agents. | |
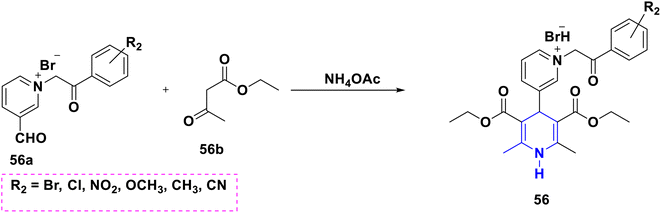 |
| Scheme 57 The benzylpyridinium moiety 1,4-DHPs as antitcancer agents. | |
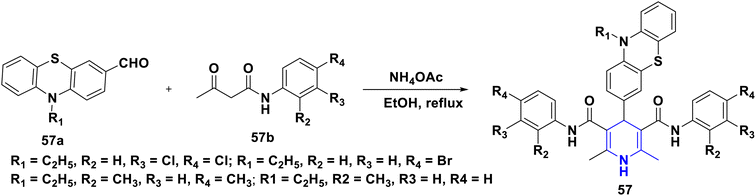 |
| Scheme 58 The phenothiaziny1-1,4-DHPs-dicarboxamides as anticancer agents. | |
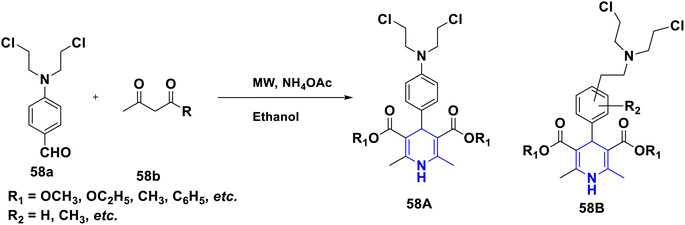 |
| Scheme 59 The notrogen mustard pharmacophore hybride as anticancer agents. | |
The SAR has been constructed, and is visually depicted in Fig. 7 based on the interpretation of the findings. Performing the activity requires the 1,4-DHP central core. It was discovered that the methyl group substitutions at the second and sixth positions were the essential component needed to exert the activity above the other alkyl systems. Considerable functional group variations, such as amide, ester, and acyl hydrazine, have been studied at the third and fifth positions. More activity was elicited by the dicarbohydrazone and amide functional group than the ester functional group. At the fourth position substituted phenyl derivatives, a six-membered aromatic system showed better activity than the six-membered aromatic heterocyclic system, pyridine. Better anticancer activity was demonstrated by compounds having alkoxycarbonyl groups (COOR2) in the third position and a SO2Me and nitrogen mustard pharmacophore at the para position of the phenyl ring, which is in the fourth position.
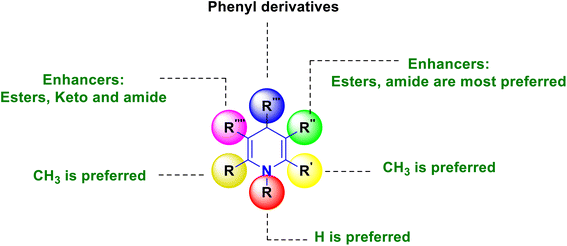 |
| Fig. 7 SAR studies of anti-cancer activity of 1,4-DHPs. | |
2.4.4. Anti-inflammatory molecules. By condensation of compounds 59a with thiosemicarbazide 59b, Idhayadhulla et al. (2015) created a novel family of dihydrazine carbothioamides 59. The synthesized compounds were evaluated for anti-inflammatory effects on Swiss albino rats (Scheme 60).90 Novel derivatives of 4-(3-arylureido)phenyl-1,4-dihydropyridine 60 were synthesized using a straightforward cascade reaction of oxidation, reduction, as well as nucleophilic addition sequences, in moderate to excellent yields. All of the compounds were subjected to anti-inflammatory ability against pro-inflammatory cytokines, such as tumor necrosis factor-alpha (α), TNF-a, and interleukin-6, (IL-6), and also studied anti-microbial activity (anti-bacterial and anti-fungal). Compound 60 exhibited potential anti-inflammatory action, inhibiting TNF-a and IL-6 by 74–83% and 91–96%, correspondingly, with the standard conventional dexamethasone drug (71% and 86%) at a MIC of 10 μM mL−1, respectively. This is the major study on the anti-inflammatory efficacy of new urea containing 1,4-DHP compounds, which are important molecular objectives (Scheme 61).91
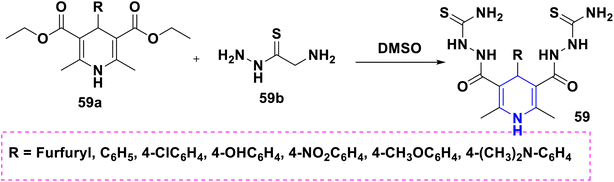 |
| Scheme 60 The dihydrazine carbothioamides 1,4-DHPs as anti-inflammatory agents. | |
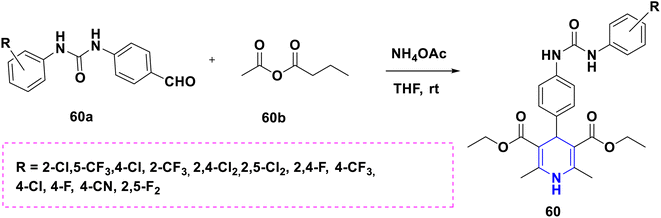 |
| Scheme 61 The 4-(3-arylureido)pheny1-1,4-DHPs urea derivatives as anti-inflammatory agents. | |
The SAR analyses suggest that 1,4-DHP's capacity to reduce inflammation was improved by the addition of dihydrazine carbothioamides or amide groups at the third and fifth positions, as well as 4-(3-arylureido) or amide groups at the fourth position of the phenyl rings (Fig. 8).
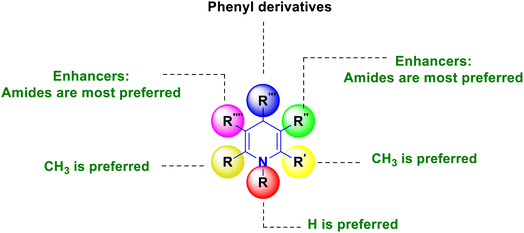 |
| Fig. 8 SAR studies of anti-inflammatory agents of 1,4-DHPs. | |
2.4.5. Anti-microbial agents. Archana et al. (2017) described a novel family of 1,2,3-triazolyl-1,4-dihydropyridine hybrids 61 through the cascade reaction of propargyl salicylaldehyde/o-propargyl naphthaldehyde 61a, β-keto compounds 61b, and organic azides 61c. The newly synthesized hybrids were tested in vitro against four distinct human infections, including Proteus mirabilis, S. aureus, K. pneumonia and E. coli. These outcomes were matched to those of tetracycline, a common antibiotic. These compounds were also tested for their anti-inflammatory effect against bovine serum albumin (BSA). They were then compared to the standard medication diclofenac, and a few hybrids were found to have antibacterial and anti-inflammatory properties that are equivalent to those of reference medicines (Scheme 62).92 Melha et al. (2013) reported that aromatic aldehydes, thiourea, hydroxylamine hydrochloride, and hydrazine hydrate were condensed with 3,5-diacetyl-1,4-dihydro-2,4,6-trimethylpyridine (62a) to generate the corresponding 1,4-DHP derivatives 62A–G. All of the newly synthesized compounds were subjected to their antimicrobial activity, and compounds 62A–C were shown to have stronger action against Gram-positive bacteria than Gram-negative bacteria out of all compounds examined (Scheme 63).93
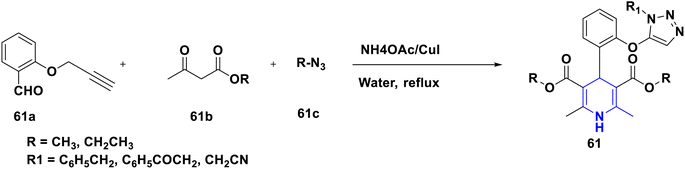 |
| Scheme 62 The 1,2,3-triazoly1-1,4-DHPs hybrids as anti-microbial agents. | |
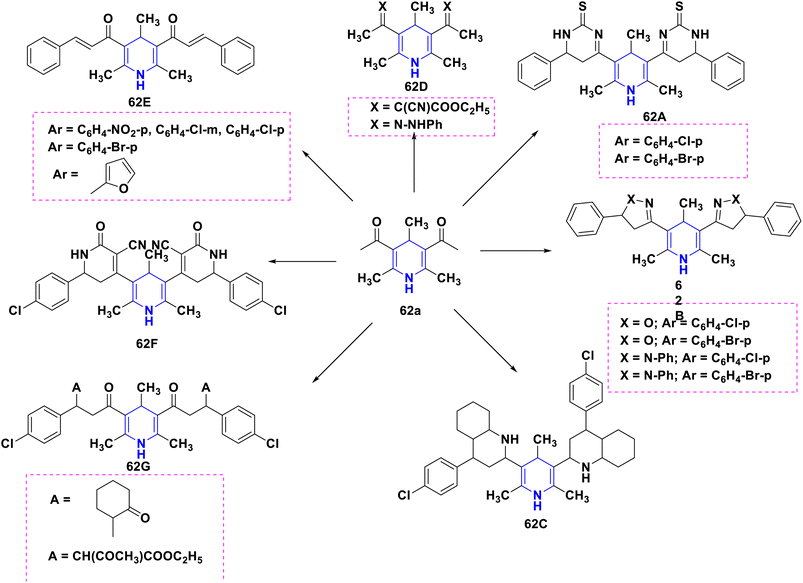 |
| Scheme 63 The 3,5-diacety1-1,4-dihydro-2,4,6-trimethylpyridine as anti-microbial agents. | |
Nkosi et al. (2016) developed a new multi-component method for the synthesis of quinoline-bearing DHP derivatives (63) involving 2-chloro-3-formyl quinolone 63a, malononitrile 63b, arylamines 63c, and dimethyl acetylenedicarboxylate 63d by using Et3N as a base. These molecules were selected for their anticancer activity in an A549 lung cancer cell line, and showed significant anti-proliferative properties. The antibacterial activity of 63 against Pseudomonas aeruginosa ATCC 27853, Escherichia coli ATCC 25922, and Staphylococcus aureus ATCC showed promising activity at the minimum inhibitory concentration (MIC) values of 16 μg mL−1 against Staphylococcus aureus and 32 μg mL−1 against Pseudomonas aeruginosa and Escherichia coli (Scheme 64).94 Lapidot et al. (2015) developed a novel 1,4-DHP 64 as anti-microbial broad-spectrum cationic peptidomimetics. This method involves two steps: the first one is the Hantzsch reaction, which is then followed by the coupling reaction for the synthesis of 1,4-DHP skeleton involving two dipeptides attaching cationic side chains. The synthesized peptidomimetics tested its antibacterial activity, and showed noteworthy MICs in the range of 35–100 μg mL−1 against Gram-(+) and Gram-(−) bacterial pathogens (Scheme 65).95 Narsinghani et al. (2017) synthesized the 1,4-DHP derivative 65, and evaluated their anti-bacterial and anti-fungal properties. They revealed that a bromo group located at the para position of the phenyl ring and a chloro atom attached to the para position of the carbamoyl phenyl ring showed excellent anti-bacterial activities against Gram-negative strains (81.76% and 75.94%, respectively). The hydroxy group at the second position and the fifth position of the bromo group attached to the phenyl moiety of the 1,4-DHP compounds showed good antifungal properties (86.85%). If the chloro group was attached to the para position of the carbamoyl phenyl ring, these molecules exhibited significant antibacterial, as well as antifungal activities. Furthermore, this activity needed electron-withdrawing groups such as fluoro or chloro at the third and fifth positions of the DHP ring (Scheme 66).96 Gomha et al. (2020) reported the reaction of (2,6-dimethyl-4-phenyl-1,4-dihydropyridine-3,5-diyl)-bis(4-amino-4H-1,2,4-triazole-3-thiol) (66a) with several aldehydes in the presence of bromine/acetic acid towards the synthesis of 1,4-DHP hybrid with 1,2,4-triazole 66A and 1,4-dihydropyridine-bis-triazolothiadiazoles hybrid derivatives (66B). The antimicrobial activity of the prepared hybrids was screened and showed excellent activity compared to standards like ampicillin, gentamicin and amphotericin B through the agar diffusion well method (Scheme 67).97
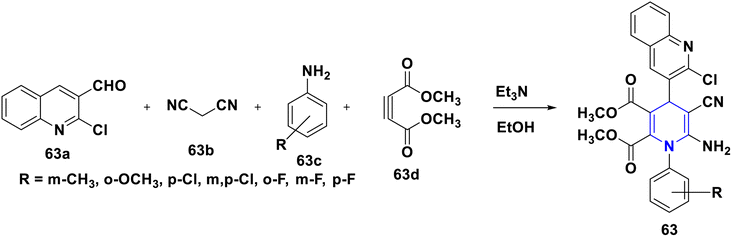 |
| Scheme 64 The quinoline bearing 1,4-DHP derivatives as anti-microbial agents. | |
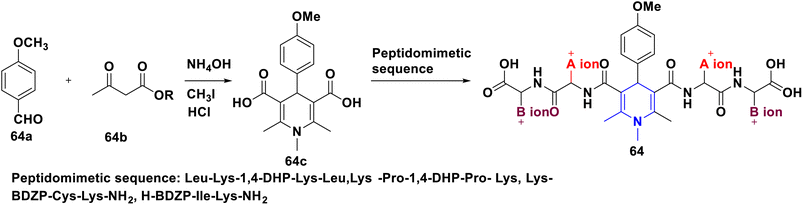 |
| Scheme 65 The cationic peptidomimetics centred on a hydrophobic 1,4-DHP as anti-microbial agents. | |
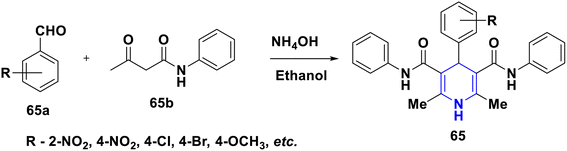 |
| Scheme 66 The carbamoyl 1,4-DHPs as anti-microbial agents. | |
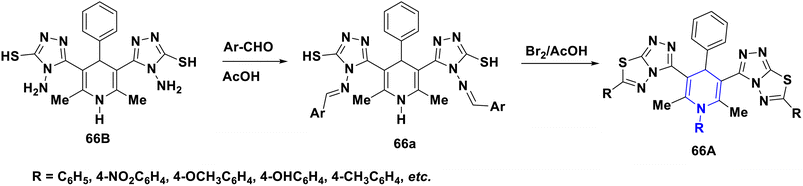 |
| Scheme 67 The triazole 1,4-DHPs as anti-microbial agents. | |
Based on the interpretation of the results, the SAR has been created and is represented visually in Fig. 9. The 1,4-DHP central core is necessary for carrying out the activity. It was found that the methyl group substitutions at the second and sixth positions were crucial for exerting the activity that was superior to other alkyl systems. Numerous functional group changes have been researched at the third and fifth positions, including ester, amide or dipeptides, anhydride, cyano, keto, and five-membered heterocyclics such as triazole-3-thiol. Ester and keto functional groups produced more activity than amide, anhydride, cyano, and five-membered heterocyclics. Therefore, the activity has been improved by the ester and keto functional groups. Six-membered aromatic systems with electron-withdrawing group such as fluoro or chloro at the third and fifth positions, 1,2,3-triazolyl of phenyl ring, 2-chloro quinoline and alkyl derivatives, have demonstrated improved activity at the fourth position.
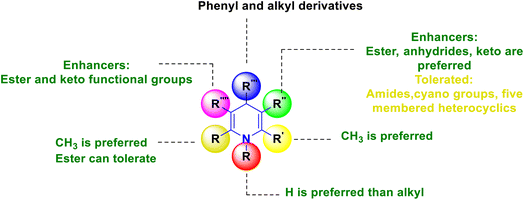 |
| Fig. 9 SAR studies of anti-microbial agents of 1,4-DHPs. | |
2.4.6. Anti-oxidant activity. Sun et al. (2012) reported a reaction of dimethyl or diethyl 2,6-dimethyl-4-phenyl-1,4-DHP-3,5-dicarboxylate 67a. Various chalcone 67b afforded a novel chalcone substituted at the third position of 1,4-DHPs 67. The synthesized molecules were also studied for their radical scavenging activities. Among them, thienyl substituted 1,4-DHPs have shown promising anti-oxidant activities (Scheme 68).98 Kruk et al. (2020) reported the chemiluminescence properties of 4-flavonil-1,4-DHP derivatives 68 by spectrophotometry methods, which showed better light emission from the structure. From this study, all observed luminescence molecules showed superoxide dismutase activity due to the transformation of superoxide radicals (Scheme 69).99 Dhinakaran et al. (2015) reported a cascade method towards the synthesis of N-aryl, 1,4-DHPs 69. They evaluated their cytotoxicity properties using the A549 cell line and antioxidant activities by DPPH methods, and also found better activities (Scheme 70).100 Cabrera et al. (2019) developed the Hantzsch cascade reactions for the synthesis of 1,4-DHPs 70, starting with various aldehydes 70a, β-ketoester 70b and ammonium acetate 70c using a reusable sulfamic acid catalyst. These 1,4-DHP derivatives were studied for their antioxidant activity by using two substituents groups. The results revealed that the nitro group and long chain fatty derivatives embedded with 1,4-DHP scaffolds showed better antioxidant properties, and were almost similar to the standard drug vitamin E (Scheme 71).101
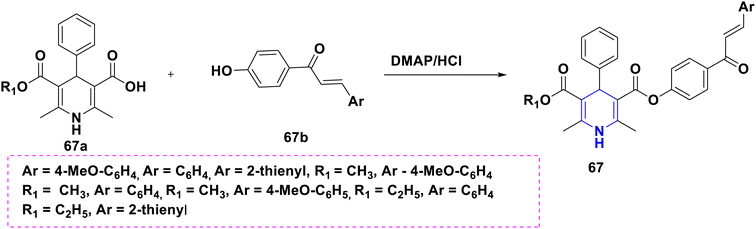 |
| Scheme 68 The chalcone-substituted 1,4-DHPs as anti-oxidant agents. | |
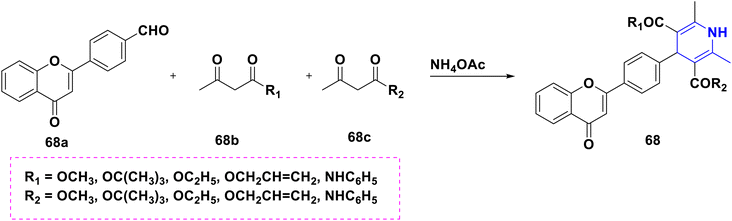 |
| Scheme 69 The flavonil-1,4-DHPs as anti-oxidant agents. | |
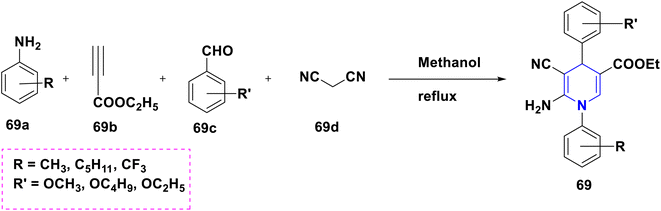 |
| Scheme 70 The N-aryl-1,4-DHPs as anti-oxidant agents. | |
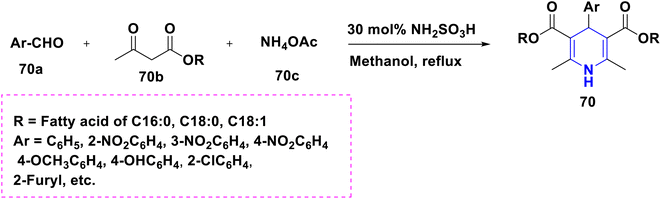 |
| Scheme 71 The long-chain fatty DHPs as anti-oxident agents. | |
Based on the interpretation of the results, the SAR has been created and is represented visually in Fig. 10. The 1,4-DHP central core is necessary for carrying out the activity. It was found that the methyl group substitutions at the second and sixth positions were crucial for exerting the activity that was superior to other alkyl systems. The ester functional group has enhanced activity compared to the cyano functional group at the third position. The chalcone and long chain fatty derivatives embedded at the third and fifth positions show enhanced activity. Phenyl, six-membered aromatic systems have demonstrated improved activity at the fourth position. The 4-flavonil or flavonoids-substituted phenyl system at the fourth position have increased the activity.
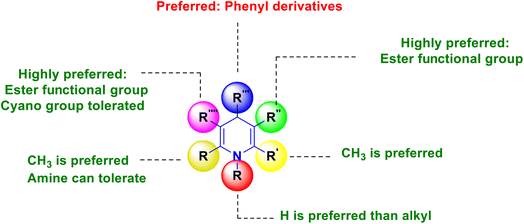 |
| Fig. 10 SAR studies of the antioxidant activity of 1,4-DHPs. | |
2.4.7. Miscellaneous activities. Tenti et al. (2013) discovered the ceric ammonium nitrate-catalyzed one-pot three-component reaction from various α,β-unsaturated ketones 71a, β-dicarbonyl compounds 71b and ammonium acetate towards the synthesis of 4,6-diaryl-1,4-DHPs 71. These compounds were evaluated for their neuroprotective activity. Among them, 1,4-DHP having a 2-thienyl substituent exhibited reasonable neuroprotective properties (Scheme 72).102 Babu et al. (2019) reported the barium nitrate catalyzed reaction of thiadiazolsulfonylamide 72a, different aldehydes 72b using liquid ammonia medium for the preparation of novel thiadiazolsulfonylamide 1,4-DHPs 72, and estimated the anticholinesterase properties by the Ellman method. All of the molecules showed potent inhibition action when compared with the present drug, donepezil HCl, against acetylcholinesterase and butyl cholinesterase enzymes. This outcome indicates the significance of thiadiazolsulfonylamide compounds for anticholinesterase properties, and showed their efficacy and reliability (Scheme 73).103 Kumar et al. (2011) described the condensation reaction of 1,4-DHP ester 73a with thiosemicarbazide 73b towards the synthesis of thiosemicarbazide 1,4-DHPs 73 and studied their anticoagulant properties (Scheme 74).104 Ahamed et al. (2018) developed an ultrasound irradiation method for the synthesis of a novel series of 1,4-DHPs (74A–C) with better yields. The newly synthesized derivatives have been studied for both antimicrobial and anticoagulant properties. Compound 74A (MIC: 0.25 μg mL−1) shows potential active against Escherichia coli, and compound 74B (MIC: 0.5 μg mL−1) also exhibited promising activity against Pseudomonas aeruginosa equal to that of standard ciprofloxacin (MIC: 1 μg mL−1). The antifungal property of 74C (MIC: 0.5 μg mL−1) was more active than that for the clotrimazole (MIC: 1 μg mL−1) standard against Candida albicans. The anticoagulant activity was determined. Compound 74B exhibited good potential in anticoagulant activity, which was almost comparable with the standard of heparin by activated partial thromboplastin time (APTT) and prothrombin time (PT) coagulation assays. The prepared molecule 74C was screened for its anti-cancer properties using HeLa (cervical), HepG2 (liver) and MCF-7 (breast) cancer cell lines, and displayed greater toxicities (GI50 = 0.02 lm). The compound 74B (GI50 = 0.03 lm) also found equipotent activity against the MCF-7 cell line. Henceforth, the molecules (74A–C) might be potent compounds for the development of novel groups of antimicrobial and anticoagulant drugs (Scheme 75).105 E. Preveenkumar et al. (2019) described the MW mediated one-pot reaction using triazole derivatives 75a, β-keto ester 75b and ammonium acetate towards the synthesis of triazole 1,4-DHP derivatives 75 and assessed their anti-diabetic properties. Comparing all derivatives, compound 75 exhibited effective anti-diabetic properties. Hit compounds were additionally confirmed using in vitro enzymatic studies by analysis of its 11-β-hydroxysteroid dehydrogenase-1 (11-β-HSD1) inhibitory property to explain the mechanism of action of these compounds. The outcomes indicated that the 11-β-HSD1 inhibitory property of the molecules was stable and efficient. Molecular docking studies revealed that compound 75 showed a stable binding pattern (−9.758) with human 11-β-HSD1 (Scheme 76).106
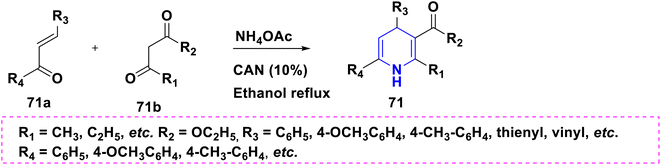 |
| Scheme 72 The 4,6-diaryl-1,4-DHPs as neuroprotective agents. | |
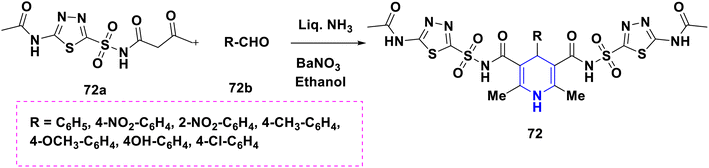 |
| Scheme 73 The thiadiazol sulfonyl anamide 1,4-DHPs as anticholinesterase agents. | |
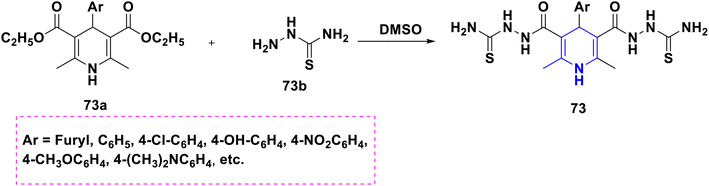 |
| Scheme 74 The thiosemicarbazide 1,4-DHPs as anticoagulant agents. | |
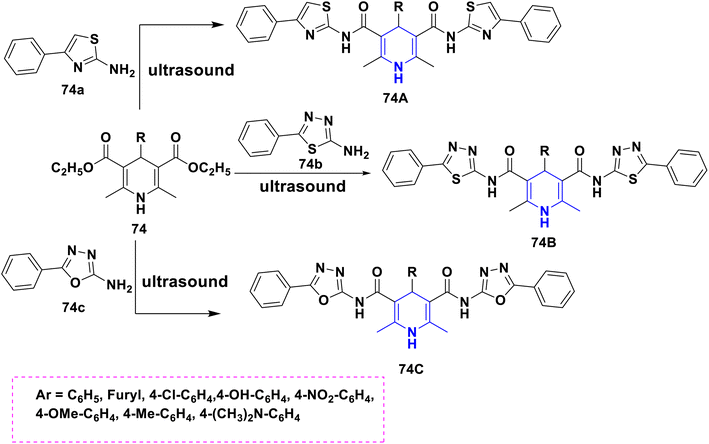 |
| Scheme 75 The phenylthiadiazol-2amine 1,4-DHPs as anticoagulant agents. | |
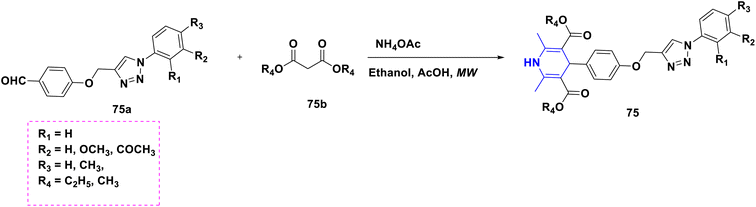 |
| Scheme 76 The triazol 1,4-DHP derivatives as antidiabetic agents. | |
2.5. Agrochemical applications
2.5.1. Insecticidal activity. Sun et al. (2012) synthesized 1,4-DHP and cis-nitenpyram molecular hybrids 76, and examined the impact of different functional group variations on the insecticidal activity. The functional group variations include the nitro group at the fifth position, CN or ester groups at the third position, various substituted aryl systems such as F, Cl, Br, and OCH3 at the fourth position and cis-nitenpyram 76a at the sixth position. The synthesis uses cis-nitenpyram 76a, where the secondary amine and nitro group are sealed in a cis-configuration, and aldehydes 76b and malononitrile 76c serve as reactants in the piperidine-catalyzed, one-pot synthesis. The insecticidal activity of cis-configured 1,4-DHPs against the most frequent pest, Aphis medicaginis, was enhanced (Scheme 77).107 In a related study, Rao and Parthiban (2014) successfully synthesized the cis-nitenpyram neonicotinoid insecticide analogue 77 by substituting 1-(6-chloropyridin-3-yl)-N-methylmethanamine 77b on 77a with good yield. The NO2 group at the neonicotinoid's fifth position is crucial for enhancing the insecticidal efficacy, according to the literature. In this work, NO2 groups were linked to 1,4-DHPs at the third and fifth locations in the hopes that this would increase their insecticidal activity.63 The effect of temperature on the product is another intriguing discovery of this study. The ArCH2NMe and NO2 groups are in steric hindrance at room temperature, and tend to rotate slowly around the C2–N bond. When the temperature was increased to 80 °C, the spectrum became clear with attaining a particular orientation without steric hindrance (Scheme 78). The synthesis and insecticidal activities of 1,4-DHPs and cis-nitenpyram molecular hybrids have been carried out by Liu et al. (2012). The MW reactor-assisted, piperidine-catalyzed one pot MCR synthesis was achieved by using methyl cyanoacetate 78a, benzaldehyde, 78b and nitenpyram, 78c. The compound 78 has exhibited insecticidal activity at 500 mg L−1 against Aphis craccivora (Scheme 79).108 In addition, Lu et al. (2012) explored the molecular hybrids of 1,4-DHPs and nitenpyram, and the molecular hybrids of 1,4-DHPs and clothianidin. Among these two, clothianidin nitromethylene neonicotinoids showed good insecticidal properties against cowpea aphid (Aphis craccivora) and armyworm (Pseudaletia separata ‘Walker’) (Scheme 80).109
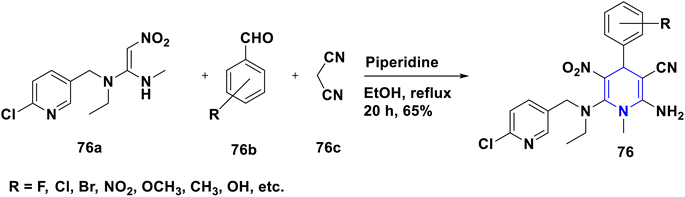 |
| Scheme 77 cis-Nitenpyram analogues containing 1,4-DHPs. | |
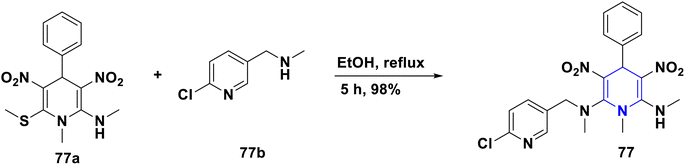 |
| Scheme 78 Synthesis of neonicotinied insecticide analogue. | |
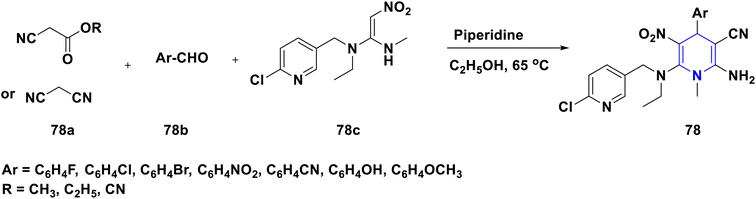 |
| Scheme 79 Synthesis of 1,4-DHPs and cis-nitenpyram hybrid analogues. | |
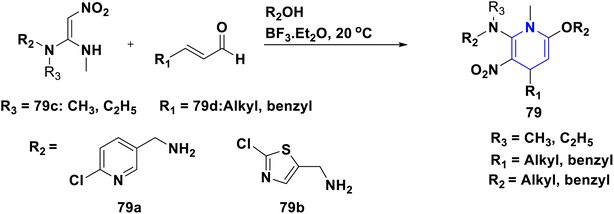 |
| Scheme 80 Synthesis and insecticidal activities. | |
A novel series of nithiazine-containing 1,4-DHP analogues 80 was designed and synthesized by Tian et al. (2014). The MCR reaction involves nithiazine 80a, malononitrile or ethyl cyanoacetate 80b, and benzaldehyde 80c as reactants. The synthesized compounds have been screened for insecticidal activity, and showed moderate activity against Aphis craccivora. When compared with the ester group, the CN at the fifth position of 1,4-DHP had greater insecticidal activity owing to the stronger electron-withdrawing nature of the substituents (Scheme 81).110 The works of Bakhite et al. (2014) designed, synthesized and identified the insecticidal activities of novel piperidinium salts of novel 1,4-DHPs 81. In their bioassay studies, the compounds showed moderate to strong aphidicidal activities. Interestingly, one of the compounds 81 showed 4-fold higher insecticidal activity than acetamiprid because of the nitro group present at the fourth position aryl of the 1,4-DHP (Scheme 82).111 Yang et al. (2018) created a new set of 1,4-DHPs 82A–C, and tested their insecticidal effectiveness against M. persicae, B. brassicae, T. cinnabarinus, B. cinerea, M. oryzae, S. sclerotiorum, and F. oxysporum. The LC50 values were 0.011, 0.0015, and 0.0007 μM. The most active compound, 82B, showed greater insecticidal efficacy against B. brassicae, M. persicae, and T. cinnabarinus, respectively. At 50 mg L−1, compound 82A inhibited F. oxysporum and S. sclerotiorum by 45% and 65.83%, respectively. They determined through SAR experiments that functional group differences in 1,4-DHP have a significant influence on insecticidal activity (Scheme 83).112 He et al. (2013) developed the synthesis of neonicotinoid and 1,4-DHP hybrids 83, as well as their insecticidal and antibacterial activities. At 500 mg L−1, compound 83 has insecticidal activity against N. lugens, and at 200 mg L−1, they have antibacterial activity against Pseudomonas solanacearum (Scheme 84).113
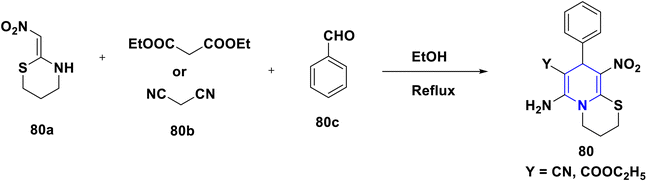 |
| Scheme 81 Synthesis of insecticides. | |
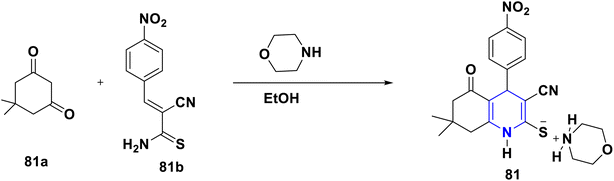 |
| Scheme 82 Synthesis of insecticides. | |
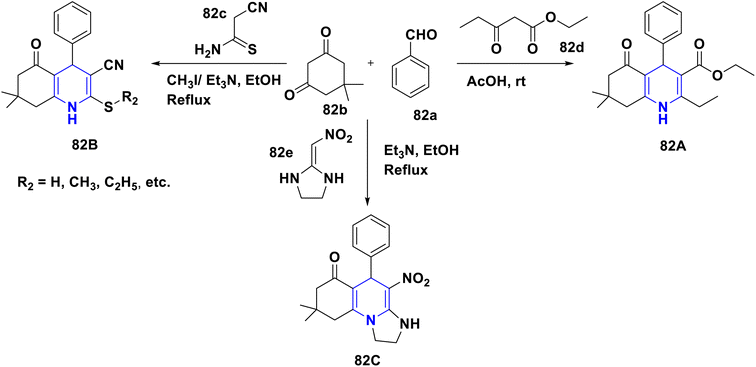 |
| Scheme 83 Synthesis of insecticides. | |
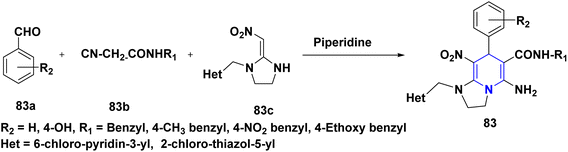 |
| Scheme 84 Synthesis of insecticidal neonicotinoid and 1,4-DHPs hybrids. | |
The enhanced π–π stacking-based neonicotinoid 1,4-DHPs 84 have been designed and synthesized by Zhang et al. (2010). From the biological assays, these compounds were found to possess significant insecticidal properties, especially against pea aphid, Aphis craccivora (Scheme 85).114 In their 2015 study, Tian et al. (2015) described the design and multicomponent reactions of nitenpyram 85a, triethoxymethane 85b, and 4,4,4-trifluoro-3-oxobutaneanilides 85c under solvent-free and catalyst-free conditions that produced a series of novel neonicotinoids with a dihydro-pyridine ring and a trifluoromethyl group 85 (Scheme 86). As an electron-withdrawing group, the trifluoromethyl group at the second position of 1,4-DHPs was crucial in increasing the reaction activity of the molecule. According to bioassays, the majority of the synthetic compounds had mild insecticidal effects on Aphis craccivora.115 According to Sun et al. (2011) nitenpyram 86a was modified by adding various straight chain amino acid alkyl esters 86b to create several unique nitenpyram analogues 86. By varying the size of the ester group and the length of the flexible ester arm, the analogues displayed diverse insecticide activity in a more manageable and sensible manner (Scheme 87). The test analogues all showed effective insecticidal action at 100 mg L−1. However, analogues 4c and 4d had the best in vitro activity and 100% mortality at 20 mg L−1.116
 |
| Scheme 85 Synthesis of insecticides. | |
 |
| Scheme 86 Synthesis of neonicotinoids insecticides with trifluoromethyl group. | |
 |
| Scheme 87 Synthesis of neonicotinoids insecticides with ester group. | |
Based on the interpretation of the results, the SAR has been created and is represented visually in Fig. 11. The 1,4-DHP central core is necessary for carrying out the activity. The replacement of hydrogen at the first position –NH– with a methyl group has shown better activity, but extending the alkyl chain beyond the propyl system reduced the activity. It was found that the amine and trifluoromethyl group enhanced the activity more than the thio group at the second position. Numerous functional group changes have been researched at the third and fifth positions. It was found that the nitro and cyano groups have enhanced the activity due to stronger electron-withdrawing activity and smaller volume than the ester and amide. Methyl ester has demonstrated greater activity among the esters than the ethyl ester group. Better activity has been seen for phenyl and substituted 4-Cl, 2-F, and electron-donating groups such OCH3, OH, and CH3 on phenyl systems at the fourth position. The important element for exerting the insecticidal activity was discovered to be the substitutions at the sixth position. The alkyl amine substituted with 6-chloro-pyridin-3-yl and 5-chloro-pyridin-3-yl, respectively, six-membered and five hetero aromatic systems, have shown enhanced activity at the sixth position. The activity has also been improved by substituting methyl and ethyl groups for hydrogen in the alkyl amine (–NH–) system. At the sixth position, clothianidin nitromethylene neonicotinoids are better than their nitenpyram counterparts in terms of insecticidal activity.
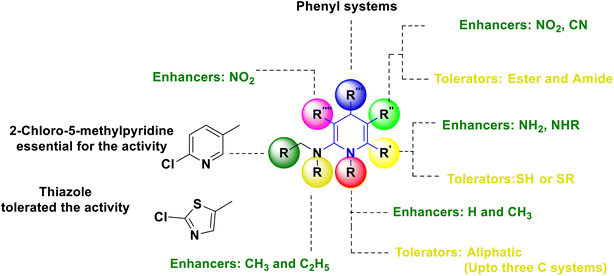 |
| Fig. 11 SAR studies of insecticidal activities. | |
3. Conclusion
The current study concludes that 1,4-DHPs constitute a pharmacologically significant class of compounds. We have systematically described the work done on 1,4-DHP compounds with synthetic methodology developments, biological applications as an agrochemical, and pharmacological activities such as antihypertensive, anticancer, calcium channel blockers, anti-inflammatory, anti-microbial, anti-fungal, anti-oxidant, anti-cholinesterase, anti-coagulant, neuro-protective, and anti-diabetic activities in this study. However, there are limited publications and no review on the agrochemical applications of 1,4-DHPs. As a result, medicinal chemists are still exploring new synthesis techniques to generate new neonicotinoid-embedded 1,4-DHPs with insecticidal action. This review covers the insecticidal properties of 1,4-DHPs and cis-nitenpyram neonicotinoid molecular hybrids. These neonicotinoid 1,4-DHP compounds could be a potential source of chemicals for the pharmaceutical industry. The biological functions and SAR analysis of the compounds were presented in this review to stress their medicinal possibilities for future medicinal chemistry developments. This could be useful and enlightening for academic and industrial researchers working in the fields of 1,4-DHP base medicinal and pharmaceutical chemistry.
Conflicts of interest
The authors declare that there are no conflicts of interest.
Acknowledgements
We thank the Director of the National Institute of Pharmaceutical Education and Research Guwahati for excellent support. This work was supported by the GMP Project, establishment of the GMP-accredited pilot scale extraction facilities for the development of herbal or phytopharmaceutical products from the Medicinal Plants of NE India, sponsored by DBT, Government of India.
References
- C. Maria Assunta, I. Daniela, R. Roberto, V. G. Salvatore and L. Laura, Curr. Med. Chem., 2019, 26, 7166–7195 Search PubMed.
- E. Khan, ChemistrySelect, 2021, 6, 3041–3064 CrossRef CAS.
- U. Eisner and J. Kuthan, Chem. Rev., 1972, 72, 1–42 CrossRef CAS.
- J. P. Wan and Y. Pan, Mini-Rev. Med. Chem., 2012, 12, 337–349 CrossRef CAS PubMed.
- R. Mathur, K. S. Negi, R. Shrivastava and R. Nair, RSC Adv., 2021, 11, 1376–1393 RSC.
- M. Żorniak, K. Mitręga and T. F. Krzemiński, Kardiol. Pol., 2011, 3, 100–103 Search PubMed.
- S. Sepehri, H. P. Sanchez and A. Fassihi, J. Pharm. Pharm. Sci., 2015, 18, 1–52 CAS.
- A. K. Samrat and B. A. Pratibha, Mini-Rev. Med. Chem., 2014, 14, 282–290 CrossRef.
- N. Edraki, A. R. Mehdipour, M. Khoshneviszadeh and R. Miri, Drug Discovery Today, 2009, 14, 1058–1066 CrossRef CAS PubMed.
- F. Bossert and W. Vater, Med. Res. Rev., 1989, 9, 291–324 CrossRef CAS PubMed.
- D. Maclean, J. J. Baldwin, V. T. Ivanov, Y. Kato, A. Shaw, P. Schneider and E. M. Gordon, J. Comb. Chem., 2000, 2, 562–578 CrossRef CAS PubMed.
- M. Abhinav Prasoon, B. Ankit and R. Awani Kumar, Mini-Rev. Med. Chem., 2019, 19, 1219–1254 CrossRef PubMed.
- J. T. David, Mini-Rev. Med. Chem., 2003, 3, 215–223 CrossRef PubMed.
- R. A. Batey, J. Am. Chem. Soc., 2007, 129, 7476 CrossRef CAS.
- L. F. Tietze and N. Rackelmann, Pure Appl. Chem., 2004, 76, 1967–1983 CrossRef CAS.
- M. H. Shih, Y. S. Su and C. L. Wu, Chem. Pharm. Bull., 2007, 55, 1126–1135 CrossRef CAS PubMed.
- A. Dömling and I. Ugi, Angew. Chem., Int. Ed., 2000, 39, 3168–3210 CrossRef.
- A. Dömling, Chem. Rev., 2006, 106, 17–89 CrossRef PubMed.
- C. Oliver Kappe, Tetrahedron, 1993, 49, 6937–6963 CrossRef.
- M. Han, S.-q. Zhang, X. Cui, Q.-w. Wang, G.-x. Li and Z. Tang, Angew. Chem., Int. Ed., 2022, 61, e202201418 CAS.
- L. O'Brien, S. P. Argent, K. Ermanis and H. W. Lam, Angew. Chem., Int. Ed., 2022, 61, e202202305 CrossRef PubMed.
- L. Xue, L. Wu, Y. Li, Q. Yang, D. Sun, H. Zhang, H. Xu and Y. Li, Luminescence, 2022, 37, 177–185 CrossRef CAS PubMed.
- I. C. Schiller, K. A. Jacobson, Z. Wen, A. Malisetty, G. Schmalzing and F. Markwardt, Molecules, 2022, 27, 1846 CrossRef CAS PubMed.
- S. Bijani, D. Iqbal, S. Mirza, V. Jain, S. Jahan, M. Alsaweed, Y. Madkhali, S. A. Alsagaby, S. Banawas, A. Algarni, F. Alrumaihi, R. M. Rawal, W. Alturaiki and A. Shah, Life, 2022, 12, 519 CrossRef CAS PubMed.
- D. S. Malhi, H. S. Sohal, K. Singh, Z. M. Almarhoon, A. B. Bacha and M. I. Al-Zaben, ACS Omega, 2022, 7, 16055–16062 CrossRef CAS PubMed.
- H. Döring, D. Kreutzer, C. Ritter and A. Hilgeroth, Molecules, 2021, 26, 3575 CrossRef PubMed.
- A. O. Kurskova, V. V. Dotsenko, K. A. Frolov, N. A. Aksenov, I. V. Aksenova, B. S. Krivokolysko, A. A. Peresypkina, E. A. Chigorina and S. G. Krivokolysko, Russ. J. Gen. Chem., 2022, 92, 779–790 CrossRef CAS PubMed.
- S. Moradi, M. Moradian and H. Naeimi, Acta Chim. Slov., 2022, 69, 349–358 CrossRef CAS PubMed.
- K. Patra, M. K. Reddy, S. Mallik and M. Baidya, Org. Lett., 2022, 24, 4014–4018 CrossRef CAS PubMed.
- P. Li, S. Wang, N. Tian, H. Yan, J. Wang and X. Song, Org. Biomol. Chem., 2021, 19, 3882–3892 RSC.
- M. Erşatır, M. Türk and E. S. Giray, J. Supercrit. Fluids, 2021, 176, 105303 CrossRef.
- N. Kerru, S. Maddila and S. B. Jonnalagadda, Front. Chem., 2021, 9, 638832 CrossRef CAS PubMed.
- S. Ghosh, F. Saikh, J. Das and A. K. Pramanik, Tetrahedron Lett., 2013, 54, 58–62 CrossRef CAS.
- N. Koukabi, E. Kolvari, A. Khazaei, M. A. Zolfigol, B. Shirmardi-Shaghasemi and H. R. Khavasi, Chem. Commun., 2011, 47, 9230–9232 RSC.
- K. L. Bridgwood, G. E. Veitch and S. V. Ley, Org. Lett., 2008, 10, 3627–3629 CrossRef CAS PubMed.
- R. Kumar, N. H. Andhare, A. Shard, Richa and A. K. Sinha, RSC Adv., 2014, 4, 19111–19121 RSC.
- C. G. Evans, U. K. Jinwal, L. N. Makley, C. A. Dickey and J. E. Gestwicki, Chem. Commun., 2011, 47, 529–531 RSC.
- J. Safari, S. H. Banitaba and S. Dehghan Khalili, Chin. J. Catal., 2011, 32, 1850–1855 CrossRef CAS.
- H. Jiang, X. Ji, Y. Li, Z. Chen and A. Wang, Org. Biomol. Chem., 2011, 9, 5358–5361 RSC.
- G.-W. Wang and C.-B. Miao, Green Chem., 2006, 8, 1080–1085 RSC.
- S. Sueki, R. Takei, Y. Zaitsu, J. Abe, A. Fukuda, K. Seto, Y. Furukawa and I. Shimizu, Eur. J. Org. Chem., 2014, 2014, 5281–5301 CrossRef CAS.
- J. Sun, E.-Y. Xia, Q. Wu and C.-G. Yan, Org. Lett., 2010, 12, 3678–3681 CrossRef CAS PubMed.
- J. Yang, C. Wang, X. Xie, H. Li and Y. Li, Org. Lett., 2010, 2010, 4189–4193 Search PubMed.
- C. Altug, A. K. Burnett, E. Caner, Y. Dürüst, M. C. Elliott, R. P. J. Glanville, C. Guy and A. D. Westwell, Tetrahedron, 2011, 67, 9522–9528 CrossRef CAS.
- M. Li, H. Cao, Y. Wang, X.-L. Lv and L.-R. Wen, Org. Lett., 2012, 14, 3470–3473 CrossRef CAS PubMed.
- Y. Nakaike, N. Nishiwaki, M. Ariga and Y. Tobe, J. Org. Chem., 2014, 79, 2163–2169 CrossRef CAS PubMed.
- Z. Arzehgar, S. Sajjadifar and H. Arandiyan, Asian J. Green Chem., 2019, 3, 43–52 Search PubMed.
- J. Davarpanah, M. Ghahremani and O. Najafi, J. Mol. Struct., 2019, 1177, 525–535 CrossRef CAS.
- M. G. Dekamin, S. Ilkhanizadeh, Z. Latifidoost, H. Daemi, Z. Karimi and M. Barikani, RSC Adv., 2014, 4, 56658–56664 RSC.
- J. Zhu, Eur. J. Org. Chem., 2003, 2003, 1133–1144 CrossRef.
- N. Devarajan and P. Suresh, New J. Chem., 2019, 43, 6806–6814 RSC.
- M. S. Maru, P. K. Sudhadevi Antharjanam and N.-u. H. Khan, ChemistrySelect, 2019, 4, 774–782 CrossRef CAS.
- R. Mahinpour, L. Moradi, Z. Zahraei and N. Pahlevanzadeh, J. Saudi Chem. Soc., 2018, 22, 876–885 CrossRef CAS.
- M. G. Sharma, D. P. Rajani and H. M. Patel, R. Soc. Open Sci., 2017, 4, 170006 CrossRef CAS PubMed.
- G. B. Dharma Rao, J. Heterocycl. Chem., 2018, 55, 2556–2562 CrossRef CAS.
- D. Hu, Y. Liu and J.-P. Wan, Tetrahedron, 2015, 71, 6094–6098 CrossRef CAS.
- J. Wan, Y. Zhou, Y. Liu, Z. Fang and C. Wen, Chin. J. Chem., 2014, 32, 219–226 CrossRef CAS.
- Z.-Q. Zhu, Z.-B. Xie and Z.-G. Le, J. Org. Chem., 2016, 81, 9449–9454 CrossRef CAS PubMed.
- S. E. Kiruthika and P. T. Perumal, RSC Adv., 2014, 4, 3758–3767 RSC.
- F.-J. Liu, T.-T. Sun, Y.-G. Yang, C. Huang and X.-B. Chen, RSC Adv., 2018, 8, 12635–12640 RSC.
- C.-S. Yao, C.-H. Wang, B. Jiang and S.-J. Tu, J. Comb. Chem., 2010, 12, 472–475 CrossRef CAS PubMed.
- M. Li, K.-N. Sun and L.-R. Wen, RSC Adv., 2016, 6, 21535–21539 RSC.
- H. Surya Prakash Rao and A. Parthiban, Org. Biomol. Chem., 2014, 12, 6223–6238 RSC.
- Y. Sambongi, H. Nitta, K. Ichihashi, M. Futai and I. Ueda, J. Org. Chem., 2002, 67, 3499–3501 CrossRef CAS PubMed.
- T. Itoh, K. Nagata, A. Kurihara, M. Miyazaki and A. Ohsawa, Tetrahedron Lett., 2002, 43, 3105–3108 CrossRef CAS.
- A. Boumendjel, H. Baubichon-Cortay, D. Trompier, T. Perrotton and A. Di Pietro, Med. Res. Rev., 2005, 25, 453–472 CrossRef CAS PubMed.
- G. Prasanthi, K. V. S. R. G. Prasad and K. Bharathi, Eur. J. Med. Chem., 2014, 73, 97–104 CrossRef CAS PubMed.
- C. G. Evans and J. E. Gestwicki, Org. Lett., 2014, 16, 6040 CrossRef CAS PubMed.
- T. D. Ananda Kumar, P. Mohan, C. V. S. Subrahmanyam and K. Satyanarayana, Synth. Commun., 2014, 44, 574–582 CrossRef.
- Z. Zarnegar, J. Safari and Z. M. Kafroudi, New J. Chem., 2015, 39, 1445–1451 RSC.
- J.-P. Wan and Y. Liu, RSC Adv., 2012, 2, 9763–9777 RSC.
- S. S. Kahandal, S. R. Kale, M. B. Gawande and R. V. Jayaram, Catal. Sci. Technol., 2014, 4, 672–680 RSC.
- T. Ogawa, A. Nakazato, K. Tsuchida and K. Hatayama, Chem. Pharm. Bull., 1993, 41, 1049–1054 CrossRef CAS PubMed.
- A. A. Nekooeian, A. Khalili, K. Javidnia, A. R. Mehdipour and R. Miri, Iran. J. Pharm. Sci., 2010, 8, 193–199 Search PubMed.
- F. Hadizadeh, Z. Fatehi-Hassanabad, M. Fatehi-Hassanabad, A. Beheshtizadeh and F. Nabati, Results Pharma Sci., 2009, 2, 85–90 Search PubMed.
- A. Ashimori, T. Ono, Y. Inoue, S. Morimoto, M. Eda, T. Uchida, Y. Ohtaki, Y. Fujino, H. Kido, Y. Ogura, C. Fukaya, M. Watanabe and K. Yokoyama, Chem. Pharm. Bull., 1991, 39, 91–99 CrossRef CAS PubMed.
- H. Kanno, H. Yamaguchi, Y. Okamiya, K. Sunakawa, T. Takeshita and T. Naruchi, Chem. Pharm. Bull., 1992, 40, 2049–2054 CrossRef CAS PubMed.
- A. Fassihi, H. Sadeghi, A. Zarghi and A. Shafiee, J. Res. Med. Sci., 2004, 1, 5–10 Search PubMed.
- E. K. Ozer, M. G. Gunduz, A. El-Khouly, Y. Sara, R. Simsek, A. B. Iskit and C. Safak, Turk. J. Biochem., 2018, 43, 578–586 CrossRef CAS.
- C. Bladen, M. G. Gündüz, R. Şimşek, C. Şafak and G. W. Zamponi, Pfluegers Arch., 2014, 466, 1355–1363 CrossRef CAS PubMed.
- T. Yamamoto, S. Niwa, S. Ohno, T. Onishi, H. Matsueda, H. Koganei, H. Uneyama, S.-i. Fujita, T. Takeda, M. Kito, Y. Ono, Y. Saitou, A. Takahara, S. Iwata and M. Shoji, Bioorg. Med. Chem. Lett., 2006, 16, 798–802 CrossRef CAS PubMed.
- R. Miri, K. Javidnia, H. Sarkarzadeh and B. Hemmateenejad, Bioorg. Med. Chem., 2006, 14, 4842–4849 CrossRef CAS PubMed.
- M. Rucins, D. Kaldre, K. Pajuste, M. A. S. Fernandes, J. A. F. Vicente, L. Klimaviciusa, E. Jaschenko, I. Kanepe-Lapsa, I. Shestakova, M. Plotniece, M. Gosteva, A. Sobolev, B. Jansone, R. Muceniece, V. Klusa and A. Plotniece, C. R. Chim., 2014, 17, 69–80 CrossRef CAS.
- P. Anaikutti and P. Makam, Bioorg. Chem., 2020, 105, 104379 CrossRef CAS PubMed.
- S. M. Gomha, Z. A. Muhammad, H. M. Abdel-aziz, I. K. Matar and A. A. El-Sayed, Green Chem. Lett. Rev., 2020, 13, 6–17 CrossRef CAS.
- I. Sabakhi, V. Topuzyan, Z. Hajimahdi, B. Daraei, H. Arefi and A. Zarghi, Iran. J. Pharm. Sci., 2015, 14, 1087–1093 CAS.
- V. Denish, M. Sheefa, S. Faraz, K. Rajesh, R. Anand, J. Nayan, R. Rakesh and S. Anamik, Anti-Cancer Agents Med. Chem., 2017, 17, 1003–1013 Search PubMed.
- R. Sivaramakarthikeyan, S. Iniyaval, K. Padmavathy, H.-S. Liew, C.-K. Looi, C.-W. Mai and C. Ramalingan, New J. Chem., 2019, 43, 17046–17057 RSC.
- R. K. Singh, D. N. Prasad and T. R. Bhardwaj, Med. Chem. Res., 2015, 24, 1534–1545 CrossRef CAS.
- A. Idhayadhulla, R. S. Kumar, A. J. A. Nasser, S. Kavimani and S. Indhumathy, Pharm. Chem. J., 2015, 49, 463–466 CrossRef CAS.
- R. H. Tale, A. H. Rodge, G. D. Hatnapure, A. P. Keche, K. M. Patil and R. P. Pawar, Med. Chem. Res., 2013, 22, 1450–1455 CrossRef CAS.
- S. Archana, M. Dinesh, R. Ranganathan, A. Ponnuswamy, P. Kalaiselvi, S. Chellammal, G. Subramanian and S. Murugavel, Res. Chem. Intermed., 2017, 43, 187–202 CrossRef CAS.
- H. Abu-Melha, Spectrochim. Acta, Part A, 2013, 113, 115–122 CrossRef CAS PubMed.
- S. b. M. v. Nk–osi, K. Anand, S. Anandakumar, S. Singh, A. A. Chuturgoon and R. M. Gengan, J. Photochem. Photobiol., B, 2016, 165, 266–276 CrossRef PubMed.
- I. Lapidot, A. Albeck, G. Gellerman, S. Shatzmiller and F. Grynszpan, Int. J. Pept. Res. Ther., 2015, 21, 243–247 CrossRef CAS.
- T. Narsinghani, L. Soni and S. Chourey, J. Drug Delivery Ther., 2017, 7, 142–145 CAS.
- S. M. Gomha, M. M. Edrees, Z. A. Muhammad, N. A. Kheder, S. Abu- Melha and A. M. Saad, Polycyclic Aromat. Compd., 2020, 1–13, DOI:10.1080/10406638.2020.1720751.
- H. Sun, C. Shang, L. Jin and J. Zhang, Heterocycl. Commun., 2012, 18, 239–243 CrossRef CAS.
- I. Kruk, A. Kladna, K. Lichszteld, T. Michalska, H. Y. Aboul-Enein, M. Tunçbilek and R. Ertan, Biopolymers, 2001, 62, 163–167 CrossRef CAS PubMed.
- I. Dhinakaran, V. Padmini and N. Bhuvanesh, J. Chem. Sci., 2015, 127, 2201–2209 CrossRef CAS.
- D. da Costa Cabrera, E. Santa-Helena, H. P. Leal, R. R. de Moura, L. E. M. Nery, C. A. N. Gonçalves, D. Russowsky and M. G. Montes D'Oca, Bioorg. Chem., 2019, 84, 1–16 CrossRef CAS PubMed.
- G. Tenti, J. Egea, M. Villarroya, R. León, J. C. Fernández, J. F. Padín, V. Sridharan, M. T. Ramos and J. C. Menéndez, MedChemComm, 2013, 4, 590–594 RSC.
- M. Niranjan Babu, K. Elumalai, S. Srinivasan, K. Eluri, M. Elumalai and S. Sivannan, Carbon Resour. Convers., 2019, 2, 191–197 CrossRef CAS.
- R. S. Kumar, A. Idhayadhulla, A. J. Abdul Nasser and J. Selvin, Eur. J. Med. Chem., 2011, 46, 804–810 CrossRef CAS PubMed.
- A. Ahamed, I. A. Arif, M. Mateen, R. Surendra Kumar and A. Idhayadhulla, Saudi J. Biol. Sci., 2018, 25, 1227–1235 CrossRef CAS PubMed.
- E. Praveenkumar, N. Gurrapu, P. Kumar Kolluri, V. Yerragunta, B. Reddy Kunduru and N. J. P. Subhashini, Bioorg. Chem., 2019, 90, 103056 CrossRef CAS PubMed.
- C. Sun, Y. Chen, T. Liu, Y. Wu, T. Fang, J. Wang and J. Xing, Chin. J. Chem., 2012, 30, 1415–1422 CrossRef CAS.
- T.-Y. Liu, S.-J. Xue, Y.-X. Chen, T. Fang, Y. Wu and J. Wang, J. Heterocycl. Chem., 2012, 49, 1376–1379 CrossRef CAS.
- X. Shao, Z. Li, X. Qian and X. Xu, J. Agric. Food Chem., 2009, 57, 951–957 CrossRef CAS PubMed.
- Z. Tian, S. Cui and Z. Xu, Res. Chem. Intermed., 2014, 40, 1053–1059 CrossRef CAS.
- E. A. Bakhite, A. A. Abd-Ella, M. E. A. El-Sayed and S. A. A. Abdel-Raheem, J. Agric. Food Chem., 2014, 62, 9982–9986 CrossRef CAS PubMed.
- G.-Z. Yang, X.-F. Shang, P.-L. Cheng, X.-D. Yin, J.-K. Zhu, Y.-Q. Liu, J. Zhang and Z.-J. Zhang, Molecules, 2018, 23, 2422 CrossRef PubMed.
- Y. He, D. Hu, M. Lv, L. Jin, J. Wu, S. Zeng, S. Yang and B. Song, Chem. Cent. J., 2013, 7, 76 CrossRef PubMed.
- W. Zhang, X. Yang, W. Chen, X. Xu, L. Li, H. Zhai and Z. Li, J. Agric. Food Chem., 2010, 58, 2741–2745 CrossRef CAS PubMed.
- Z. Tian, S. Cui, L. Dongmei and Z. Xu, Res. Chem. Intermed., 2015, 41, 7437–7447 CrossRef CAS.
- C. Sun, X. Xu, Y. Xu, D. Yan, T. Fang and T. Liu, J. Agric. Food Chem., 2011, 59, 4828–4835 CrossRef CAS PubMed.
|
This journal is © The Royal Society of Chemistry 2022 |