DOI:
10.1039/D2RA04573G
(Paper)
RSC Adv., 2022,
12, 24088-24100
Degradation of sulfadiazine in aqueous media by peroxymonosulfate activated with biochar-supported ZnFe2O4 in combination with visible light in an internal loop-lift reactor†
Received
23rd July 2022
, Accepted 15th August 2022
First published on 24th August 2022
Abstract
Solid waste resource utilization and the treatment of wastewater are two important aspects in environmental protection. Here, biochar (BC) derived from municipal sewage sludge has been combined with ZnFe2O4 to form the photocatalyst ZnFe2O4/biochar (ZnFe/BC), and it was used to degrade sulfadiazine (SDZ) in the presence of peroxymonosulfate (PMS) under visible (Vis) light irradiation in an internal loop-airlift reactor (ALR). The surface morphology and structure of ZnFe/BC have been characterized by X-ray diffraction (XRD), scanning electron microscopy equipped with an attachment for energy-dispersive spectroscopy (SEM-EDS), X-ray photoelectron spectroscopy (XPS), and UV-Vis diffuse reflectance spectroscopy (UV-Vis DRS). ZnFe/BC displays outstanding photocatalytic performance and reusability. After four reuse cycles of ZnFe/BC in the Vis/ZnFe/BC/PMS system, the SDZ degradation rate and efficiency still reached 0.082 min−1 and 99.05%, respectively. Reactive species in this system included free radicals SO4˙−, ˙OH, and ˙O2−, as well as non-radicals 1O2, e−, and h+, as established from the results of chemical quenching experiments and electron paramagnetic resonance (EPR) analyses. Moreover, a mechanism of action of the Vis/ZnFe/BC/PMS system for SDZ degradation was proposed. The acute toxicity of the reaction solution towards Photobacterium phosphoreum T3 spp. in the Vis/ZnFe/BC/PMS process increased during the first 40 min and then decreased, illustrating that Vis/ZnFe/BC/PMS provided an effective and safe method for the removal of SDZ.
1. Introduction
With the rapid development of the social economy and increase of peoples' quality of life, sulfonamide antibiotics have been used globally to cure infectious diseases in humans as well as in farmed livestock and poultry as a food supplement.1–3 However, these antibiotics cannot be completely decomposed by animals or humans and are released to the environment through excretion, causing serious harm to the ecosystem due to their chronic toxicity and broad-spectrum antibacterial properties.2–4 SDZ, as a typical antibiotic, has been widely used and is frequently detected in environmental media.5,6 Moreover, it is difficult to effectively degrade SDZ by traditional biological methods due to its good solubility and persistence in the aquatic environment.6 Thus, an effective treatment method is required to treat SDZ-contaminated wastewater before it is released to municipal wastewater treatment plants (WWTPs).
Sulfate radical (SO4˙−)-based advanced oxidation processes (SR-AOPs) represent effective treatment or detoxification methods for wastewater contaminated with refractory organics.7–10 This is because SO4˙− has high average redox potential, good solubility, wide pH flexibility, and a long half-life.6,10–14 PMS, as a source of SO4˙− in SR-AOPs, has been advantageously applied in the remediation of wastewater due to its ease of storage and convenient transportation. PMS may be activated by transition metal ions,15 ultraviolet light,14,16–18 heat,19 metal oxides,20–22 or carbonate-rich materials,23–28 to produce highly reactive species, such as SO4˙− and hydroxyl radicals (˙OH).
Among these activation methods, heterogeneous photocatalytic systems constitute an effective approach for improving the degradation of recalcitrant pollutants due to their low negative impact on water quality and excellent separation of the catalyst from wastewater, such that a synergistic effect has been observed by some researchers.29–31 ZnFe2O4, as a typical representative of bimetallic oxides, has attracted widespread interest because of the natural abundances and nontoxicity of its constituent metals, as well as its high theoretical specific capacitance, narrow band gap, and desirable optical structure, which permit its use as a photocatalyst.32–34 However, the application of pure ZnFe2O4 is limited by its low valence-band (VB) potential, rapid recombination of charge carriers, and aggregation.35–37 Some carbon-based materials have been employed to overcome these bottlenecks, which show excellent activity due to their high conductivity, large surface area, and better charge separation.21,38,39 Graphene and carbon nanotubes have been used as support materials, but their practical applications are limited by their complicated preparation processes.21 Meanwhile, BC is finding increasing applications as a support material and adsorbent due to its simple preparation process and low cost.40–43 In addition, oxygen-containing functional groups on the surface of BC, such as –COOH and C–OH, can activate PMS to generate reactive species.42,43 However, we are not aware of any previous report on visible-light irradiation enhanced BC-supported ZnFe2O4 for PMS activation (Vis/ZnFe/BC/PMS).
In the present study, SDZ was selected as a target antibiotic to assess the enhancing effect of combining PMS with visible light irradiation and ZnFe/BC in ALR. Compared with a mechanically stirred batch reactor or a bubble column reactor, the ALR can provide better mixing, high gas–liquid–solid mass transfer, and low shear stress, resulting in its extensive application in chemical engineering and biotechnology.44,45 Therefore, the primary objectives of this study were to test the catalytic ability and efficiency of ZnFe/BC for PMS activation and to delineate the reaction mechanism of the Vis/ZnFe/BC/PMS system for the degradation of SDZ in an ALR. Finally, the acute toxicity of the reaction effluent was analyzed.
2. Materials and methods
2.1. Materials
SDZ (C10H9N4NaO2S, >98%), 2,2,6,6-tetramethyl-4-piperidinol (TMP), and 5,5-dimethyl-1-pyrrolidine N-oxide (DMPO) were purchased from Aladdin Industrial Corporation (Shanghai, China). PMS (KHSO5·0.5KHSO4·0.5K2SO4, 4.5% active oxygen) was purchased from Macklin Industrial Corporation (Shanghai, China). Ethanol (EtOH), tert-butyl alcohol (TBA), potassium dichromate (K2Cr2O7), ethylenediamine tetraacetic acid (EDTA), 1,4-benzoquinone (BQ), iron chloride (FeCl3), sodium azide (NaN3), acetonitrile (C2H3N), formic acid (CH2O2), and zinc chloride (ZnCl2) were supplied by Sinopharm Chemical Reagent Co., Ltd. (Shanghai, China). All chemicals were of analytical grade or better and were used as received. All solutions were prepared with deionized water. Dewatered sewage sludge was collected from a municipal wastewater treatment plant in Chuzhou, China.
2.2. Preparation of BC and ZnFe/BC
BC was fabricated by directly calcining drying sewage sludge powder. Dewatered sewage sludge was firstly dried in an oven at 80 °C. It was then smashed and sifted through an 80 mesh sieve. Finally, the sludge powder was transferred into a ceramic boat and was pyrolyzed at 500 °C for 2 h in a tubular reactor (SK-G04123K, China) in N2 atmosphere.
ZnFe/BC was synthesized by a hydrothermal method.46,47 BC (1 g) was fully dispersed in deionized water (80 mL) in a beaker with the aid of ultrasonication. ZnCl2 (10 mmol) and FeCl3 (20 mmol) were then added and the mixture was stirred for 100 rpm by means of a mechanical agitator (Eurostar 20 Digital, IKA RW20, Germany) for 20 min. Thereafter, NaOH (1 g) was added to the mixture, which converted it into a brown suspension. This brown suspension was stirred for 10 min and then poured into a 100 mL Teflon-lined stainless steel autoclave. The autoclave was covered and placed in an oven at 180 °C for 24 h. After allowing the autoclave to cool to room temperature, the obtained precipitate was washed several times with deionized water, and then dried at 80 °C for 12 h. The obtained ZnFe/BC was stored in a sample bottle prior to use.
2.3. Experimental procedures
All experiments were performed in an ALR containing 30 mg L−1 SDZ solution (1.5 L) at room temperature (25 °C) under irradiation from a lamp. The detailed structure and dimensions of the ALR (Fig. S1†) have been described in a previous report.48 The requisite compressed gas was injected continuously (40 mL min−1) for 10 min before the experiment from the bottom of the ALR to circulate the catalyst particles throughout the reactor. An Xe lamp with a cut-off filter (≥400 nm) (Heraeus-6, 300 W, providing simulated solar light) was fixed inside a cylindrical Pyrex tube. The Xe lamp was turned on for 30 min before starting the experiment to stabilize its radiation. The requisite amounts of ZnFe/BC and PMS were then added to the ALR, which was taken as t = 0 with regard to the reaction time. Aliquots (1 mL) were withdrawn at certain intervals, filtered through a 0.22 μm membrane, and immediately quenched reaction with 20 μL EtOH prior to analysis.
2.4. Analytical methods
The residual SDZ concentration was determined by high-performance liquid chromatography (HPLC, LC-20AB). The HPLC system was equipped with an SPDM20A chromatograph, an LC-20AB pump, and an SPD-10A UV/Vis detector set at a wavelength of 265 nm. A shim-pack VP-ODS C18 column (4.6 mm × 250 mm, 5 μm) was used for separation. The mobile phase was a mixture of acetonitrile and 0.1% (v/v) aqueous formic acid (30
:
70, v/v) and the flow rate was set at 1 mL min−1. The degradation efficiency (R) of SDZ was calculated according to eqn (1): |
R (%) = (1 − C/C0) × 100
| (1) |
where C0 and C refer to the SDZ concentrations at time 0 and t min. The total organic carbon (TOC) was measured using a Shimadzu TOC analyzer (TOC-L series, Shimadzu, Japan).
The morphologies of the catalysts were inspected with SEM-EDS (Hitachi S-3000N, Japan). The XRD patterns of BC and ZnFe/BC were collected on a D8 Advance spectrometer (Bruker, Germany) operated at 40 kV and 30 mA, employing Cu-Kα radiation (λ = 1.5406 Å). The surface chemical state was assessed by XPS (Thermo Fisher, USA) with a monochromated Al-Kα X-ray source. Diffuse reflectance spectra were collected on a UV visible spectrophotometer (UV 3600, Shimadzu, Japan) equipped with a diffuse reflectance measurement accessory and BaSO4 was used as a reflectance standard.
The active species generated in the Vis/PMS, Vis/BC/PMS, and Vis/ZnFe/BC/PMS systems were identified by EPR on an A300-10/12 spectrometer (Bruker, Germany) with a microwave bridge (modulation amplitude 2 G, microwave power 20 mW, modulation frequency 100 kHz). Metal ions (Zn2+ and Fe3+) leaching from the catalyst were quantified by atomic absorption spectrometry (ZEEnit 700, Germany).
2.5. Acute toxicity assay
Active toxicities were determined towards luminescent bacteria based on the Chinese standard for water quality determination of acute toxicity (GB/T 15441-1995). Each test was carried out in six replicates.
Freeze-dried powder of Photobacterium phosphoreum T3 spp. was purchased from the Institute of Soil Science, Chinese Academy of Sciences (Nanjing, China) and was reconstituted with 3% NaCl solution prior to testing.49 The variation of luminescence was detected using a Toxicity Determinator (DXY-3, Institute of Soil Science, Chinese Academy of Sciences, Nanjing, China). In a blank test, the effluent of SDZ degradation was replaced by deionized water. The toxicity was characterized by the percentage inhibition of luminosity (X) according to the following equation (eqn (2)):
|
 | (2) |
where CK denotes the blank sample.
3. Results and discussion
3.1. Characterization of catalysts
The morphologies and elemental compositions of BC and ZnFe/BC were determined by SEM-EDS, and the results are shown in Fig. 1a–c. As shown in Fig. 1a and b, BC obtained from sewage sludge by calcination exhibited a rough surface, with C, O, and Si as its main elements. Fig. 1c reveals many spherical particles on the surface of ZnFe/BC such as the yellow mark, and Fig. 1d shows the atomic ratio of Zn to Fe to be approximately 1
:
2, suggesting that these spherical particles may consist of ZnFe2O4.
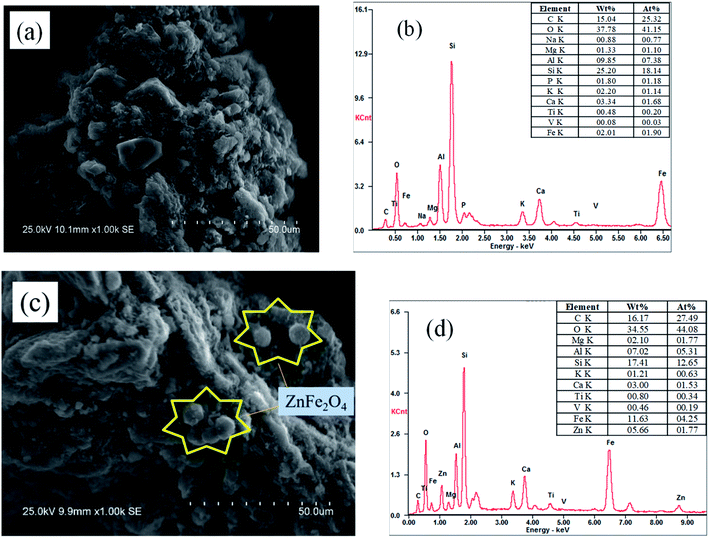 |
| Fig. 1 SEM images of (a) BC and (c) ZnFe/BC, EDS spectrum of (b) BC and (d) ZnFe/BC. | |
XRD spectral data shown in Fig. 2 were used to further confirm the crystallinity of the synthesized catalysts. The XRD peaks of BC at 19.73°, 23.86°, 26.03°, 26.72°, and 28.69° corresponded to the (111), (200), (201), (210), and (211) planes and are consistent with the standard reference file of SiO2 (JCPDS card no. 76-0912). Besides the obvious peak of BC at 26.03°, ZnFe/BC also displayed a well-defined crystal structure, with peaks at 29.92°, 35.26°, 42.88°, 56.63°, and 62.12° attributable to the (220), (311), (400), (511), and (440) planes of spinel ZnFe2O4 (JCPDS card no. 22-1012).50,51 Overall, the main component on the surface of BC was ZnFe2O4, consistent with the SEM-EDS results.
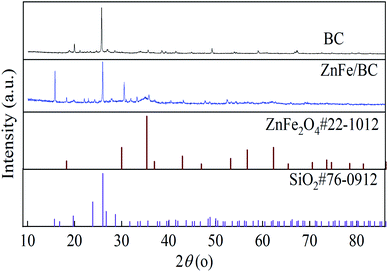 |
| Fig. 2 XRD patterns of BC and ZnFe/BC. | |
XPS was employed to further interpret the chemical state of the prepared ZnFe/BC sample, and the results are shown in Fig. 3. As can be seen from the survey spectrum in Fig. 3a, the binding energy peaks for the different electronic states confirmed the presence of Zn, Fe, C, and O. The two characteristic Fe 2p peaks seen in Fig. 3b at 711.2 and 724.5 eV corresponded to Fe 2p3/2 and Fe 2p1/2, respectively.32,51 Interestingly, the two deconvoluted Fe 2p peaks could be resolved to the Fe3+ state at binding energies of 710.5 and 726.5 eV and the Fe2+ state at 712.6 and 724.4 eV. The satellite peak at 720.1 eV also stemmed from the Fe3+ state.32 The Zn 2p spectrum of ZnFe/BC in Fig. 3c features two peaks at 1021.8 eV and 1044.9 eV, attributable to Zn 2p3/2 and Zn 2p1/2, as expected for Zn2+.33,52 Fig. 3d shows the C 1s spectrum of ZnFe/BC, which could be deconvoluted into four peaks at 283.5, 285.0, 286.1, and 288.0 eV, attributable to graphitic C, C–H or C–C, C–OH, and C
O, respectively.33,53 The O 1s spectrum is depicted in Fig. 3e and could be deconvoluted into three peaks at 529.7, 531.5, and 532.6 eV, reflecting the presence of Fe–O/Zn–O, Fe–OH/Zn–OH, and –OH.32,54 The presence of these elements in their respective states corroborated the formation of ZnFe2O4 composite on the surface of BC.
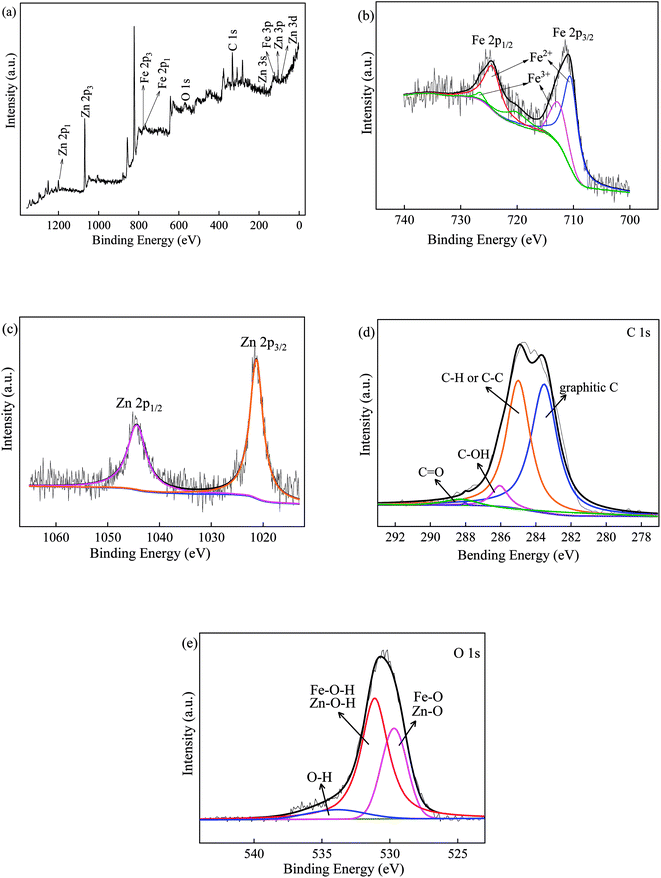 |
| Fig. 3 XPS profile of ZnFe/BC: (a) survey and core spectra of (b) Fe, (c) Zn, (d) C and (e) O. | |
The UV-Vis DRS spectra of BC, ZnFe2O4, and ZnFe/BC are displayed in Fig. 4a and revealed that the photocatalysts exhibited wide visible-light absorption capacity. Based on the Kubelka–Munk equation and the Tauc relationship (eqn (3) and (4)),37,55,56 the band-gap energies (Eg) of BC, ZnFe2O4, and ZnFe/BC were calculated as 2.46, 1.95, and 2.16 eV, respectively, from plots of (αhv)2 versus energy (hv) (Fig. 4b).
Here,
R is the diffuse reflectance,
F(
R) is the transformed reflectance according to the Kubelka–Munk equation coefficient,
h is Planck's constant,
v is the frequency of the light,
A is a material constant, and
α is the absorption coefficient, which is equivalent to
F(
R) numerically.
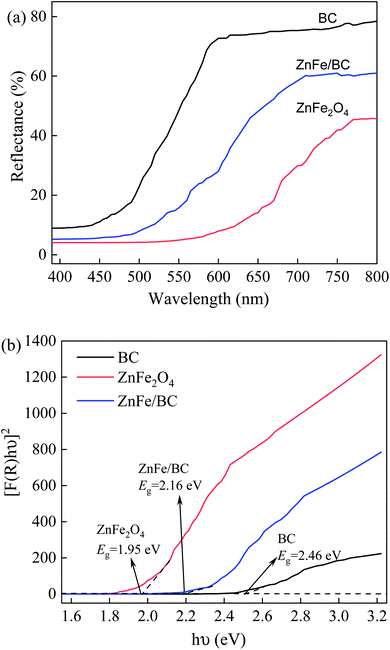 |
| Fig. 4 UV-Vis DRS spectra of the catalyst samples (a) reflectance spectra and (b) Kubelka–Munk model of BC, ZnFe2O4 and ZnFe/BC. | |
3.2. High catalytic activity and reusability of ZnFe/BC
In order to illustrate the catalytic performance of ZnFe/BC in Vis/ZnFe/BC/PMS, the degradation of SDZ was performed in various systems at natural pH (6.08). The loading of ZnFe/BC was 0.5 g L−1 and the SDZ concentration was 30 mg L−1. The results are depicted in Fig. 5. Evidently, negligible degradation of SDZ was obtained with visible-light irradiation alone, reflecting its high stability. Direct oxidation of SDZ by PMS was also only slight due to the limited oxidizing ability of PMS (E0 = 1.82 V).57 Adsorption of SDZ on the surfaces of BC or ZnFe/BC was less than 9%. Therefore, no high catalytic activity of ZnFe/BC under visible-light irradiation was observed. Obviously, compared with pure physico-chemical adsorption, the degradation capacities were significantly enhanced in the presence of PMS. As shown in Fig. 5, the removal efficiency of SDZ was 6.42% in the Vis/PMS system, illustrating that visible-light irradiation has only weak activating ability and cannot activate PMS. Although the use of MIL-100(Fe)/ZnFe2O4/flake-like porous carbon nitride has been reported for the photocatalytic degradation of nonylphenol (NP) under illumination from an Xe lamp, the generation of ˙O2− radicals contributed to NP degradation.58 Light from an Xe lamp was not powerful enough (350 W) to efficiently generate free radicals such as ˙O2−, leading to an unfavorable ability to degrade SDZ. Interestingly, the removal efficiency of SDZ reached 39.6% in the BC/PMS system, suggesting that PMS was activated by BC to generate reactive oxygen species for SDZ degradation.42,43 The composite sample showed stronger catalytic performance for PMS and the degree of decomposition was significantly improved to 80% in the ZnFe/BC/PMS system. The catalytic degradation of SDZ followed pseudo-first-order kinetics. The calculated reaction rate constants (k) are summarized in Table S1.† The SDZ degradation efficiency was significantly improved by the introduction of visible-light irradiation into the ZnFe/BC/PMS system and increased to 98.8%. Moreover, the decomposition rate constant of SDZ in the Vis/ZnFe/BC/PMS system (0.082 min−1) was 3.56 times larger than that in the ZnFe/BC/PMS system (0.018 min−1). This further confirmed that the synergistic interaction between ZnFe/BC and visible-light irradiation in enhancing PMS activation resulted in an obvious improvement of catalytic performance.
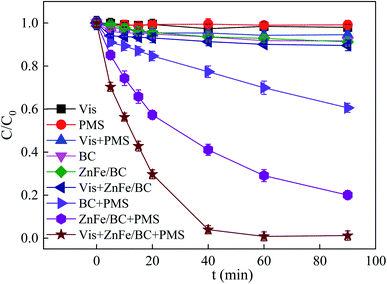 |
| Fig. 5 Degradation of SDZ under different reaction conditions (C0 = 30 mg L−1, pH = 6, PMS = 5 mmol L−1, ZnFe/BC = 0.5 g L−1, λ ≥ 400 nm). | |
Recyclability and stability are important factors for practical applications. Therefore, the reusability of ZnFe/BC was assessed by monitoring the degradation of SDZ over four cycles under the same operation conditions, and the results are shown in Fig. 6a. It can be seen in Fig. 6a and b that the extents of removal of SDZ in the Vis/ZnFe/BC/PMS system over the four cycles ranged from 97.8% to 99.1%, with the leaching of Zn and Fe ions not exceeding 0.41 mg L−1 and 0.39 mg L−1, respectively. The low leaching concentrations of Zn and Fe in the effluent are within the limits stipulated by the Discharge Standard of Pollutants for Municipal Wastewater Treatment Plant (GB18918-2002) in China. Meanwhile, the ZnFe/BC particles maintained their initial crystallinity after four cycles (Fig. 6c), indicating excellent stability and reusability.
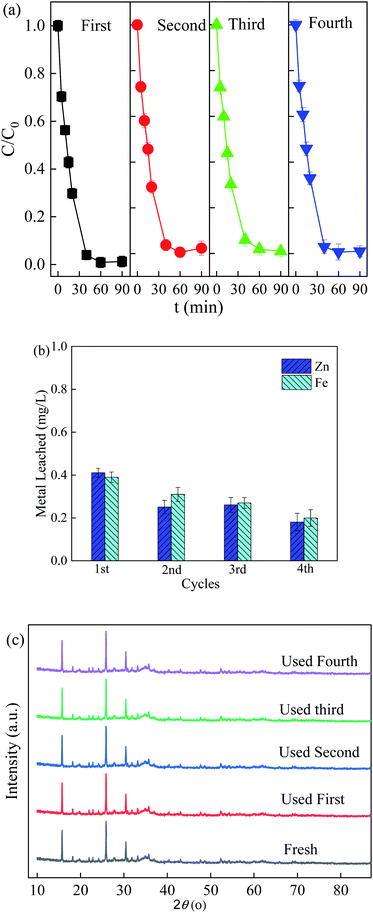 |
| Fig. 6 (a) Reusability of ZnFe/BC in the Vis/ZnFe/BC/PMS system (C0 = 30 mg L−1, pH = 6, PMS = 5 mmol L−1, ZnFe/BC = 0.5 g L−1, λ ≥ 400 nm); (b) content of metal elements in the leaching solutions; (c) XRD patterns of fresh and used ZnFe/BC. | |
3.3. Performance comparison of dissolved gas and reactor
The degradation of SDZ by PMS activated by ZnFe/BC under visible light (Vis) irradiation was performed in both the abovementioned ALR and a conventional bubble column reactor (BCR; the ALR without the internal draft tube). As shown in Fig. 7, a higher degradation extent and removal of SDZ were achieved in the ALR compared to those in the conventional BCR. The degree of degradation and removal rate of SDZ in the ALR were 98.8% and 0.082 min−1, respectively, as compared to 85.9% and 0.030 min−1 in the BCR. Compared with the BCR, the ALR with an internal draft tube allowed for smooth and steady circulation of the catalyst particles in the reactor, which favored extensive contact between the reactants as well as high mass- and heat-transfer rates. Therefore, the ALR was used in subsequent experiments due to its superior performance.
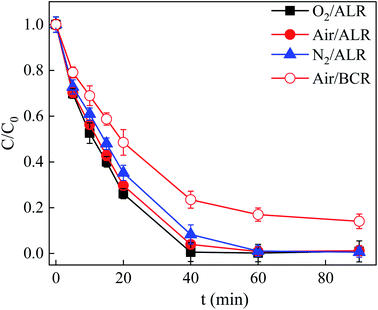 |
| Fig. 7 Effect of dissolved gas on SDZ degradation in ALR and BCR (C0 = 30 mg L−1, pH = 6, PMS = 5 mmol L−1, ZnFe/BC = 0.5 g L−1, λ ≥ 400 nm). | |
Some studies have shown that superoxide free radical (˙O2−) is generated through electron transfer to dissolved O2.59,60 Thus, experiments in different atmospheres (N2, O2, and air) were carried out to confirm the existence and contribution of ˙O2− in the Vis/ZnFe/BC/PMS system. The role of O2 in the degradation of SDZ was investigated by bubbling N2 or O2 instead of air in the Vis/ZnFe/BC/PMS system. As indicated in Fig. 7, the degradation efficiency of SDZ decreased from 98.8% to 88.0% and the degradation rate decreased from 0.082 min−1 to 0.075 min−1 when air was replaced by N2, presumably because less ˙O2− was formed.61 The SDZ degradation efficiency and removal rate increased to 99.1% and 0.115 min−1, respectively, when air was replaced by pure O2 such that there was more dissolved O2 and hence more ˙O2−.61
3.4. Effects of operating parameters
Considering practical application of the Vis/ZnFe/BC/PMS system, the effects of ZnFe/BC dosage, PMS dosage, and initial pH on SDZ degradation were studied, and the results are shown in Fig. 8. It can be seen from Fig. 8a that the degradation efficiency of SDZ was obviously increased from 40.2% to 98.8% with increasing PMS dosage from 1 to 5 mmol L−1 at 30 mg L−1 SDZ concentration, natural initial pH 6.0, and 0.5 g L−1 ZnFe/BC. This implied that increased amount of oxidant was directly correlated with the quantities of reactive radicals produced by its decomposition. However, when the PMS concentration was further increased to 7 mmol L−1, the SDZ degradation efficiency and rate tended to saturation. The active sites of ZnFe/BC at 0.5 g L−1 addition were occupied by 5 mmol L−1 PMS and the limited activation ability of Vis could not significantly enhance the degradation of SDZ. No further sites were available when the PMS concentration was increased to 7 mmol L−1. A similar phenomenon was reported by Fan and co-workers when employing PMS activated by a sewage sludge biochar-based catalyst to degrade bisphenol A.43
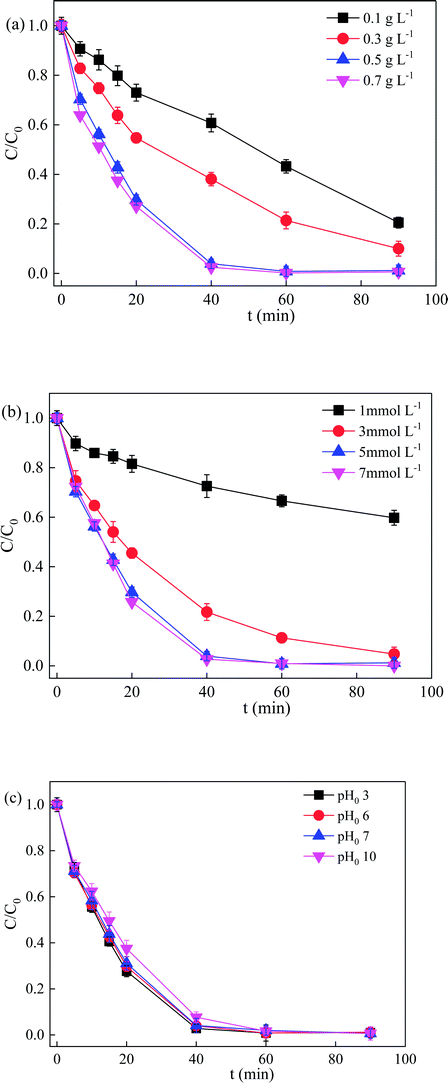 |
| Fig. 8 Effect of reaction conditions for the degradation of SDZ: (a) catalyst dosage, (b) PMS concentration, and (c) initial pH0 (C0 = 30 mg L−1, pH = 6, PMS = 5 mmol L−1, ZnFe/BC = 0.5 g L−1, λ ≥ 400 nm). | |
SDZ removal rates at various ZnFe/BC dosages were investigated (Fig. 8b) at 5 mmol L−1 PMS, 30 mg L−1 SDZ, and initial pH 6. For increasing ZnFe/BC loadings of 0.1, 0.3, 0.5, and 0.7 g L−1, the SDZ degradation efficiency and rate constant increased from 79.5% to 99.4% and from 0.013 min−1 to 0.091 min−1, respectively. A higher dosage of catalyst provides more active sites for PMS activation and SDZ degradation.
The influence of varying pH0 from 3 to 10 was examined using the Vis/ZnFe/BC/PMS system for SDZ degradation (Fig. 8c). SDZ degradation efficiencies remained similar over a wide pH range. When the pH0 was increased from 3 to 10, the rate constant decreased significantly from 0.084 to 0.069 min−1, while the degradation efficiency in 90 min changed very slightly from 99.0% to 99.4%. The promising removal results demonstrated that the ZnFe/BC catalyst may be applied over a wide range of initial pH (3–10) for the activation of PMS under visible-light irradiation.
3.5. Reaction mechanism
In order to delineate the photocatalytic reaction mechanism, a series of radical quenching experiments was conducted to ascertain the main active species. The scavengers EtOH, TBA, BQ, NaN3, K2Cr2O7, and EDTA were employed to quench SO4˙− and ˙OH, ˙OH, ˙O2−, 1O2, e−, and h+, respectively. As shown in Fig. 9, the SDZ degradation rate in the presence of TBA decreased from 0.082 to 0.058 min−1, suggesting that ˙OH played a limited role in the process. Similarly to TBA, when EtOH, as a quencher of both SO4˙− and ˙OH, was added to the reaction solution, the SDZ removal rate decreased to 0.038 min−1, implying that both SO4˙− and ˙OH were generated in the Vis/ZnFe/BC/PMS system. However, adding excess EtOH or TBA did not fully suppress SDZ removal, demonstrating that other active oxygen species were also operative in the reaction solution. Moreover, previous literature reports have indicated that ˙O2− may be generated during the PMS activation process.37,62,63 Therefore, BQ was selected to verify the involvement of ˙O2− due to the high rate constant of their reaction
.62 Indeed, when BQ was introduced, the SDZ degradation rate decreased significantly to 0.028 min−1, suggesting that ˙O2− played an important role in the process. In addition, 1O2 also contributed to the degradation of SDZ, as proved by the addition of NaN3. Clearly, the introduction of NaN3 had a suppressing effect on the degradation of SDZ, revealing that 1O2 also has a notable impact in the Vis/ZnFe/BC/PMS system. The elimination rate of SDZ decreased from 0.082 to 0.036 min−1. Besides, EDTA and K2Cr2O7 are widely utilized to investigate the presence of photogenerated h+ and e−, respectively. As shown in Fig. 9, the SDZ degradation efficiency decreased from 98.78% to 80.85% and 83.6%, while the rate constant decreased from 0.082 min−1 to 0.022 min−1 and 0.029 min−1, when EDTA and K2Cr2O7 were added, respectively, revealing that both h+ and e− played important roles in the degradation of SDZ.
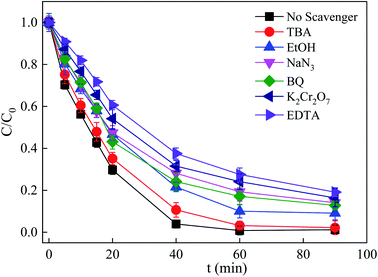 |
| Fig. 9 Effect of radical scavengers on the degradation of SDZ (C0 = 30 mg L−1, pH = 6, PMS = 5 mmol L−1, ZnFe/BC = 0.5 g L−1, CTBA = CEtOH = 100 mmol L−1, CBQ = CNaN3 = CK2Cr2O7 = CEDTA = 10 mmol L−1, λ ≥ 400 nm). | |
To further confirm the reactive species involved in the degradation of SDZ in the Vis/ZnFe/BC/PMS system, EPR measurements were carried out in the presence of DMPO and TMP, and the results are shown in Fig. 10. It can be seen from Fig. 10a that strong 1
:
2
:
2
:
1 quartet and 1
:
1
:
1
:
1
:
1
:
1 sextet characteristic signals in the combination systems (such as BC/PMS, ZnFe/BC/PMS, and Vis/ZnFe/BC/PMS) were detected due to DMPO adduct formation, confirming the presence of ˙OH and SO4˙−. However, only low-intensity signals were observed in Vis/PMS system because of low catalytic capacity of Vis for PMS. Compared with the combination systems, these peaks were inconspicuous in only PMS, illustrating that ˙OH and SO4˙− could not be generated through the self-decomposition of PMS. Meanwhile, a significant triplet signal of 1O2 (1
:
1
:
1 ratio) could be seen from Fig. 10b in the presence of TMP as a spin-trapping agent, which implies that abundant 1O2 was generated in the BC/PMS, ZnFe/BC/PMS, and Vis/ZnFe/BC/PMS system. Similarly, the triplet signal of 1O2 was weak in Vis/PMS, while it was inconspicuous in only PMS. This further revealed that 1O2 could been produced via activating PMS by BC, ZnFe/BC, and the combination of ZnFe/BC and Vis.
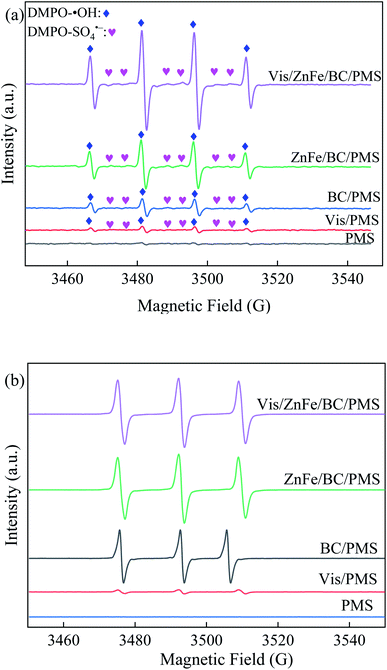 |
| Fig. 10 ESR spectra of (a) DMPO-˙OH and DMPO-SO4˙℡, (b) TMP-1O2 signals in PMS, Vis/PMS, BC/PMS, ZnFe/BC/PMS, and Vis/ZnFe/BC/PMS systems (CDMPO = 10 mg L−1, CTMP = 10 mg L−1, pH = 6, PMS = 5 mmol L−1, ZnFe/BC = 0.5 g L−1). | |
The energy band positions of ZnFe2O4 and BC were investigated in order to obtain deeper insight into the photocatalytic mechanism. Mott–Schottky analysis was employed with an initial frequency of 1000 Hz, and the results are shown in Fig. 11a. By extrapolating the graph to 1/C2 = 0, the slope of the plot intercepts the x-axis at the flat band potential (Efb), where Efb is roughly equal to the band energy for most semiconductors. Moreover, a positive slope indicates the conduction band (CB) potential (ECB) for an n-type semiconductor, whereas a negative slope indicates the valence band (VB) potential (EVB) for a p-type semiconductor.56 In this way, the CB energy of ZnFe2O4 was obtained as 2.35 eV and the VB energy of BC was obtained as −0.73 eV with respect to Ag/AgCl. According to the UV/Vis DRS analysis results, the EVB of ZnFe2O4 and the ECB of BC were calculated according to the following formula56,64,65 as 0.40 eV and 1.73 eV, respectively.
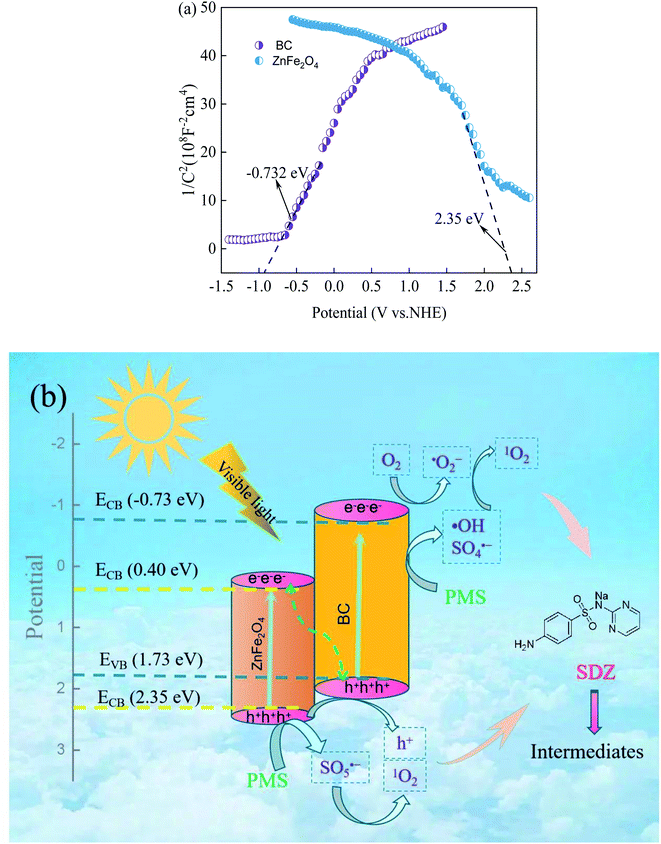 |
| Fig. 11 (a) Mott–Schottky plots of ZnFe2O4 and BC and (b) The possible removal mechanism of SDZ by Vis/ZnFe/BC/PMS system. | |
Based on the above discussion and analysis, a preliminary photocatalytic degradation mechanism for SDZ by PMS activated by ZnFe/BC under visible light may be proposed, as shown in Fig. 11b. BC showed excellent catalytic ability towards PMS due to its abundant oxygenated functional groups, which serve to effectively generate 1O2, ˙OH, and SO4˙−.66–68 ZnFe/BC was excited under visible-light irradiation to produce e℡ and h+ (eqn (6)). Photogenerated e℡ in BC were captured by adsorbed O2 to produce ˙O2− according to eqn (7),59,60 owing to its more negative CB than that of O2/˙O2− (−0.33 eV).69,70 The photogenerated e− could easily migrate from the CB of ZnFe2O4 to the h+ in the VB of BC and recombine with them. Moreover, the h+ in ZnFe2O4 showed strong oxidizing ability and could directly degrade pollutants, and could also activate PMS to generate SO5˙− according to eqn (8).37 Both the self-reaction of SO5˙− and the reaction between ˙O2− and ˙OH (eqn (9) and (10)) could generate 1O2.37 The photogenerated e℡, h+, ˙O2−, 1O2, SO4˙−, and ˙OH reacted with SDZ through free radical and non-radical pathways to produce intermediates, achieving its efficient removal (eqn (11)).
|
ZnFe/BC + hv → ZnFe/BC (e− + h+)
| (6) |
|
HSO5− + h+ → SO5˙− + H+
| (8) |
|
SO5˙− + SO5˙− → 2SO4˙− + 1O2
| (9) |
|
˙O2− + ˙OH → OH− + 1O2
| (10) |
|
e−/h+/1O2/˙O2−/˙OH/SO4˙− +SDZ → intermediates
| (11) |
3.6. TOC and toxicity evaluation of effluent
Complete degradation of SDZ does not mean that it was completely oxidized to harmless small molecules, such as CO2, H2O, and NO3−, but the TOC removal gives a measure of the mineralization degree of organic pollutants in AOPs.71,72 Thus, the TOC removal of the SDZ solution was explored, and the results are shown in Fig. 12. The extent of SDZ degradation reached 96.05% after 40 min of reaction, but the TOC removal was less than 24%. On further extending the treatment to 2 h, the TOC removal efficiency displayed a satisfactory result (approximately 64.8%), revealing that recalcitrant intermediates were formed.
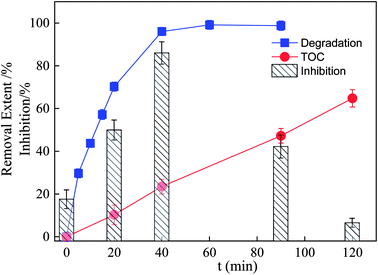 |
| Fig. 12 Changes of acute toxicity and TOC removal during SDZ degradation process (C0 = 30 mg L−1, pH = 6, PMS = 5 mmol L−1, ZnFe/BC = 0.5 g L−1, λ ≥ 400 nm). | |
Compared to the parent compound, more toxic intermediates are usually produced during the photocatalytic treatment of antibiotics.73–75 Thus, the acute toxicity was investigated towards luminescent bacteria, and the results are shown in Fig. 12. It can be seen from Fig. 12 that the luminescence inhibition ratio increased from 17.43% to 85.97% within the first 40 min, proving that some toxic intermediates were produced at the beginning of the reaction. The luminescence inhibition ratio then decreased sharply to just 6.41% at 120 min, demonstrating that SDZ and its intermediates had been significantly decomposed into low/nontoxic compounds by the Vis/ZnFe/BC/PMS system. Hence, the present system could be described as ecotoxicologically safe.
4. Conclusion
ZnFe2O4 has been combined with BC to form ZnFe/BC and ZnFe/BC was applied as an efficient PMS activator to achieve efficient SDZ elimination under visible light irradiation in an internal loop-lift reactor. The photocatalytic performance of ZnFe/BC has been assessed on the basis of its structure, activity, stability, and reusability towards SDZ in the visible-light-induced process. The catalyst displayed excellent degradation performance, and EPR and chemical quenching tests revealed that e−, h+, 1O2, ˙O2−, ˙OH, and SO4˙− were responsible for the removal of SDZ. The Vis/ZnFe/BC/PMS system is efficient over a wide pH range. In addition, the mineralization rate of SDZ and biotoxicity be considered before practical applications. The TOC removal only reached 64.8% after 2 h reaction, but the acute toxicity of the effluent achieved the satisfactory effect. The acute toxicity of the effluent during the SDZ degradation process first increased to 85.97% after 40 min of reaction, but then gradually decreased to 6.41%. In summary, the findings of this study provide a novel method for enhancing the photo-catalytic effect of ZnFe/BC in PMS activation systems. Moreover, the development of ZnFe/BC constituted an effective method for solid waste resource utilization and the remediation of antibiotic-contaminated wastewater.
Author contributions
Yan Wang: Investigation, Methodology, Data curation, Formal analysis, Visualization, Writing – original draft, Writing – review & editing, Supervision. Tao Gan: Investigation, Data curation. Jingyu Xiu: Investigation, Data curation. Ganghua Liu: Conceptualization, Data curation, Methodology. Haiming Zou: Formal analysis, Funding acquisition, Supervision.
Conflicts of interest
There are no conflicts to declare.
Acknowledgements
This study was supported by the Natural Science Foundation of Anhui Province (No. 1808085MB49, 2008085ME169, 2108085MD140, 2108085QB56), in China; Innovation and Entrepreneurship Training Program for College Students in Anhui Province (S202110879254, S202110879251).
References
- J. C. Serna-Carrizales, V. H. Collins-Martínez, E. Flórez, C. F. A. Gomez-Duran, G. Palestino and R. Ocampo-Pérez, J. Mol. Liq., 2021, 324, 114740 CrossRef CAS.
- Y. Liu, X. Zhang, J. Deng and Y. Liu, Chemosphere, 2021, 277, 130305 CrossRef CAS PubMed.
- F. Zhu, J. Pan, Q. Zou, M. Wu, H. Wang and G. Xu, Chemosphere, 2021, 274, 129713 CrossRef CAS PubMed.
- L. Zhu, Z. Shi, L. Deng and Y. Duan, Colloids Surf., A, 2021, 609, 125637 CrossRef CAS.
- W. Yang, P. Hong, D. Yang, Y. Yang, Z. Wu, C. Xie, J. He, K. Zhang, L. Kong and J. Liu, J. Colloid Interface Sci., 2021, 597, 56–65 CrossRef CAS PubMed.
- H. Cui, Y. Tian, J. Zhang, S. Ma, L. Li, W. Zuo, L. Zhang and T. Wang, Chem. Eng. J., 2021, 417, 128152 CrossRef CAS.
- Z. Y. Lu, Y. L. Ma, J. T. Zhang, N. S. Fan, B. C. Huang and R. C. Jin, J. Water Proc. Eng., 2020, 38, 101681 CrossRef.
- M. Wang, C. Jin, J. Kang, J. Liu, Y. Tang, Z. Li and S. Li, Chem. Eng. J., 2021, 416, 128118 CrossRef CAS.
- W. Wang, M. Chen, D. Wang, M. Yan and Z. Liu, Sci. Total Environ., 2021, 772, 145522 CrossRef CAS PubMed.
- T. Zhang, Y. Yang, X. Li, H. Yu, N. Wang, H. Li, P. Du, Y. Jiang, X. Fan and Z. Zhou, Sep. Purif. Technol., 2020, 239, 116537 CrossRef CAS.
- W. Guo, Q. Zhao, J. Du, H. Wang, X. Li and N. Ren, Chem. Eng. J., 2020, 388, 124303 CrossRef CAS.
- A. Shabanloo, M. Salari, N. Shabanloo, M. H. Dehghani, C. U. Pittman and D. Mohan, J. Mol. Liq., 2020, 298, 112088 CrossRef CAS.
- S. Xiao, M. Cheng, H. Zhong, Z. Liu, Y. Liu, X. Yang and Q. Liang, Chem. Eng. J., 2020, 384, 123265 CrossRef CAS.
- Y. Lei, J. Lu, M. Zhu, J. Xie, S. Peng and C. Zhu, Chem. Eng. J., 2020, 379, 122339 CrossRef CAS.
- J. Zhang, H. Song, Y. Liu, L. Wang, D. Li, C. Liu, M. Gong, Z. Zhang, T. Yang and J. Ma, Sep. Purif. Technol., 2019, 224, 142–151 CrossRef CAS.
- Z. Lin, W. Qin, L. Sun, X. Yuan and D. Xia, J. Water Process Eng., 2020, 38, 101636 CrossRef.
- Y. Qi, R. Qu, J. Liu, J. Chen, G. Al-Basher, N. Alsultan, Z. Wang and Z. Huo, Chemosphere, 2019, 237, 124484 CrossRef CAS PubMed.
- X. Zhang, Z. Chen, J. Kang, S. Zhao, B. Wang, P. Yan, F. Deng, J. Shen and W. Chu, J. Hazard. Mater., 2021, 401, 123837 CrossRef CAS PubMed.
- H. Milh, D. Cabooter and R. Dewil, Chem. Eng. J., 2021, 422, 130457 CrossRef CAS.
- Y. Nie, H. Zhou, S. Tian, X. Tian, C. Yang, Y. Li, Y. Tian and Y. Wang, Chem. Eng. J., 2022, 427, 130998 CrossRef CAS.
- H. Fu, S. Ma, P. Zhao, S. Xu and S. Zhan, Chem. Eng. J., 2019, 360, 157–170 CrossRef CAS.
- H. L. So, K. Y. Lin, W. Chu and H. Gong, Ind. Eng. Chem. Res., 2020, 59, 4257–4264 CrossRef CAS.
- B.-M. Jun, S. S. Elanchezhiyan, Y. Yoon, D. Wang, S. Kim, S. Muthu Prabhu and C. M. Park, Chem. Eng. J., 2020, 393, 124733 CrossRef CAS.
- J. Wang, J. Cai, S. Wang, X. Zhou, X. Ding, J. Ali, L. Zheng, S. Wang, L. Yang, S. Xi, M. Wang and Z. Chen, Chem. Eng. J., 2022, 428, 131233 CrossRef CAS.
- Y. Yu, N. Li, X. Lu, B. Yan, G. Chen, Y. Wang, X. Duan, Z. Cheng and S. Wang, J. Hazard. Mater., 2021, 421, 126735 CrossRef PubMed.
- S. Wang, L. Xu and J. Wang, Chem. Eng. J., 2022, 428, 131066 CrossRef CAS.
- Y. Wang, Y. Song, N. Li, W. Liu, B. Yan, Y. Yu, L. Liang, G. Chen, L. a. Hou and S. Wang, J. Hazard. Mater., 2022, 421, 126794 CrossRef CAS PubMed.
- Z. Zhang, X. Huang, J. Ma, Z. Pei, L. Luo, X. Ke, F. Qin, Y. Li, R. Yang, Y. Zhu and Q. Zhang, Water Res., 2021, 201, 117288 CrossRef CAS PubMed.
- H. Zhang, Y. Song, L. C. Nengzi, J. Gou, B. Li and X. Cheng, Chem. Eng. J., 2020, 379, 122362 CrossRef CAS.
- A. Eslami, M. Hashemi and F. Ghanbari, J. Clean. Prod., 2018, 195, 1389–1397 CrossRef CAS.
- D. Roy, S. Neogi and S. De, Chem. Eng. J., 2022, 428, 131028 CrossRef CAS.
- B. Joshi, E. Samuel, C. Park, Y. Kim, H. S. Lee and S. S. Yoon, Appl. Surf. Sci., 2021, 559, 149951 CrossRef CAS.
- J. Feng, P. Nian, L. Peng, A. Zhang and Y. Sun, Chemosphere, 2021, 271, 129575 CrossRef CAS PubMed.
- K. Shetty, L. Renuka, H. P. Nagaswarupa, H. Nagabhushana, K. S. Anantharaju, D. Rangappa, S. C. Prashantha and K. Ashwini, Mater. Today Proc., 2017, 4, 11806–11815 CrossRef.
- Y. L. Yan, Q. J. Fang, J. K. Pan, J. Yang, L.-l. Zhang, W. Zhang, G.-l. Zhuang, X. Zhong, S. W. Deng and J. G. Wang, Chem. Eng. J., 2021, 408, 127358 CrossRef CAS.
- S. S. Sonu, V. Dutta, P. Raizada, A. Hosseini-Bandegharaei, V. Thakur, V. H. Nguyen, Q. VanLe and P. Singh, J. Environ. Chem. Eng., 2021, 9, 105812 CrossRef CAS.
- H. Tang, R. Li, X. Fan, Y. Xu, H. Lin and H. Zhang, J. Environ. Chem. Eng., 2022, 10, 107797 CrossRef CAS.
- A. Behera and K. Parida, Mater. Today Proc., 2021, 35, 203–206 CrossRef CAS.
- C. Zheng, C. Zhang, K. Zhang, J. Zhang, L. Jin, A. M. Asiri, K. A. Alamry, L. He and X. Chu, Sens. Actuators, B, 2021, 330, 129280 CrossRef CAS.
- J. Ding, W. Xu, S. Liu, Y. Liu, X. Tan, X. Li, Z. Li, P. Zhang, L. Du and M. Li, J. Colloid Interface Sci., 2021, 588, 776–786 CrossRef CAS PubMed.
- L. Chen, X. Jiang, R. Xie, Y. Zhang, Y. Jin and W. Jiang, Sep. Purif. Technol., 2020, 250, 117232 CrossRef CAS.
- Y. Zhao, X. Yuan, X. Li, L. Jiang and H. Wang, J. Hazard. Mater., 2021, 409, 124893 CrossRef CAS PubMed.
- X. Fan, H. Lin, J. Zhao, Y. Mao, J. Zhang and H. Zhang, Sep. Purif. Technol., 2021, 272, 118909 CrossRef CAS.
- S. M. Teli and C. S. Mathpati, Chin. J. Chem. Eng., 2021, 32, 39–60 CrossRef CAS.
- J. Tao, J. Huang, S. Geng, F. Gao, T. He and Q. Huang, Chem. Eng. J., 2020, 386, 122769 CrossRef CAS.
- A. Makofane, D. E. Motaung and N. C. Hintsho-Mbita, Ceram. Int., 2021, 47, 22615–22626 CrossRef CAS.
- X. Rong, H. Chen, J. Rong, X. Zhang, J. Wei, S. Liu, X. Zhou, J. Xu, F. Qiu and Z. Wu, Chem. Eng. J., 2019, 371, 286–293 CrossRef CAS.
- Y. Wang, R. Priambodo, H. Zhang and Y.-H. Huang, RSC Adv., 2015, 5, 45276–45283 RSC.
- A. J. Santos, I. Sirés and E. Brillas, Chemosphere, 2021, 263, 128271 CrossRef PubMed.
- X. Zhu, C. Cao, S. Su, A. Xia, H. Zhang, H. Li, Z. Liu and C. Jin, Ceram. Int., 2021, 47, 15173–15179 CrossRef CAS.
- A. S. Basaleh, J. Mater. Res. Technol., 2021, 11, 1260–1271 CrossRef CAS.
- S. Li, Y. Zhang, L. Han, X. Li and Y. Xu, Sens. Actuators, B, 2021, 344, 130251 CrossRef CAS.
- S. Krishnan, S. Murugesan, V. Vasanthakumar, A. Priyadharsan, M. Alsawalha, T. Alomayri and B. Yuan, Colloids Surf., A, 2021, 611, 125835 CrossRef CAS.
- K. K. Das, L. Paramanik and K. Parida, Int. J. Hydrogen Energy, 2021, 46, 24484–24500 CrossRef CAS.
- F. G. El Desouky, M. M. Saadeldin, M. A. Mahdy and I. K. El Zawawi, Vacuum, 2021, 185, 110003 CrossRef CAS.
- R. Li, H. Hu, Y. Ma, X. Liu, L. Zhang, S. Zhou, B. Deng, H. Lin and H. Zhang, J. Clean. Prod., 2020, 276, 124246 CrossRef CAS.
- W. V. Steele and E. H. Appelman, J. Chem. Thermodyn., 1982, 14, 337–344 CrossRef CAS.
- G. Zeng, R. Yang, X. Fu, Z. Zhou, Z. Xu, Z. Zhou, Z. Qiu, Q. Sui and S. Lyu, Sep. Purif. Technol., 2021, 264, 118441 CrossRef CAS.
- K. Zhang, P. Sun, A. Khan and Y. Zhang, Sci. Total Environ., 2021, 765, 144630 CrossRef CAS PubMed.
- N. Welter, J. Leichtweis, S. Silvestri, P. I. Z. Sánchez, A. C. C. Mejía and E. Carissimi, J. Alloys Compd., 2022, 901, 163758 CrossRef CAS.
- S. Renukadevi and A. Pricilla Jeyakumari, Inorg. Chem. Commun., 2020, 118, 108047 CrossRef CAS.
- A. Khan, K. Zhang, A. K. A. Taraqqi, X. Wang, Y. Chen and Y. Zhang, J. Colloid Interface Sci., 2021, 599, 805–818 CrossRef CAS PubMed.
- Y. Wang, L. Ding, C. Liu, Y. Lu, Q. Wu, C. Wang and Q. Hu, Sep. Purif. Technol., 2022, 283, 120164 CrossRef CAS.
- K. Wang, S. Zhan, D. Zhang, H. Sun, X. Jin and J. Wang, Colloids Surf., A, 2021, 618, 126362 CrossRef CAS.
- B. Zhu, H. Cheng, J. Ma, Y. Kong and S. Komarneni, Chemosphere, 2019, 237, 124547 CrossRef CAS PubMed.
- Z. Feng, B. Zhou, R. Yuan, H. Li, P. He, F. Wang, Z. Chen and H. Chen, Chem. Eng. J., 2022, 440, 135669 CrossRef CAS.
- Q. Fang, S. Ye, H. Yang, K. Yang, J. Zhou, Y. Gao, Q. Lin, X. Tan and Z. Yang, J. Hazard. Mater., 2021, 420, 126569 CrossRef CAS PubMed.
- H. Luo, H. Fu, H. Yin and Q. Lin, J. Hazard. Mater., 2022, 426, 128044 CrossRef CAS PubMed.
- K. Xu, L. Jiao, C. Wang, Y. Bu, Y. Tang, L. Qiu, Q. Zhang and L. Wang, J. Environ. Sci., 2022, 111, 93–103 CrossRef PubMed.
- H. Peng, Y. Li, J. Wen and X. Zheng, Ind. Crops Prod., 2021, 172, 114066 CrossRef CAS.
- K. Zhu, Q. Bin, Y. Shen, J. Huang, D. He and W. Chen, Chem. Eng. J., 2020, 402, 126090 CrossRef CAS.
- L. Guo, J. Zhao, L. Zhao, Y. Tang, J. Zhou and B. Shi, Chem. Eng. J., 2021, 420, 127698 CrossRef CAS.
- E. Evgenidou, Z. Chatzisalata, A. Tsevis, K. Bourikas, P. Torounidou, D. Sergelidis, A. Koltsakidou and D. A. Lambropoulou, J. Environ. Chem. Eng., 2021, 9, 105295 CrossRef CAS.
- H. B. Truong, B. T. Huy, S. K. Ray, G. Gyawali, Y. I. Lee, J. Cho and J. Hur, Chemosphere, 2022, 299, 134320 CrossRef CAS PubMed.
- Y. Sun, R. Xiong, J. Zhang, Y. Ma, Y. Li, W. Ji, Y. Ma and Z. Wang, Sep. Purif. Technol., 2022, 293, 121066 CrossRef CAS.
|
This journal is © The Royal Society of Chemistry 2022 |
Click here to see how this site uses Cookies. View our privacy policy here.