DOI:
10.1039/D2RA03437A
(Paper)
RSC Adv., 2022,
12, 24130-24138
Anticancer and antibacterial flavonoids from the callus of Ampelopsis grossedentata; a new weapon to mitigate the proliferation of cancer cells and bacteria†
Received
2nd June 2022
, Accepted 17th August 2022
First published on 25th August 2022
Abstract
A new flavonoid angelioue (1) together with five known compounds cuminatanol (2), myricetin (3), epigallocatechin (4), taxifolin (5) and dihydromyricetin (6) was isolated from the callus extract of Ampelopsis grossedentata (Hand.-Mazz.) W. T. Wang and the structures were elucidated based on their detailed spectroscopic data. Among the compounds, the new compound angelioue (1) displayed significant antibacterial activity against methicillin-resistant Staphylococcus aureus (MRSA) with the MIC value of 6.68 μg mL−1 and MBC value of 53.42 μg mL−1; in contrast the other compounds showed moderate to no antibacterial activity. In addition, known dihydromyricetin (6) exhibited potent cytotoxic activities against mouse breast cancer cells (4T1), human lung adenocarcinoma (A549) and human non-small cell lung cancer (NCI-H1975) tumor cell lines with GI50 values of 17.47, 18.91 and 20.50 μM mL−1, respectively. The compounds 1–5 exhibited low micro-molar inhibitory activities. Moreover, the structure–activity relationships of the most active compounds for antibacterial and cytotoxic activities are discussed. The present findings clearly suggest that the A. grossedentata callus is a good source of bioactive compounds.
Introduction
Globally, serious infections by bacteria and the development of resistance due to genetic modification against currently available antibiotics kill around 700
000 patients each year. This is one of the biggest healthcare problems in the community and hospital settings.1,2 In addition to this, cancer still poses a serious threat to mankind and causes a huge economic burden, although numerous efforts have been made for its prevention and control. In the past year, according to the World Health Organization, 7.6 million deaths were recorded due to cancer worldwide. Globally, the deaths from cancer are projected to continue to increase to over 13.1 million in 2030.3,4 In order to overcome this serious global threat, there is an urgent need to find new drugs to effectively treat cancer and drug-resistant bacteria.
Natural compounds produced by plants are a major resource of antibacterial and anticancer drugs. In particular, flavonoids constitute one of the most precious and structurally diverse groups of natural compounds which have been shown to possess potent antibacterial activity against drug-resistant bacteria and anticancer activity with minimal toxicity against normal cells. Flavonoids are capable of exerting of antibacterial activities via various mechanisms such as nucleic acid synthesis inhibition, alteration in cytoplasmic membrane function, energy metabolism inhibition, reduction in cell attachment and biofilm formation, inhibition of the porin on the cell membrane, changing of the membrane permeability and attenuation of the pathogenicity.5,6 It also scavenge cancer cell by various molecular mechanisms viz., modulating ROS-scavenging enzyme activities, participating in arresting the cell cycle, inducing apoptosis, autophagy and suppressing cancer cell proliferation and invasiveness.7,8 Biologically derived compounds are proved to be safe to patients and these compounds will not cause any serious damage to normal cells. Moreover, treatment with these bio-compounds against bacterial pathogens and cancer will be cheap and safe.9–12 Medicinal plants the principal craftsman of novel candidates are well known to prevent and treat human ailments; conventional treatments have been known since ancient times.13 In addition, plants provide basic raw materials for various medicinal, pharmaceutical, agricultural, cosmetics and food industries.14
Ampelopsis grossedentata is an important edible medicinal plant commonly called as vine tea belonging to the plant family Vitaceae and is mainly distributed in southern China.15 Scientifically, high levels of flavonoids especially dihydromyricetin, myricetin and myricitrin were present in vine tea which were reported to possess antioxidant,16 antibacterial,17 antitumor,18 anti-inflammatory,19 neuroprotective,20 antihypertensive and antidiabetic activities.21 To the best of our knowledge the capacity of in vitro shoot culture of A. grossedentata in the production of secondary metabolites has not been studied until now. However, the callus culture could be a realistic source of bioactive secondary metabolites production with no time and space limitation and the technique has been widely used as an alternative for the production of plant secondary metabolites.22 Therefore, the current research was designed to establish the in vitro callus cultures of this species as an alternative bioactivity-based reproducible approach for extraction and isolation of key bioactive compounds. Further, we assessed the cytotoxicity and antibacterial potential of the callus extract on A549, 4T1 and NCI-H1975 and against a panel of Gram positive bacteria and Gram negative bacterial pathogens, respectively. Moreover, the active ingredients that play a crucial role in the biological activity of the extract were isolated and characterized using detailed spectroscopic analysis.
Results and discussion
Preliminary antibacterial activity and cytotoxicity
The antibacterial susceptibility of the callus extract (EtOH-AG) was determined and the results with zones of inhibition are tabulated (Table 2). Fascinatingly, the extract showed varying degrees of antibacterial activity against the tested bacterial pathogens. Particularly, the extract was found to be effective against methicillin-resistant Staphylococcus aureus (MRSA) and Staphylococcus epidermidis with highest zones of inhibition ranging from 10.88 ± 0.07–10.35 ± 0.07 mm. Further, EtOH-AG exhibited activities depending on bacterial strains with the MIC and MBC values in the ranges of 24.15–48.30 μg mL−1 and 96.60 μg mL−1, respectively. A previous report by Vignesh et al. (2022)23 stated that the callus extract of Saraca asoca showed remarkable antibacterial activity against Enterococcus faecium and Salmonella typhi with zones of inhibition of 17 and 14 mm, respectively. In addition, the extracts also exhibited maximum inhibitory effects at 75 μg mL−1 against the tested Gram positive and Gram negative bacterial pathogens. On the other hand, EtOH-AG exhibited substantial cytotoxicity against NCI-H1975, 4T1 and A549 cell lines with the GI50 values of 63.89, 69.10 and 74.00 μg mL−1 as shown in Fig. 1. The results were compared with standard drug doxorubicin. Our results are in agreement with the previous report of Li et al. (2022)24 in which callus extract exerted potent cytotoxic effect against the cancer cell lines. This significant in vitro antibacterial and cytotoxic activity is due to the presence of diverse phytochemicals in the callus extract of A. grossedentata inspired us to investigate its chemical constituents and to isolate the compounds.
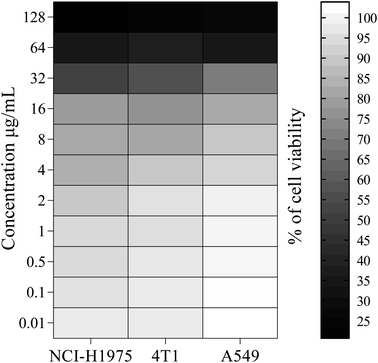 |
| Fig. 1 Comparative analysis of heatmap showing cytotoxic effects of A. grossedentata callus extract against NCI-H1975, 4T1 and A549 cells. The color codes of columns and rows represent the cell lines tested and the concentration of crude extract, respectively; similarly, the color codes for % of cell viability of NCI-H1975, 4T1 and A549 are depicted on the left side of the heatmap. | |
Isolation and identification of compounds from active callus extract
Compound 1 was obtained as a white powder; [α]D25 +52.6 (c 0.45, MeOH); UV (MeOH); λmax (log
ε) 244 (3.25), 263 (3.12), 273 (4.74) nm (Fig. S1†); IR (ATR)νmax 1803, 1922, 2036, 2867, 2901, 3228, 3796, 3935 cm−1; the molecular formula was established as C22H16O12 by negative HRESIMS m/z 471.0571 ([M
−
H]−, calcd for C22H15O12, 471.0569, err −0.4 ppm) (Fig. S2†). The 1H-NMR spectrum revealed six aromatic protons at δH 6.97 (2H, s), 6.52 (2H, s), 5.95 (2H, s), two oxygenated methine proton at δH 5.84 (1H, d, J = 11.4 Hz), 5.31 (1H, d, J = 11.4 Hz) (Fig. S3†). The 13C-NMR spectrum displayed 22 carbon resonance signals, which were assigned along with DEPT135, DEPT90 and HSQC spectroscopy as three benzene rings (Fig. S4–S7†). 15 carbon resonance signals at δC 74.31, 83.05, 96.79, 97.83, 102.24, 107.91, 128.08, 135.30, 146.59, 147.12, 164.38, 165.63, 166.90, 169.47 and 193.47 were in agreement with dihydromyricetin.44 7 carbon resonance signals at δC 110.56, 120.56, 140.38, 146.59 and 169.47 were in agreement with 3,4,5-trihydroxybenzoic acid.22 These carbon resonance signals revealed that compound 1 contained two structural units dihydromyricetin and 3,4,5-trihydroxybenzoic acid. To assign the relative configurations of compound 1, the ROESY correlations between H-1/H-2′, H-1/H-6′, H-2/H-2′ and H-2/H-6′ were done; it indicated that H-1, H-2 H-2′, and H-6′ were cofacial (Fig. S8 and S9†). Finally, compound 1 was identified as a new compound, and was named angelioue (Table 1; Fig. 2a).
Table 1 1H (600 MHz), 13C (150 MHz) and DEPT NMR spectroscopic data of compound 1 in CD3OD
Position |
δC |
Carbon type |
DEPT135 |
DEPT90 |
δH, multi. (J in Hz) |
2 |
83.05 |
CH |
82.92 |
82.92 |
5.31, d (11.4) |
3 |
74.31 |
CH |
74.17 |
74.17 |
5.84, d (11.4) |
4 |
193.47 |
CO |
|
|
|
5 |
164.38 |
C |
|
|
|
6 |
96.79 |
CH |
96.65 |
96.65 |
5.95 (s) |
7 |
165.63 |
C |
|
|
|
8 |
97.83 |
CH |
97.96 |
97.96 |
5.95 (s) |
9 |
166.90 |
C |
|
|
|
10 |
102.24 |
C |
|
|
|
1′ |
128.08 |
C |
|
|
|
2′ |
107.91 |
CH |
107.77 |
107.77 |
6.52 (s) |
3′ |
147.12 |
C |
|
|
|
4′ |
135.30 |
C |
|
|
|
5′ |
147.12 |
C |
|
|
|
6′ |
107.91 |
CH |
107.77 |
107.77 |
6.52 (s) |
1′′ |
120.56 |
C |
|
|
|
2′′ |
110.56 |
CH |
110.43 |
110.43 |
6.97 (s) |
3′′ |
146.59 |
C |
|
|
|
4′′ |
140.38 |
C |
|
|
|
5′′ |
146.59 |
C |
|
|
|
6′′ |
110.56 |
CH |
110.43 |
110.43 |
6.97 (s) |
|
169.47 |
COO |
|
|
|
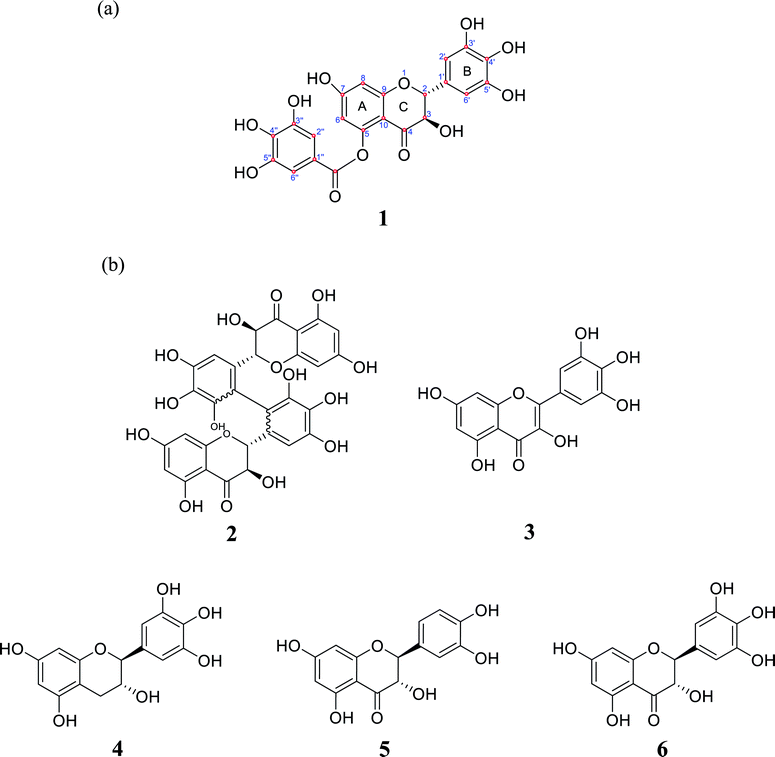 |
| Fig. 2 (a) Chemical structure of angelioue (1); (b2) cuminatanol (2), (b3) myricetin (3), (b4) epigallocatechin (4), (b5) taxifolin (5) and (b6) dihydromyricetin (6). | |
Compound 2 was obtained as a white powder and identified as acuminatanol (Fig. 2b2).25 Compound 3 was isolated as a yellow amorphous powder and identified as myricetin (Fig. 2b3).26 Compound 4 was obtained as a white amorphous powder and identified as epigallocatechin (Fig. 2b4).27 Compound 5 was obtained as a green amorphous powder and identified as taxifolin (Fig. 2b5).28 Compound 6 was obtained as a white amorphous powder and identified as dihydromyricetin (Fig. 2b6).29 The NMR, HRESIMS data and HPLC chromatograms of compounds 1–6 are given as supplementary files (Tables S1 and S2, Fig. S10–S35†).
Cytotoxicity and structure activity relationship of flavonoids
Flavonoids are specialized plant secondary metabolites which are ubiquitous throughout the plant kingdom. They belong to a chemically heterogeneous group of small molecules found in sufficient amounts in fresh fruits, green leafy vegetables, cocoa, tea, seed, tubers and peel with diverse biological activities. As part of our continuous search for potent anticancer compounds, the isolated flavonoids were evaluated for their cytotoxic efficiency against the aforementioned cell lines. All the tested compounds showed strong to weak anticancer activity in a concentration-dependent manner with lower GI50 values ranging from 6.93 to 98.36 μM mL−1 in comparison with standard drug doxorubicin against human lung adenocarcinoma A549 cells (A549), mouse breast cancer cells (4T1) and human non-small cell lung cancer (NCI-H1975). These data are consistent with preliminary cytotoxic potential of the extract; such a trend reported previously by Wang et al. (2016)30 in which the total flavonoid content of Cotinus coggygria Scop., significantly increased the tumor cell killing efficacy in a concentration-dependent manner against human glioblastoma cell lines compared with the control. Interestingly, among the compounds screened, dihydromyricetin (6) was able to reduce survival percentages as 13.07 ± 3.60–96.44 ± 2.07, 13.29 ± 0.84–89.66 ± 1.39 and 15.72 ± 1.88–98.32 ± 2.79% with GI50 values of 18.91, 17.47 and 20.50 μM mL−1 against A549, 4T1 and NCI-H1975 cancer cells, respectively (Fig. 3a–f). On the other hand angelioue (1), acuminatanol (2), myricetin (3), epigallocatechin (4) and taxifolin (5) exhibited moderate to weak inhibitory activities with low micro-molar concentration. The cytotoxicity was further validated by comparing with the reported results of Liu et al. (2014) in which dihydromyricetin (6) showed no cytotoxic effect against the normal human hepatic cell (HL7702 and L-02).9 Similarly another study conducted by Han et al. (2021) reported that dihydromyricetin showed no activity against MRC-5 fetal lung fibroblast and 267B1 prostate epithelial cells.10 In addition, Zhou et al. (2014) has reported that dihydromyricetin showed no cytotoxic effect on normal human breast epithelial cells (MCF-10A).11 Correlating the earlier reports obtained for the normal cells which showed that the dihydromyricetin was found to be selective towards cancer cells. Therefore, this study also confirmed their selective anticancer activity.9–11 Based on the current interest in flavonoids, the cytotoxic potential of the most active compound was further validated by structure–activity relationships (SAR) as predictors of cytotoxicity with most well-known mechanism of action involved in the anticancer activity of flavonoids. In this line, the SAR of the dihydromyricetin (6) can be explained as follows. It has been proposed that the carbonyl group (C
O) located at C4 position in the C ring showed a trend towards higher cytotoxicity of flavonoids at low micromolar concentration than other compounds without a carbonyl group in C4. The importance of the C4 carbonyl group for cytotoxicity is further underlined by the fact that compound 4 (epigallocatechin), the only compound in our study without a carbonyl group in C4 position, exhibited weak cytotoxicity among all tested compounds. These data are in conjunction with the previous report of Plochmann et al. (2007)31 in which catechin without a C4 carbonyl group exhibited lower cytotoxicity whereas taxifolin with a C4 carbonyl group showed 30-fold higher toxicity against human leukemia cells after 24 and 48 h treatment. This structure–activity study was further confirmed by another report of Mutoh et al. (2000)32 in which the presence of oxo group at C4 position of the C ring and a low electron density of 5 and 7 oxygen atoms in the hydroxyl group of the A ring were important in the suppression of cyclooxygenase-2 (COX-2) transcriptional activity that played an important role in carcinogenesis.30 In addition by correlating the previous report of Jacob et al. (2011),33 the presence of carbonyl at position 4 together with the hydroxyl at position 5 can be attributed to significant activity, but only if hydroxyl 3 is present; otherwise, the combination of 4-oxo with 5-OH is not active. Examining the involvement of the B ring on cytotoxic effects, Plochmann and his coworkers have reported that the ortho-hydroxylated flavonoid (quercetin) was about three times more cytotoxic than the meta-hydroxylated flavonoid (morin) signifying that the hydroxylation pattern of the B ring was involved in cytotoxic activity of ortho-hydroxylated compound (dihydromyricetin).31 In addition, this fact was also further confirmed by another report by Lopez-Lazaro, (2002)34 in which the number of hydroxyl groups on the B-ring was related to a molecular conformation that influenced the interactions between enzymes such as protein kinase C and tyrosine kinase with flavone thereby increasing the transcriptional activity of COX-2. Therefore, our results clearly suggest that an oxo group at 4 position of the C ring, the number of OH groups located on the B ring and the low electron density of the oxygen at 5 and 7 position of the A ring are important features of dihydromyricetin structure that afford increased cytotoxicity activity associated with cell shrinkage, cytoplasmic condensation and distortion which prevent tumor cell's metabolism and nutrient uptake leading to amplification of autophagy.
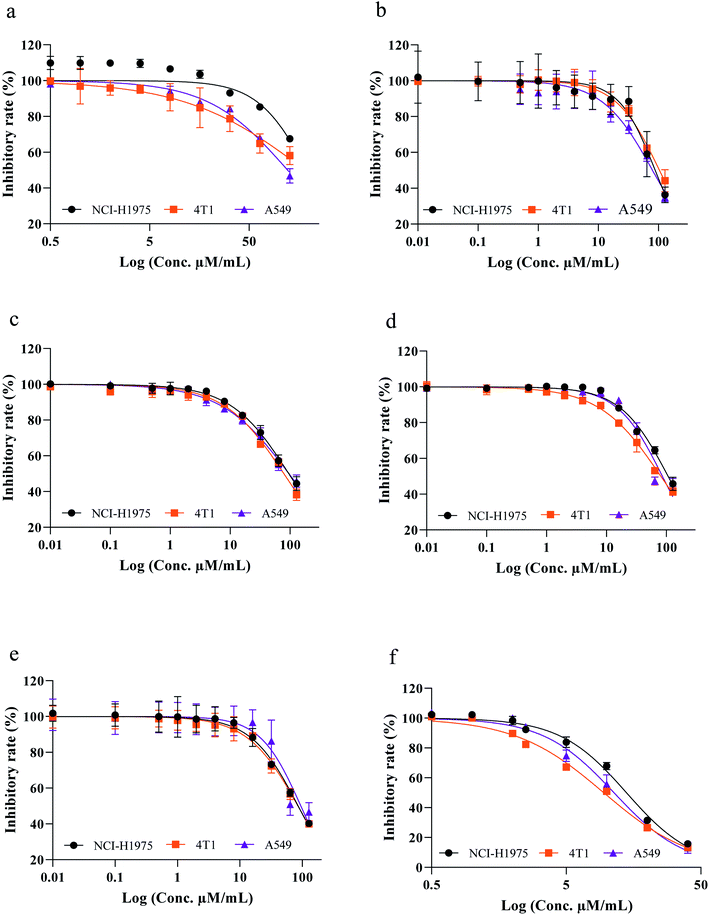 |
| Fig. 3 Cytotoxicity effects of the compounds 1–6, (3a) angelioue 1, (3b) cuminatanol 2, (3c) myricetin 3, (3d) epigallocatechin 4, (3e) taxifolin 5, and (3f) dihydromyricetin 6 against NC1-H1975, 4T1 and A549 cell lines. | |
Antibacterial activity and structure activity relationship of flavonoids
As presented in Table 2, all the six tested compounds displayed variable antibacterial activity at very low MIC and MBC values ranging from 6.67 to 99.48 and from 53.42 to 198.96 μg mL−1 against methicillin-resistant Staphylococcus aureus (MRSA), Staphylococcus epidermidis and Klebsiella pneumonia. Among the flavonoids tested, the new compound angelioue (1) exhibited significant bactericidal and bacteriostatic activity against MRSA with inhibition zone of 18.21 ± 0.31 mm (Fig. S36†) and the MIC value of 6.68 μM mL−1 and MBC value of 53.42 μM mL−1 which correlated well with previous reports of Alcaraz et al. (2000)35 and Yin et al. (2004)36 in which the flavonoids and its derivatives showed markedly strong in vitro antibacterial activity against MRSA, Staphylococcus aureus and S. epidermidis. The structural–antibacterial activity of angelioue (1) can be inferred from the results of significant anti-MRSA activity with some regular patterns of SAR, which are: the presence of adjacent hydroxyl group at C-3′, C-4′and C-5′ in the B ring greatly reduced the growth of bacteria. The antibacterial activity of the flavonoids has been proposed to be linked to their chemical structure especially the position and of number hydroxyl groups.35–37 Additionally, the presence of two hydroxyl substituents on C-7 and C-3 of A and C rings is presumed to possess good antibacterial activity.38 The previous research by Wu et al. (2013)39 reported that hydroxyl groups are an important aspect of antibacterial activity related to DNA gyrase inhibition of flavonoids. The importance of the C4 carbonyl group for anti-MRSA is further emphasized by the fact that flavones are less active than corresponding flavanone angelioue > dihydromyricetin > epigallocatechin > myricetin. This result may indicate that the saturation of the C2
C3 double bond increases the antibacterial activity.38 On the other hand, the presence of 5-gallate in the A ring offered a stronger antibacterial activity.40 Incidentally, the purified compounds exhibited higher cytotoxicity and antibacterial activities than crude extract; this may be due to the fact that purification process would have freed the active compounds which mask the biological activity of its chemical constituents on certain therapeutic targets. In this regard, Azizah et al. (2020) as reported that the mass spectrometry-based molecular networking is a powerful dereplication strategy that identifies known and unknown compounds in crude extract but also suggests the presence of related analogues for further drug discovery paradigms.41
Table 2 Antibacterial activity of callus extracts and compounds from A. grossedentataa
Microbes |
K. pneumonia |
MRSA |
S. epidermidis |
Test |
IOZ (mm) |
MIC |
MBC |
IOZ (mm) |
MIC |
MBC |
IOZ (mm) |
MIC |
MBC |
—No activity, IOZ–inhibition zones (in mm), MIC–minimum inhibitory concentration, MBC–minimum bacteriostatic concentration. |
Callus extract |
— |
24.15 |
96.60 |
10.88 ± 0.07 |
48.30 |
96.60 |
10.35 ± 0.07 |
48.30 |
96.60 |
Angelioue |
— |
— |
— |
18.21 ± 0.31 |
6.67 |
53.42 |
— |
— |
— |
Myricetin |
10.18 ± 0.55 |
24.87 |
99.48 |
10.77 ± 0.44 |
24.87 |
99.48 |
11.98 ± 0.27 |
99.48 |
198.96 |
Epigallocatechin |
— |
— |
— |
11.77 ± 0.40 |
19.14 |
76.56 |
12.05 ± 0.58 |
19.14 |
76.56 |
Dihydromyricetin |
12.10 ± 0.25 |
20.02 |
80.06 |
11.34 ± 0.15 |
10.01 |
80.06 |
11.87 ± 0.15 |
40.03 |
80.06 |
Kanamycin |
10.21 ± 0.34 |
0.02 |
3.13 |
— |
— |
— |
11.25 ± 0.38 |
0.02 |
6.25 |
Experimental
General experimental procedures
UV-Vis Spectroscopy was taken on Shimadzu UV 2550 spectrophotometer in analytical grade methanol. The optical rotation of pure compound was measured using digital polarimeter MCP 100 in CH3OH. FTIR and HR-ESI-MS spectra were recorded on a Nicolet iS20 FTIR Spectrometer and maXis II Q-TOF mass instrument (Bruker, Germany), respectively. NMR spectra were obtained on a Bruker NMR 600 spectrometer operated at 600 MHz (1H) and 150 MHz (13C) with TMS as an internal standard (Bruker, Germany). Thin-Layer Chromatography (TLC) was performed on 0.2 mm thick silica gel plates (HS-GF254). Analysis and preparation of compounds were performed using an Agilent 11260 Infinity II Prime with an ODS column (YMC, 250 × 10 mm, 5 μm). The organic solvents used for extraction and purification were procured from Beijing chemical factory (Beijing, China).
Plant material and callus induction
Ampelopsis grossedentata explants were obtained from Central China Medicinal Botanical Garden (Enshi, China, E109°45′24′′; N30°10′51′′) (Fig. S37a†). The identity of this plant material was verified by Dr Meijun He, Hubei Academy of Agricultural Sciences. The collected young shoot tips were washed in running tap water followed by rinsing with sterile water and soaked in 75% ethanol for 45 s with intermittent soaking in 0.1% HgCl2 for 20 min. The treated explants were washed with sterile water and then were cut into small pieces under aseptic condition. About 5–10 mm long shoot discs of in vitro explants were inoculated on 40 mL of sterile Murashige & Skoog medium (MS medium) with 3% sucrose, 1-naphthaleneacetic acid (NAA) (0.25 mg L−1), 6-benzylaminopurine (6-BA) (1.5 mg L−1) and 0.8% agar. The medium was adjusted to pH-5.8 before sterilization (121 °C, 15 psi, for 15 min). The cultures were kept at 25 ± 2 °C under photoperiod of 16 hours with white fluorescent light (50 μmol m−2s−1) for 14 days.
Extraction and isolation
The healthy callus tissues were collected at the maximum biomass production phase (four weeks of culture, Fig. S37b†) and used for extraction of secondary metabolites. Briefly, the harvested callus was dried in a hot air oven at 45 °C. The dried biomass of A. grossedentata callus weighing 324.25 grams was extracted thrice with ethanol (5L). The solvent was evaporated under reduced pressure to obtain yellowish green gum (6.48 g). The extract was fractionated over silica gel chromatographic column and eluted with solvents of increasing polarity of CHCl3/MeOH. A total of 9 fractions (Fr.A1–Fr.A9) were collected and similar fractions were combined based on their thin layer chromatography (TLC) profiles. Fr.A5 (318.47 mg) was fractionated over silica gel column chromatography to obtain 8 fractions (Fr.B1–Fr.B8). Fr.B4 and Fr.B5 were purified by semi-preparative high-performance liquid chromatography (HPLC) to give compound 2 (10.3 mg) and compound 4 (8.7 mg). Based on TLC profile, the Fr.A6 and Fr.A7 (528.48 mg) were combined and fractionated using silica gel column chromatography to obtain 12 fractions (Fr.C1–Fr.C12). Fr.C6 and Fr.C8 were purified by semi-preparative HPLC to give compound 3 (11.3 mg) and compound 5 (20.4 mg). Fr.A8 (108.48 mg) was fractionated using a silica gel column chromatography to obtain 10 fractions (Fr.D1–Fr.D10). Fr.D7–Fr.D9 were purified by semi-preparative HPLC to give compound 1 (9.3 mg) and compound 6 (7.5 mg). The purity of the isolated compounds was tested by HPLC, and the purity of which exceeded 98% was further identified (Fig. S35†).
Cell line maintenance and viability assay
Human lung adenocarcinoma A549 cells ATCC CCL-185 (A549), mouse breast cancer cells ATCC CRL-2539 (4T1) and human non-small cell lung cancer ATCC CRL-5908 (NCI-H1975) cells were obtained from the department of Central Hospital of Tujia and Miao Autonomous Prefecture were grown in RPMI-1640 medium supplemented with 10% FBS (v/v), 1% antibiotic and antimycotic solution (1000 U mL−1 penicillin and 10 mg mL−1 streptomycin sulphate), and maintained in 5% CO2 and 95% air with 90% relative humidity at 37 °C in CO2 incubator. Callus extract and compounds were evaluated for their cytotoxic activity on aforementioned cancer cell lines. Cell viability was determined by Quanti-Blue reduction assay. Briefly, the cells were inoculated in 96-well microtiter plate (5 × 104 cells per well) and treated with callus extract and compounds at concentrations of 0.01–128 μM mL−1. DMSO and doxorubicin were added as solvent and positive controls. After 24 h of treatment the cells were incubated at 37 °C for 2 h with 10% Quanti-Blue solution (v/v). Viability was calculated by measuring the OD value at 544 nm and 590 nm with a plate reader.45
Microbes and antibacterial activity assay
A panel of selected Gram positive bacterial pathogens such as methicillin-resistant Staphylococcus aureus ATCC 43300 (MRSA), Staphylococcus epidermidis ATCC 12228 (MRSE) and Gram negative bacterium Klebsiella pneumonia ATCC 13883 were obtained from the Key Laboratory of Tropical Marine Biological Resources and Ecology, South China Sea Institute of Oceanology, Chinese Academy of Sciences. The antibacterial susceptibility testing of the extract and compounds were performed using disc diffusion assay by following the method of Kumar et al. (2017).42 Briefly, the fresh bacterial inoculums were prepared by growing the cells in Mueller–Hinton broth (MHB, OXOID, USA) overnight at 37 °C. About 50 μL of freshly prepared test cultures corresponding to 1 × 104−6 CFU mL−1 were swabbed on the sterile Mueller–Hinton agar (MHA, OXOID, USA) and allowed to dry for 5 minutes. After drying, the pre-coated paper discs containing extracts (10 μL per disc) and compounds (5 μL per disc) were placed on the surface of the test plates and kept at 37 °C overnight. Kanamycin (50 μg per disc) DMSO (5 μL per disc) was used as positive and solvent controls, respectively. The antibacterial activity was perceived as a zone of clearing in the turbid medium around the disk containing inhibitory activity. The clear zones were measured using a zone scale from Hi-media and expressed in millimeters.
Determination of minimum inhibitory concentration (MIC) and minimum bacteriostatic concentration (MBC)
The MIC and MBC values of extract and compounds were determined by using broth microdilution assay in sterile 96-well microtiter plate according to Li et al. (2022) and Rozman et al. (2017).24,43 About 25 μL of extract and purified compounds were serially diluted in 75 μL of MHB to obtain 0–200 μg mL−1 and 0–100 μM mL−1, respectively and 5 μL of freshly grown bacterial cultures were added to each well. DMSO and kanamycin were added as solvent and positive controls. The treated plates were kept at 37 °C for 24 hours followed by addition of 40 μL of iodonitrotetrazolium chloride (200 μg mL−1) into each well and re-incubated at 3 °C for 30 min. The colour changed from yellow to purple indicating the microbial growth in the well. To check the viability of the tested bacterial pathogens, the mixture in each well was streaked on MHA and incubated overnight at 37 °C.
Statistical analysis
All statistical analyses were performed using the SPSS software 24.0 (Systat Software, Systat, Evanston, IL, USA). Data were presented as mean ± SEM. The significance among all groups was evaluated with one-way ANOVA. P < 0.05 was considered statistically significant. The Anova analyses were carried out using Graphpad version 8.4.3 for Windows version 7.
Conclusions
In summary, the study of Ampelopsis grossedentata callus extract led to the isolation and characterization of one novel compound along with five known compounds with narrow spectrum antibacterial and cytotoxic activities. It is noteworthy that the new compound angelioue (1) showed significant anti-methicillin-resistant Staphylococcus aureus activity and the known compound dihydromyricetin (6) exerted significant cytotoxicity against mouse breast cancer cells (4T1), Human lung adenocarcinoma A549 cells (A549) and human non-small cell lung cancer (NCI-H1975) tumor cell lines with very low micro-molar concentrations. In addition, the structure–activity relationship (SAR) provided vital information regarding the types of moieties that were involved in the suppression of bacteria and cancer cells. Therefore, the results of this study clearly demonstrate that the observed antibacterial and cytotoxic activities of these compounds may justify the use of the extracts of this plant in folk medicine for the treatment of bacterial infections and cancer. This warrants further attention in the future for the drug development.
Author contributions
Conceptualization – Yu Li, Pachaiyappan Saravana Kumar and Meijun He; methodology – Jiao Qiu and Xuhui Tan; software and validation – Shengquan Tan, Jiao Qiu and Chuying Huang; formal analysis, investigation and resources – Zhixin Xiang and Pachaiyappan Saravana Kumar; data curation– Meijun He; writing – original draft preparation and writing-review and editing – Yu Li and Pachaiyappan Saravana Kumar; visualization – Jianqun Luo; supervision – Shengquan Tan; project administration and funding acquisition – Meijun He and Yu Li.
Conflicts of interest
There are no conflicts to declare.
Acknowledgements
This research was funded by Hubei Academy of Agricultural Sciences, grant number 2022NKYJJ17; Hubei Technology Innovation Center for Agricultural Sciences –‘2020 key technology research and demonstration project of safe and efficient production of genuine medicinal materials’, grant number 2020-620-000-002-04; Modern Agricultural Industrial Technology System in Hubei Province: Innovation and Demonstration of Production Technology of Famous-region drug, grant number HBHZD-ZB-2020-005 and Study on key technology of solid beverage processing using Selenium-rich Pueraria thomsonii Benth as raw material, grant number D20210018; and We thank all the technicians and faculty in the analytical facility center of the Institute of Chinese Herbal Medicines, Hubei Academy of Agricultural Sciences, China.
Notes and references
- S. J. Dunachie, N. P. Day and C. Dolecek, Curr. Opin. Microbiol., 2020, 57, 95–101 CrossRef PubMed.
- F. M. Aarestrup, Basic Clin. Pharmacol. Toxicol., 2005, 96, 271–281 CrossRef CAS PubMed.
- S. S. Coughlin and D. U. Ekwueme, Cancer Epidemiol., 2009, 33, 315–318 CrossRef PubMed.
- S. Khanna, Int. J. Environ. Res. Publ. Health, 2008, 5, 418–422 CrossRef CAS PubMed.
- F. Farhadi, B. Khameneh, M. Iranshahi and M. Iranshahy, Phytother. Res., 2019, 33, 13–40 CrossRef CAS PubMed.
- N. F. Shamsudin, Q. U. Ahmed, S. Mahmood, S. A. Ali Shah, A. Khatib, S. Mukhtar, M. A. Alsharif, H. Parveen and Z. A. Zakaria, Molecules, 2022, 27(4), 1149 CrossRef CAS PubMed.
- D. M. Kopustinskiene, V. Jakstas, A. Savickas and J. Bernatoniene, Nutrients, 2020, 12(2), 457 CrossRef CAS PubMed.
- U. T. Ferdous and Z. N. Balia Yusof, Molecules, 2021, 26, 6844 CrossRef CAS PubMed.
- J. Liu, Y. Shu, Q. Zhang, B. Liu, J. Xia, M. Qiu, H. Miao, M. Li and R. Zhu, Oncol. Lett., 2014, 8, 1645–1651 CrossRef PubMed.
- J. M. Han, H. L. Kim and H. J. Jung, Int. J. Mol. Sci., 2021, 22, 4265 CrossRef CAS PubMed.
- Y. Zhou, F. Shu, X. Liang, H. Chang, L. Shi, X. Peng, J. Zhu and M. Mi, PLoS One, 2014, 9, e89021 CrossRef PubMed.
- B. S. Negi and B. P. Dave, J. Pure Appl. Microbiol., 2010, 4, 557–564 Search PubMed.
- M. Adnan, R. Bibi, S. Mussarat, A. Tariq and Z. K. Shinwari, Ann. Clin. Microbiol. Antimicrob., 2014, 13, 1–18 CrossRef PubMed.
- P. K. Rai and H. Lalramnghinglova, Ethnobot. leafl., 2010, 14, 247–305 Search PubMed.
- L. Gu, N. Zhang, C. Feng, Y. Yi and Z. W. Yu, Mitochondrial DNA, 2020, 5, 2423–2424 CrossRef PubMed.
- X. Zhang, Y. Xu, H. Xue, G. C. Jiang and X. J. Liu, Food Sci. Nutr., 2019, 7, 1735–1745 CrossRef CAS PubMed.
- H. Liang, K. He, T. Li, S. Cui, M. Tang, S. Kang, W. Ma and L. Song, Sci. Rep., 2020, 10, 21416 CrossRef CAS PubMed.
- H. Tong, X. Zhang, L. Tan, R. Jin, S. Huang and X. Li, Eur. J. Pharmacol., 2020, 870, 172888 CrossRef CAS PubMed.
- H. Y. Zhou, S. Q. Gao, Y. S. Gong, T. Lin, S. Tong, W. Xiong, C. Y. Shi, W. Q. Wang and J. G. Fang, J. Global Antimicrob. Resist., 2020, 23, 370–376 CrossRef PubMed.
- J. Qian, X. Wang, J. Cao, W. Zhang, C. Lu and X. Chen, Neurosci. Lett., 2021, 756, 135963 CrossRef CAS PubMed.
- Q. Zhang, Y. Zhao, M. Zhang, Y. Zhang, H. Ji and L. Shen, J. Pharm. Anal., 2020, 11(5), 555–563 CrossRef PubMed.
- M. A. Kamarul Zaman, A. M. Azzeme, I. K. Ramle, N. Normanshah, S. N. Ramli, N. A. Shaharuddin, S. Ahmad and S. N. A. Abdullah, Plants (Basel), 2020, 9, 1772 CrossRef PubMed.
- A. Vignesh, S. Selvakumar and K. Vasanth, Phytomed. Plus, 2022, 2, 100167 CrossRef.
- Y. Li, P. S. Kumar, Y. Ran, X. Tan, R. Zhao, L. Ai, M. Yuan, J. Zhu and M. He, Arab. J. Chem., 2022, 15(6), 103854 CrossRef CAS.
- J.-F. Hu, E. Garo, G. W. Hough, M. G. Goering, M. O'Neil-Johnson and G. R. Eldridge, Tetrahedron Lett., 2007, 48, 5747–5749 CrossRef CAS.
- C. Seo, E.-K. Ahn, J.-S. Kang, J.-H. Lee, J. S. Oh and S. S. Hong, Phytochem. Lett., 2017, 20, 93–97 CrossRef CAS.
- A. Suedee, S. Tewtrakul and P. Panichayupakaranant, Pharm. Biol., 2014, 52, 58–61 CrossRef CAS PubMed.
- M. Matsuda, Y. Otsuka, S. Jin, J. Wasaki, J. Watanabe, T. Watanabe and M. Osaki, Biochem. Biophys. Res. Commun., 2008, 366, 414–419 CrossRef CAS PubMed.
- Z. J. Hui, C. Gang, F. L. Xue, H. S. Yun, K. Y. Shi, Z. Lu, Y. Ming and D. Z. Wei, Chem. Nat. Compd., 2009, 45, 85–86 CrossRef.
- G. Wang, J.-J. Wang, L. Du, L. Fei and S.-s. T. To, Nutr. Cancer, 2016, 68, 1357–1368 CrossRef CAS PubMed.
- K. Plochmann, G. Korte, E. Koutsilieri, E. Richling, P. Riederer, A. Rethwilm, P. Schreier and C. Scheller, Arch. Biochem. Biophys., 2007, 460, 1–9 CrossRef CAS PubMed.
- M. Mutoh, M. Takahashi, K. Fukuda, H. Komatsu, T. Enya, Y. Matsushima-Hibiya, H. Mutoh, T. Sugimura and K. Wakabayashi, Jpn. J. Cancer Res., 2000, 91, 686–691 CrossRef CAS PubMed.
- V. Jacob, T. Hagai and K. Soliman, Curr. Org. Chem., 2011, 15, 2641–2657 CrossRef CAS.
- M. Lopez-Lazaro, Curr. Med. Chem. Anticancer Agents, 2002, 2, 691–714 CrossRef CAS PubMed.
- L. Alcaraz, S. Blanco, O. Puig, F. Tomas and F. Ferretti, J. Theor. Biol., 2000, 205, 231–240 CrossRef CAS PubMed.
- S. Yin, C.-Q. Fan, Y. Wang, L. Dong and J.-M. Yue, Bioorg. Med. Chem., 2004, 12, 4387–4392 CrossRef CAS PubMed.
- H. Tsuchiya, M. Sato, T. Miyazaki, S. Fujiwara, S. Tanigaki, M. Ohyama, T. Tanaka and M. Iinuma, J. Ethnopharmacol., 1996, 50, 27–34 CrossRef CAS.
- Y. Xie, W. Yang, F. Tang, X. Chen and L. Ren, Curr. Med. Chem., 2015, 22, 132–149 CrossRef CAS PubMed.
- T. Wu, X. Zang, M. He, S. Pan and X. Xu, J. Agric. Food Chem., 2013, 61, 8185–8190 CrossRef CAS PubMed.
- M. A. Farag, D. A. Al-Mahdy, R. Salah El Dine, S. Fahmy, A. Yassin, A. Porzel and W. Brandt, Chem. Biodivers., 2015, 12, 955–962 CrossRef CAS PubMed.
- M. Azizah, P. Pripdeevech, T. Thongkongkaew, C. Mahidol, S. Ruchirawat and P. Kittakoop, Antibiotics, 2020, 9, 606 CrossRef CAS PubMed.
- P. S. Kumar, A. Stalin, V. Duraipandiyan, N. A. Al-Dhabi, P. Yuvaraj, K. Balakrishna and S. Ignacimuthu, Bangladesh J. Biol. Agric. Sci., 2017, 6, 15–23 Search PubMed.
- N. Rozman, N. Hamin, L. C. Ring, T. W. Nee, M. B. Mustapha and T. W. Yenn, Mycobiology, 2017, 45, 178–183 CrossRef PubMed.
- A. Baiseitova, J. Jenis, J. Y. Kim, Z. P. Li and K. H. Park, Nat. Prod. Res., 2021, 35(5), 880–883 CrossRef CAS PubMed.
- C. C. Su, S. C. Wang, I. C. Chen, F. Y. Chiu, P. L. Liu, C. H. Huang and C. Y. Li, Front. Pharmacol., 2021, 12, 1–12 Search PubMed.
|
This journal is © The Royal Society of Chemistry 2022 |