DOI:
10.1039/D2RA03692D
(Paper)
RSC Adv., 2022,
12, 21135-21144
Intermolecular hydrogen bonds between catechin and theanine in tea: slow release of the antioxidant capacity by a synergetic effect†
Received
15th June 2022
, Accepted 14th July 2022
First published on 1st August 2022
Abstract
The health benefits of drinking tea stem from it being rich in polyphenols and other physiologically-active substances. Thus, exploring the synergistic effect between polyphenols and a variety of physiologically-active substances can contribute to our understanding of how tea benefits health. In this work, we have studied the interactions between catechin and theanine, exploring the synergetic antioxidant mechanism of the two molecules. Electrochemical characterization results showed that the oxidation peak current of catechin decreased gradually with the concentration of theanine, which is due to theanine spontaneously binding to catechin through intermolecular hydrogen bonds and forming molecular clusters via two hydrogen bonds. The binding constant is 4.75 at room temperature. The molecular clusters reduce the diffusion coefficient of catechin in solution, leading to the slow release of its antioxidant capacity (ability to effectively inhibit free radical oxidation reactions). Density functional theory calculations were also performed and verified the binding behavior. In identifying the synergistic effect between catechin and theanine on the antioxidant capacity of tea, this study adds to our understanding of the efficacy of tea polyphenols.
1. Introduction
Tea is one of the most popular water-based drinks and is loved by consumers for its refreshing, anti-aging, anti-cancer and other functions.1 The health benefits of tea have been extensively studied in recent years.2 Increasingly, researchers are focusing on the link between tea consumption and human cancer incidence, and the potential of tea or tea polyphenols to prevent or improve chronic diseases is also the subject of quite a few scientific studies.3 Many laboratories have shown that tea has a certain inhibitory effect on tumor formation and growth, and this inhibitory activity is believed to be closely related to the antioxidant capacity of tea polyphenols.4
The health benefits of tea are mainly due to the fact that it contains a large number of physiologically active ingredients, such as tea polyphenols, theanine, and caffeine.5,6 Tea polyphenols are one of the most important physiologically active substances in tea,7 it has unique pharmacological properties to prevent cancer, cardiovascular disease, neurodegenerative diseases and other diseases,8 studies have shown that in neurodegenerative diseases, oxidative stress in Alzheimer's disease is its underlying pathological mechanism, possibly due to an imbalance of reactive oxygen species and antioxidant molecules, and the antioxidant properties of catechin can be relieved to a certain extent.8 In addition, catechin inhibits tumor growth and carcinogenesis, as well as the release of tumor necrosis factor, due to its antioxidant properties, can play a protective role in low density lipoprotein antioxidant and anti-atherosclerosis.9 Theanine is a unique non-protein amino acid that accounts for about 50% of the amino acids in tea leaves and is one of the main components that form the flavor of tea soup. Theanine can not only be used as an additive, but also has a variety of physiological functions, which can directly affect the central nervous system, regulate mood, memory ability and so on.10–12 At the same time, its pharmacological effects have shown that theanine can improve the antioxidant capacity of liver cells and prevent liver damage caused by ethanol.13 It also has an antitumor and antihypertensive effect, which can reduce the oxidation of low-density lipoprotein and prevent atherosclerosis like theaflavins. Since the positive effects of tea on health stem from its high content of chemicals with antioxidant capacity,14 the beneficial effects of these substances on health and their analysis have been the focus of the scientific community.15,16
In recent years, researchers devote to research that the antioxidant activities and synergistic effects of polyphenols, Erskine et al.17 revealed synergistic and antagonistic effects of phenols between different components of coffee and food substrates. Hajimehdipoor et al.18 proved that gallic acid and caffeic acid had strong synergistic effect by FRAP (ferric reducing antioxidant power) method. Catechin are one of the polyphenols in tea, both inside and outside the body are a powerful antioxidant, and when there are certain minerals and vitamins, it can improve antioxidant capacity which makes it a hot research topic. Therefore, some scholars used the Co(II)–EDTA luminol chemiluminescence to verify that the increase in ascorbic acid content has a positive synergistic effect on the antioxidant capacity of catechin.19 At the same time, according to reports, catechin and theanine have a synergistic effect in regulating the body's nutrient metabolism and repairing oxidative damage in cells.20 However, there has been little research on the effects of the interaction of various ingredients in tea on the antioxidant capacity and the mechanism of their action, the traditional in vitro antioxidant detection method uses a UV-spectrophotometer to detect changes in the content of various color components, but this will be affected by the color and turbidity of the sample itself, so that the result is a large error, while electrochemical sensors have been widely used in food detection because of their high sensitivity, good selectivity, low cost, and fast analysis speed.21–23 Therefore, the use of electrochemical technology to explore the synergistic effect of theanine on the antioxidant capacity of catechin can not only allow consumers to clear the mechanism of action to understand the benefits of drinking tea, but also promote the development of the food industry.
In this paper, the effect of theanine in tea on the antioxidant capacity of catechin was studied by electrochemical techniques. Electrochemical results showed that catechin spontaneously bind to theanine through two hydrogen bonds in aqueous solutions and slowly release the antioxidant capacity to a certain extent. Density functional theory theoretically provides a good explanation for the mechanism.
2. Experiments
2.1 Chemicals
(+)-Catechin hydrate (95%), theanine (98%) and ferrocenemethanol (95%) were purchased from Aladdin, Shanghai. 2-Mercaptoethanol (99%) was purchased from Energy Chemical. DPPH (2,2-diphenyl-1-picrylhydrazyl (98.5%)) was purchased from Macklin, Shanghai. Potassium phosphate monobasic (99.5%), sodium phosphate dibasic dodecahydrate (99%), potassium hexacyanoferrate (99.5%) and potassium ferrocyanide trihydrate (99.5%) were purchased from Peagent. Sodium hydroxide (99%), hydrochloric acid (37%), ethanol (99.7%), hydrogen peroxide (30%) were purchased from Chuandong Chemical Plant, Chongqin.
2.2 Electrochemical experiments
Platinum ultra-micro working electrodes (radius 5 μm), Ag/AgCl (3 M KCl) reference electrodes, gold working electrodes, platinum wire counter electrodes were purchased from CH Instruments, Inc. The scanning electrochemical microscopy (CHI 900D) was employed to conduct all electrochemical experiments. The potentials in all experiments referred to the Ag/AgCl (3 M KCl) reference electrode.
The cyclic voltammograms of 10 mM catechin in the absence of L-theanine and presence of 10 mM theanine were conducted in a water solution of 0.1 M PBS on gold electrode at pH 1.79, 2.87, 3.40, 4.75, 5.87, 6.65, 7.26, respectively, scanning from 0 to 0.8 V with a scan rate of 0.1 V s−1. The differential pulse voltammograms of 10 mM catechin were conducted on gold electrode in the presence of 17 mM, 34 mM, 51 mM, 68 mM, 85 mM, 102 mM, 119 mM, 136 mM, 153 mM theanine, respectively.
The scanning electrochemical microscopy images of the mercaptoethanol self-assembled monolayers in the absence and presence of catechin and theanine were conducted in a solution of 1.0 mM ferrocenemethanol. The tip electrode was biased with 0.4 V, the tip to substrate distance was set 0.5 d/a, the scan rate was set 1 μm s−1 in the range of 10 μm × 10 μm.
2.3 Preparation of self-assembled monolayers
Firstly, gold working electrode was polished by alumina powder for 30 min. Later, the press polished electrode was treated by sonic leaning. The clean electrode was immersed in 0.2 M H2SO4 and reactivated by cyclic voltammetry, where the potential was set from 0 to 1.6 V (scan rate: 0.1 V s−1). Lastly, the reactive gold surface was immersed 0.1 M 2-mercaptoethanol for 6 hours to gain the self-assembled monolayers.
2.4 Theory calculations
All the density functional theory (DFT) calculations were performed with the Gaussian 16 program with the supercomputer in Liaoning Shihua University. The calculations were performed with the B3LYP method, 6-311+g(d,p) basis and SMD solvent model. The ground states of catechin and catechin–theanine clusters were optimized in the water environment. Frequency calculations were also performed to make sure that the geometries of the ground-state reached the minimum point on the potential energy surfaces. All the computations were performed without symmetry constraints.
3. Results and discussion
3.1 The effect of theanine on the catechin antioxidant capacity
First, the effect of theanine on the electrochemical behavior of catechin was determined. Fig. 1(a) showed the results of differential pulse voltammetry measurements performed on mixed solutions of catechin and theanine (in equimolar amounts) with increasing concentration. As the concentration increases, the peak potential remains essentially the same, while the peak current undergoes a marked increase. As Fig. 1(b) shown, the oxidation peak current value was linearly related to the concentration of the two compounds, indicating that the change in the oxidation reaction was caused by the change in the concentration, and the change was the amount of oxidation rather than the degree. Fig. 1(c) showed the results of differential pulse voltammetry measurements of an increasing concentration of theanine in a 10 mM catechin solution. Initially, the oxidation peak current of catechin decreased as the theanine concentration increased, then it stabilized. Whereas, the peak potential did not change significantly. This may be evidence of a slow release of catechin antioxidant capacity caused by the presence of theanine. In addition, the redox behavior of catechin and theanine were discussed separately. Fig. S1(a) and (b)† showed differential pulse voltammograms and cyclic voltammograms of blank solution, catechin solution, and theanine solution, respectively. It can be seen from the Fig. S1(a)† that catechin had a significant oxidation peak, theanine doesn't had an oxidation peak. In Fig. S1(b),† catechin showed a significant redox peak, while theanine does not had a redox peak, which indicates that catechin has a good redox ability, while theanine itself does not have this ability.
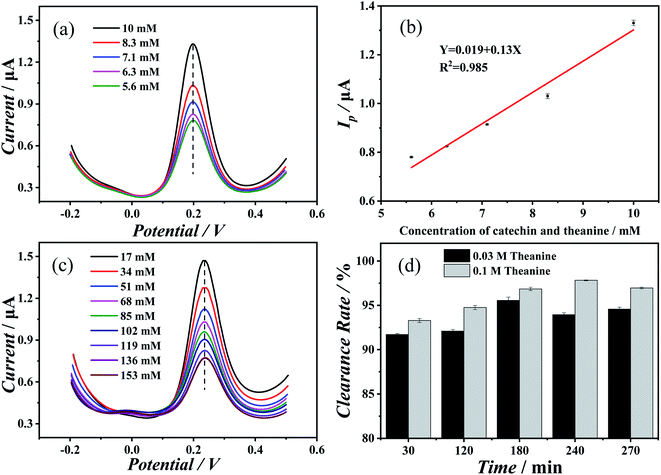 |
| Fig. 1 (a) Differential pulse voltammograms of different concentrations of catechin and theanine (catechin and theanine were equal) at pH 6.65. (b) Plot of oxidation peak current of catechin and L-theanine concentrations. (c) Differential pulse voltammetry of catechin in solutions with different theanine concentrations at pH 6.65. (d) Plot of superoxide radical clearance of 0.03 M and 0.1 M theanine in catechin versus time. (Catechin concentration: 6.8 mM.) | |
To verify the sustained release of catechin due to the presence of the theanine, we prepared a catechin–theanine solution to remove superoxide free radicals in a DPPH solution. In this experiment, 5 mL of DPPH solution (8 mmol L−1) was added to a 5 mL catechin solution containing different concentrations of theanine (the catechin concentration remains constant). UV-Vis spectroscopy was performed on three solutions in parallel, measuring the absorbance at 510 nm.24,25 The clearance of DPPH could then be calculated as follows:
|
(DPPH)% = (A0 − A2 + A1)/A0 × 100%
| (1) |
where
A0,
A1, and
A2 are the absorbances of the blank solution, the sample replaced by ethanol, and of the sample after the addition of a catechin solution containing theanine, respectively.
Fig. 1(d) showed the change in the scavenging ability of catechin solutions containing different concentrations of theanine to superoxide radicals over time. As can be seen, the clearance of superoxide radicals gradually increased over time, with the solution with 0.1 M theanine having the greatest clearance at 250 min. However, the peak clearance of the solution containing 0.03 M theanine occurred at 190 min, which shows that catechin was released more slowly in the solution with 0.1 M theanine than in the solution with 0.03 M theanine. In other words, as the concentration of theanine increased, the rate at which the catechin antioxidant capacity was released decreased, which is consistent with the results of the electrochemical experiments.
3.2 The slow-release mechanism of catechin antioxidant capacity
Fig. 2(a) showed the results of cyclic voltammetry measurement with an aqueous solution containing 10 mM of both catechin and theanine at a pH of 1.77, 1.79, 3.40, 4.75, 5.87, 6.65, and 7.26. As the concentration of protons in the solution decreases, the oxidation peak potential and the reduction peak potential move in the negative direction. This negative shift of the oxidation peak showed that the oxidation process was more likely to occur, which indicated that catechin can more easily provide electrons to prevent the oxidation of other substances. While conversely, the negative shift of the reduction peak indicates that reduction is less likely to occur. Therefore, in the case of catechin in solutions containing theanine, as the proton concentration decreases, the antioxidant capacity increases. Fig. 2(b) and (c) showed the relationship between the pH and the oxidation peak potential and the reduction peak potential, respectively. As can be seen, both potentials showed a good linear relationship with the pH, and the slopes were −0.056 and −0.052, respectively. According to the Nernst equation:26 |
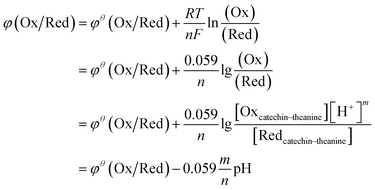 | (2) |
where φθ(Ox/Red) is the standard electrode potential, R is the gas constant, T is the temperature, n is the number of electron transfers during the electrode reaction, F is Faraday's constant, Oxcatechin–theanine is the amount of catechin oxidation in the theanine solution, and Redcatechin–theanine is the amount of catechin reduction in the theanine solution. With the addition of protons in the solution, both the oxidation peak potential and the reduction peak potential have slopes of close to −0.059, which means that m/n in eqn (2) was close to 1, and, thus, the redox that occurs in the solution released an equal amount of electrons and protons. As Fig. 2(d) showed, the ratio of the redox current, which was an important basis for judging whether an electrochemical reaction was reversible, was also close to 1. As the closer the ratio of the two was to 1, the higher the degree of reversibility, the redox reaction of catechin in the theanine solution was quasi-reversible.
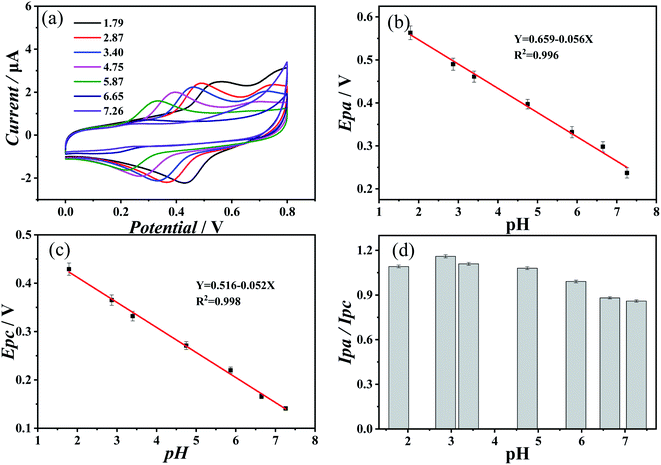 |
| Fig. 2 (a) Cyclic voltammograms of 10 mM catechin and theanine in aqueous solution on gold electrode at pH 1.77, 1.79, 3.40, 4.75, 5.87, 6.65, 7.26. Scan rate: 0.1 V s−1. (b) Plot of peak oxidation potential versus pH. (c) Diagram of the reduced peak potential versus pH. (d) Ratio plot of oxidation peak current to reduction peak current to pH. | |
Fig. 3(a) showed the cyclic voltammogram of 10 mM catechin at a range of pH in the absence of theanine. The redox process of catechin in water was reversible, and its redox capacity was closely related to 3′-OH and 4′-OH on the B ring,27 as shown in the molecular structural formula of the catechin in Fig. 3(c). The first step of the oxidation of catechin occurred on the B ring of the o-phenol hydroxyl group, and the corresponding o-quinone structure was generated through 2e−/2H+ reaction,28,29 followed by the second step of oxidation of 5-OH and 7-OH on the A ring.27,30,31 Fig. 3(b) showed a comparison of the oxidation peak potential of catechin solution with and without theanine. The presence of theanine does not significantly change the oxidation potential of the catechin, which was consistent with the previous conclusion. This suggests that theanine did not interact with the o-hydroxyl group of catechin.
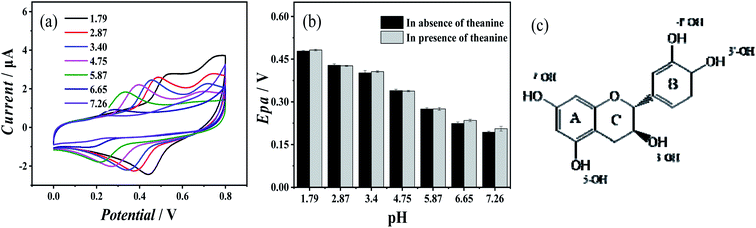 |
| Fig. 3 (a) Cyclic voltammograms of 10 mM catechin in aqueous solution on a gold electrode at pH values of 1.79, 2.87, 3.40, 4.75, 5.87, 6.87, 6.65 and 7.26, respectively – the scan rate was 0.1 V s−1. (b) Oxidation potential of an aqueous catechin solution without and with theanine. (c) The molecular structure of catechin. | |
Cyclic voltammetry was also used to study the electrochemical behavior of 10 mM catechin in the presence of different concentrations of theanine. The results were consistent with those of the differential pulse voltammetry. The oxidation peak current of catechin gradually decreased with increasing theanine concentration. The oxidation peak current could be calculated as follows:32
|
Ipa = (2.69 × 105)n3/2SD1/2Cv1/2
| (3) |
where
Ipa is the oxidation peak current,
n is the number of electron transfers,
S is the electrode area,
D is the diffusion coefficient,
C is the concentration of the redox agent, and
v is the scanning rate.
33 This allows the diffusion coefficient of catechin to be calculated. The results of those calculations were shown in
Table 1. The diffusion coefficient of catechin gradually decreased with increasing theanine concentration which might be the hydrogen bonding between catechin and theanine. The formation of molecular clusters would reduce the diffusion coefficient of the catechin in the solution, resulting in a slower release.
Table 1 The diffusion coefficient of catechin at different theanine concentrations
Concentration of theanine |
17 mM |
34 mM |
51 mM |
68 mM |
85 mM |
102 mM |
119 mM |
136 mM |
Ip/μA |
1.06 |
0.94 |
0.86 |
0.79 |
0.72 |
0.68 |
0.65 |
0.59 |
D/10−10 cm2 s−1 |
1.84 |
1.52 |
1.27 |
1.07 |
0.90 |
0.80 |
0.73 |
0.61 |
The experiments detailed above had verified that theanine would slow the release of catechin's antioxidant. We can use these conclusions to arrive at the mechanism of the action of theanine on catechin. Structurally, catechin contains five phenolic hydroxyl groups. It is the ortho-phenolic hydroxyl groups that give catechin its strong antioxidant capacity, the redox capacity of meso-phenolic hydroxyl being not as significant as that of ortho-phenolic hydroxyl groups.34,35 Theanine is composed of one molecule of glutamate and one molecule of acetyl joined by peptide bonds formed by shrinkage. Phenolic hydroxyl group was weak in acidity and proton giving ability, and will decompose mostly in alkaline environment. However, our experiment was carried out in neutral environment, which was not conducive to the proton giving by phenolic hydroxyl group, at the same time, the amino group was easy to obtain protons in acidic environment, so it was difficult for the amino group to obtain protons in neutral environment. Therefore, it could be judged that the existence of ion pair effect was very weak in this study. It was suggested that the resorcinol of the catechin formed a strong polar bond with an oxygen atom and nitrogen atom on theanine and the molecular clusters reduce the diffusion coefficient of catechin in the solution, resulting in the continuous release of the catechin's antioxidant capacity. This hypothesis was consistent with the results of this study. The mechanism was summarized in Fig. 4.
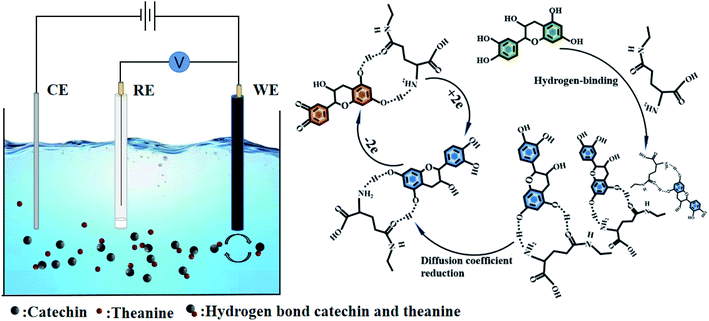 |
| Fig. 4 Mechanism of interaction between catechin and theanine. | |
3.3 Adsorption behavior of catechin and theanine in the presence of hydrogen bonds
Scanning electrochemical microscopy experiments were adopted to verify the hypothetical mechanism of action of catechin and theanine. A gold electrode coated with 2-mercaptoethanol, which was a short-chain thiol that both increases the electron transport rate, promoting the redox reaction of electron transport, and contains a large number of exposed hydroxyl groups, was used in the experiments.36,37 Ferrocenemethanol was used as an electrochemical tag to detect the electrochemical behavior with and without catechin and theanine present. Ferrocenemethanol will undergo an oxidation reaction near the tip and then diffuse to the gold electrode. When the solution contains catechin and theanine, the hydroxyl groups on the 2-mercaptoethanol assembled on the gold electrode will promote the formation of hydrogen bonds between catechin and theanine, thereby reducing the diffusion coefficient of the solution, which, in turn, will lead to a decrease in the tip feedback current. The experiment was summarized in Fig. 5(a). The electrochemical impedance spectrogram (Fig. 5(b)) showed that, as the theanine concentration increases, the charge transfer impedance also increases (10 mM theanine (420 Ohms), 5 mM theanine (450 Ohms), 10 mM theanine (470 Ohms), 15 mM theanine (500 Ohms)). This may be due to the decrease in electron transfer rate caused by the formation of hydrogen bonds with hydroxyl groups by more and more theanine molecules.38 Fig. 5(c) showed a scanning electrochemical micrograph of the ferrocenemethanol only, showing that the tip feedback current varied between 2.18 nA and 2.17 nA. Fig. 5(d) showed the same results with catechin and theanine added. The feedback current has dropped to between 1.23 nA and 1.10 nA, which shows that the molecular clusters formed by the hydrogen bonds between the theanine and hydroxyl groups in the solution reduce the diffusion coefficient, resulting in a decrease in the current.
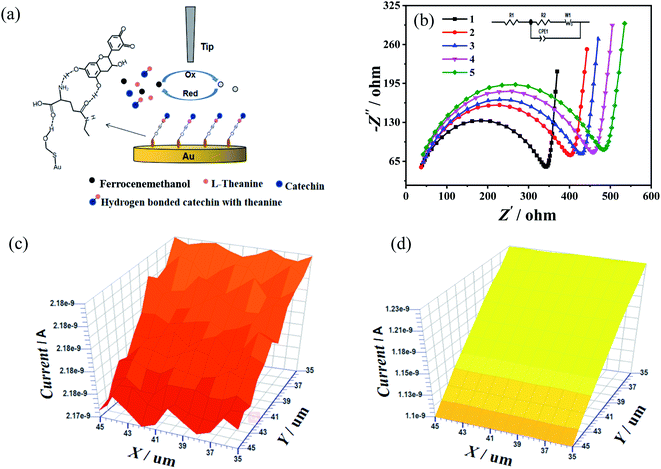 |
| Fig. 5 (a) Scanning electrochemical microscopy to verify the protocol of thiol to promote the formation of hydrogen bonds between catechin and theanine. (b) Electrochemical impedance spectra: (1) 1.0 mM [Fe(CN)6]3−/4−. (2) 1.0 mM [Fe(CN)6]3−/4− and 10 mM catechin. (3) 1.0 mM [Fe(CN)]63−/4−, 10 mM catechin and 5 mM theanine. (4) 1.0 mM [Fe(CN)6]3−/4−, 10 mM catechin and 10 mM theanine. (5) 1.0 mM [Fe(CN)6]3−/4−, 10 mM catechin and 15 mM theanine are self-assembled in single-layer electrodes in 2-mercaptoethanol, respectively. (c) Scanning electrochemical microscopy image of 2-mercaptoethanol self-assembled monolayers in the absence of catechin and theanine. (d) Scanning electrochemical microscopy image of 2-mercaptoethanol self-assembled monolayers in the presence of 10 mM catechin and theanine. (Tip-to-substrate distance, 0.5 d/a; ET = 0.4 V; scan rate, 1 μm s−1 in the range of 10 μm × 10 μm.) | |
3.4 Thermodynamic parameters and the interactions between catechin and theanine
It can be seen from the above experiments that theanine can slow down the release of catechin and, thereby, prolong its antioxidant capacity. The effect of theanine on catechin can be quantified by measuring the binding constants at two different temperatures (284 K and 294 K). Assuming that catechin and theanine only form a simple complex and calculating the binding rate and binding constant of the two according to approaches given in the literature, the binding reaction can be summarized as follows:39,40
Catechin + mA ↔ catechin − mA |
β = [catechin − mA]/[catechin][A]m |
The peak oxidation current is related to the binding constant, β, and the binding number m, according to the formula:
|
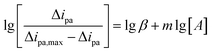 | (4) |
|
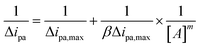 | (5) |
where Δ
ipa,max is the maximum difference in oxidation peak current before and after the addition of theanine, and A is the catechin concentration. Thus, if catechin and theanine only form a simple complex, then the difference in oxidation peak current in the presence of theanine in the catechin and the absence of theanine should be linearly related to the logarithmic relationship curve of the change in catechin concentration, and the binding constant, and binding number, can be obtained from the intercept and slope of the straight line, respectively. The main types of non-covalent bonds are hydrogen bonds, hydrophobic interactions, electrostatic interactions, and van der Waals forces. The parameters of the thermodynamic binding reactions, including Gibbs free energy, enthalpy, and entropy, can be used to elucidate the types of interaction between matter, according to the van't Hoff equation:
|
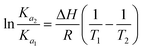 | (6) |
|
ΔG = −RT ln Ka = ΔH − TΔS
| (7) |
where
Ka1 and
Ka2 are the correlation constants at temperature
T1 and
T2,
R is the general gas constant, the symbols and magnitude of the thermodynamic parameters are related to various possible interactions, namely Δ
H > 0, Δ
S > 0, the main reason for these changes is the hydrophobic force between the substances, when Δ
H > 0, when Δ
S < 0, van der Waals force and hydrogen bond play the main role. When Δ
H < 0, Δ
S > 0, electrostatic interaction force was the main force.
41 As
Fig. 6(a) was at 284 K and
Fig. 6(c) at 294 K show, there is a good linear relationship between the oxidation peak current of catechin and the change of catechin concentration, both with and without theanine in the solution. The binding constant between catechin and theanine was found to be 1.6 at 284 K (
Fig. 6(b)) and 4.75 at 294 K (
Fig. 6(d)), from
eqn (6) and
(7), Δ
G was calculated to be <0, indicating that catechin and theanine can bind spontaneously by hydrogen bonds. Comparing to other literature, Hayashi
et al.42 determined binding energy of catechin/caffeine complexes in water solution by titration experiments with
1H-NMR. The binding energy of catechin and caffeine was 8.37 kJ mol
−1 at 301 K, so the binding constant was obtained to be 28.2. Therefore, our result was reasonable.
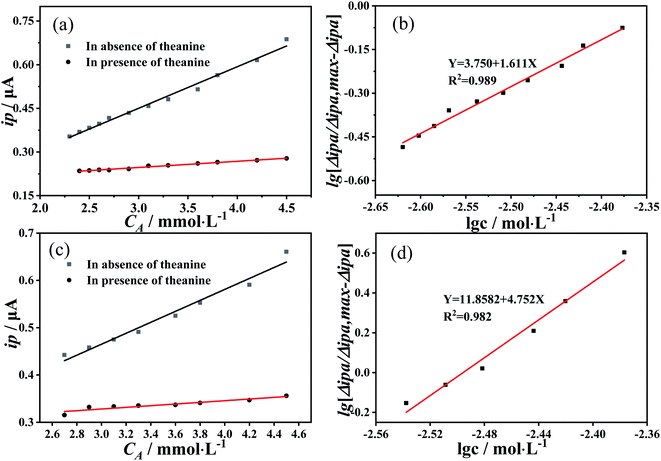 |
| Fig. 6 (a) Relationship between the oxidation peak current and catechin concentration in the presence and absence of catechin in catechin at 284 K. (b) Logarithmic relationship between the difference in the oxidation peak current in the presence of theanine in catechin and the absence of theanine at 284 K and the change in catechin concentration. (c) Relationship between the oxidation peak current and catechin concentration in the presence and absence of catechin at 294 K. (d) Logarithmic relationship between the difference in oxidation peak current and the change in catechin concentration at 294 K in the presence of theanine and the absence of theanine. | |
3.5 DFT calculations
Expect for the experimental investigation on the hydrogen bonds between catechin and theanine, the DFT calculations were also employed to further inspect the molecular interactions. The calculations were performed with the B3LYP method, 6-311+g(d,p) basis and SMD solvent model, by Gaussian 16 program. The ground states of catechin and catechin–theanine cluster were optimized in the water environment. Frequency calculations were also performed to make sure that the geometries of the ground-state reached the minimum point on the potential energy surfaces. The optimized geometries were shown in Fig. 7, the structure (a) was the ground state of catechin, (b) was the ground state of catechin–theanine cluster. It could be seen that the 5-OH and 7-OH on the catechin was hydrogen bonded with N and O atoms on theanine, respectively. The hydrogen bonds lengths were obtained to be 1.86635 Å and 2.00326 Å, for O⋯HO and N⋯HO, respectively. In addition, the electron energies for each structure were also calculated, shown in Fig. 7. Thus, the two hydrogen bonds energy could be obtained to be −82.67 kJ mol−1 in total. It could be regarded as the strong hydrogen bonds, according to the bond length and bond energy. Fig. 8 showed the HOMO–LUMO plots of catechin and the catechin–theanine cluster, respectively. The HOMO energy of catechin, as well as LUMO energy, exhibited a slight decrease after the hydrogen bonds formed. The energy gap of catechin in water environment was found to be 5.484 eV. And the energy gap of the catechin–theanine cluster in water environment was found to be 5.418 eV. The two energy gap values were very close, indicating an equivalent energy barrier for electron transition. This result was in accord with the little electrochemical peak displacement in Fig. 3(b).
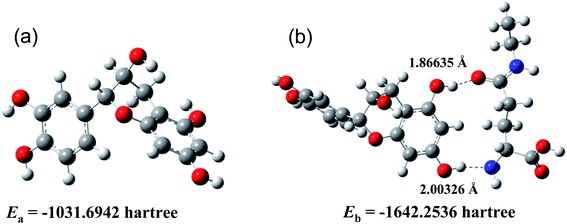 |
| Fig. 7 Optimized geometries of catechin (a) and catechin–theanine cluster (b). | |
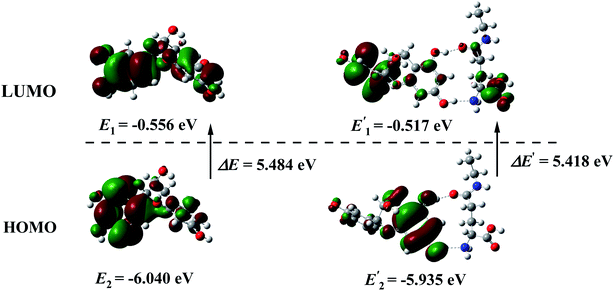 |
| Fig. 8 HOMO–LUMO plots of catechin and the catechin–theanine cluster, respectively. | |
Lastly, the object of this study was a match-free solution based on catechin and theanine in tea, which avoids the interference of other substances, magnifies the experimental phenomenon, and can more intuitively and clearly reflect the electrochemical phenomenon of the reaction between catechin and theanine. Moreover, the mechanism of double hydrogen bond formation and theanine slowing down the release of catechin's antioxidant capacity can also be applied to real tea samples.
4. Conclusion
Electrochemical experiments and radicals scavenging experiments were used to study the synergistic effect of theanine on the antioxidant capacity of catechin in tea. The results showed that theanine and catechin spontaneously combined through two hydrogen bonding and the presence of theanine promoted the slow release of catechin's antioxidant capacity, which was attributed to the fact that the formed molecular clusters reduced the diffusion coefficient in solution. The above mechanism was well described by scanning electrochemical microscopy and DFT calculations. The results showed that the binding constant between catechin and theanine was 4.75 at room temperature. The bonds lengths were 1.86635 Å and 2.00326 Å for each hydrogen bond, respectively. And the two hydrogen bonds energy could be obtained to be −82.67 kJ mol−1 in total.
Author contributions
Yirong Xia: experiments, data curation, original draft preparation. Wei Ni: correction and revision. Xintong Wang: software. Yanyan Wang: reviewing and editing. Ximing Huang: conceptualization, methodology, validation.
Conflicts of interest
We declare that there is no conflict of interest including any financial, personal or other relationships with other people or organizations.
Acknowledgements
We gratefully acknowledge the financial support from National College Students' Innovation and Entrepreneurship Training Program (202110547021), Innovation Foundation for Postgraduate of Shaoyang University (CX2021SY070). We gratefully acknowledge the Density Functional Theory calculation supported by Liaoning Shihua University.
References
- Y. C. Liu, X. Y. Li and L. Shen, Appl. Microbiol. Biotechnol., 2020, 104, 981–987 CrossRef CAS PubMed.
- N. Khan and H. Mukhtar, Life Sci., 2007, 81, 519–533 CrossRef CAS PubMed.
- B. Frei and J. V. Higdon, J. Nutr., 2003, 133, 3275S–3284S CrossRef CAS PubMed.
- C. S. Yang and Z. Y. Wang, JNCI, J. Natl. Cancer Inst., 1993, 85, 1038–1049 CrossRef CAS PubMed.
- Y. Li, S. Zhang and Y. Sun, Saudi J. Biol. Sci., 2020, 27, 214–221 CrossRef CAS PubMed.
- R. Song, D. Kelman, K. L. Johns and A. D. Wright, Food Chem., 2012, 133, 707–714 CrossRef CAS.
- X. Y. Zhao, H. D. Xu and R. X. Yang, Food Sci., 2014, 35, 169–175 CAS.
- M. Isemura, Molecules, 2019, 24, 528 CrossRef PubMed.
- H. M. Princen, D. W. Van, R. Buytenhek, C. Blonk, L. B. Tijbury, J. A. Langius, A. E. Meinders and H. Pijl, Arterioscler., Thromb., Vasc. Biol., 1998, 18, 833–841 CrossRef CAS PubMed.
- A. C. Nobre, A. Rao and G. N. Owen, Asia Pac. J. Clin. Nutr., 2008, 17, 167–168 CAS.
- L. R. Juneja, D. C. Chu, T. Okubo, Y. Nagato and H. Yokogoshi, Trends Food Sci. Technol., 1999, 10, 199–204 CrossRef CAS.
- C. F. Haskell, D. O. Kennedy, A. L. Milne, K. A. Wesnes and A. B. Scholey, Biol. Psychol., 2008, 77, 113–122 CrossRef PubMed.
- Y. Liu, S. Liu and C. L. Wang, Food Res. Dev., 2016, 37, 211–214 CrossRef CAS PubMed.
- J. P. Zegarac, V. Lidija, T. Stipcevic and S. Martinez, Food Chem., 2010, 121, 820–825 CrossRef.
- S. U. Lule and W. S. Xia, Food Rev. Int., 2005, 21, 367–388 CrossRef.
- S. Coimbra, E. Castro, P. R. Pereira, I. Rebelo, S. Rocha and A. S. Silva, Clin. Nutr., 2006, 25, 790–796 CrossRef CAS PubMed.
- E. Erskine, B. G. Subasi, B. Vahapoglu and E. Capanoglu, ACS Omega, 2022, 7, 1595–1601 CrossRef CAS PubMed.
- H. Hajimehdipoor, R. Shahrestani and M. Shekarchi, Res. J. Pharmacogn., 2014, 1, 35–40 CAS.
- M. A. A. Mahmoud, V. S. Chedea, A. Detsi and P. Kefalas, Food Res. Int., 2013, 51, 907–913 CrossRef CAS.
- L. Liu, L. Zeng, A. Liu, Y. Q. Peng, D. Y. Yuan, S. Zhang, Y. H. Li, J. H. Chen, W. J. Xiao and Z. H. Gong, Food Funct., 2020, 11, 1798–1809 RSC.
- T. S. K. Sharma and K. Y. Hwa, Chem. Eng. J., 2022, 439, 135591 CrossRef CAS.
- T. S. K. Sharma and K. Y. Hwa, Inorg. Chem., 2021, 60, 6585–6599 CrossRef CAS PubMed.
- T. S. K. Sharma, K. Y. Hwa and A. Ganguly, Inorg. Chem. Front., 2020, 7, 1981–1994 RSC.
- T. Nagai, R. Inoue, H. Inoue and N. Suzuki, Nutr. Res., 2002, 22, 519–526 CrossRef CAS.
- K. Zhu, H. Zhou and H. Qian, Process Biochem., 2006, 41, 1296–1302 CrossRef CAS.
- S. Y. Tan, V. Y. Y. Chia, K. H. Otto and A. Franklin, J. Chem. Educ., 2020, 97, 2238–2243 CrossRef CAS.
- Y. R. Xia, X. T. Wang, H. C. Sun and X. M. Huang, RSC Adv., 2021, 11, 39985–39993 RSC.
- S. Martinez, L. Valek, Z. Petrovic, M. Metikos-Hukovic and J. Piljac, J. Electroanal. Chem., 2005, 584, 92–99 CrossRef CAS.
- H. Hotta, H. Sakamoto, S. Nagano, T. Osakai and Y. Tsujino, Biochim. Biophys. Acta, Gen. Subj., 2001, 1526, 159–167 CrossRef CAS.
- K. M. Bark, J. E. Yeom, J. I. Yang, I. J. Yang, C. H. Park and H. R. Park, Bull. Korean Chem. Soc., 2011, 32, 3443–3447 CrossRef CAS.
- M. Mochizuki, S. I. Yamazaki, K. Kano and T. Ikeda, Biochim. Biophys. Acta, Gen. Subj., 2002, 1569, 35–44 CrossRef CAS.
- T. Asakawa, H. Sunagawa and S. Miyagishi, Langmuir, 1998, 14, 7091–7094 CrossRef CAS.
- X. Yuan and N. Xu, J. Alloys Compd., 2001, 316, 113–117 CrossRef CAS.
- S. Steenken and P. Neta, J. Phys. Chem., 1982, 86, 3661–3667 CrossRef CAS.
- P. Janeiro and A. M. O. Brett, Anal. Chim. Acta, 2004, 518, 109–115 CrossRef CAS.
- S. Chattopadhyay, S. Bandyopadhyay and A. Dey, Inorg. Chem., 2021, 60, 597–605 CrossRef CAS PubMed.
- E. Hengge, M. Hirber, P. Brunner, E. M. Steyskal, B. Nidetzky and R. Wurschum, Phys. Chem. Chem. Phys., 2021, 23, 14457–14464 RSC.
- M. H. Hussin and M. J. Kassin, Int. J. Electrochem. Sci., 2011, 6, 1396–1414 CAS.
- W. Yang, E. Chow, G. D. Willett, D. B. Hibbert and J. J. Gooding, Analyst, 2003, 128, 712–718 RSC.
- B. Rafique, A. M. Khalid, K. Akhtar and A. Jabbar, Biosens. Bioelectron., 2013, 44, 21–26 CrossRef CAS PubMed.
- J. H. Wu, J. Y. Huo, M. Q. Huang, M. M. Zhao, X. L. Luo and B. G. Sun, J. Agric. Food Chem., 2017, 65, 10495–10504 CrossRef CAS PubMed.
- N. Hayashi, T. Ujihara and K. Kohata, Biosci., Biotechnol., Biochem., 2004, 68, 2512–2518 CrossRef CAS PubMed.
|
This journal is © The Royal Society of Chemistry 2022 |