DOI:
10.1039/D2RA03237F
(Paper)
RSC Adv., 2022,
12, 27125-27130
Particle size effect on millimeter-wave absorption, rotation, and ellipticity of gallium-substituted epsilon iron oxide†
Received
23rd May 2022
, Accepted 19th September 2022
First published on 23rd September 2022
Abstract
Various applications employ millimeter waves. For example, the carrier frequencies of vehicle radar in advanced driver assistance systems are 76–81 GHz millimeter waves. Here, we investigate the particle size effect on millimeter-wave absorption of gallium-substituted epsilon iron oxide ε-GaxFe2−xO3 with x = 0.44 ± 0.01. Samples were composed of nanoparticles with sizes of 16.9(1) nm, 28.8(2) nm, and 41.4(1) nm. Millimeter wave absorption, Faraday rotation, and Faraday ellipticity were measured by terahertz time-domain spectroscopy. This series exhibits millimeter-wave absorption at 78.7, 78.2, and 77.7 GHz without an external magnetic field. The millimeter-wave absorption increases from 4.6 dB to 9.4 dB as the particle size increases. In the magnetized sample, the Faraday rotation angle increases from 9.1° to 18.4°, while the Faraday ellipticity increases from 0.27 to 0.52. The particle size effect can be explained by the change in the ratio of the surface and core of the nanoparticles. The present study should contribute to the realization of high-performance millimeter-wave absorbers.
Introduction
Advanced driver assistance systems (ADAS) for automobiles are becoming ubiquitous.1,2 Millimeter-wave car radars (76.5 GHz, 79 GHz, and 81 GHz) are equipped in ADAS for forward monitoring because they are less susceptible to weather conditions such as rain and fog.3,4 Additionally, the demand for technologies to suppress noise and electromagnetic interference is increasing.5,6 Consequently, the development of millimeter-wave absorbers and circulators is an important issue. Magnetic materials can absorb electromagnetic waves, which are due to magnetic loss.7–15 However, the absorption frequencies of magnetic materials such as ferrite and metal are generally low compared to the carrier frequency of millimeter-wave car radar.16,17 Epsilon iron oxide ε-Fe2O3 nanomagnets have attracted much attention. They exhibit large magnetic anisotropy and high-frequency millimeter-wave absorption.18–45 Additionally, metal-substituted epsilon iron oxide, ε-MxFe2−xO3,46–70 exhibits millimeter-wave absorption in the range of 35–222 GHz, in which the resonance frequency is controlled by a kind of substitution metal and substitution ratio. Recently, the resonance frequency shift due to change on the particle size of ε-Fe2O3 nanomagnets has been also reported.45
Gallium-substituted epsilon iron oxide ε-GaxFe2−xO3 is suitable for the resonance of the millimeter-wave car radar frequency and should serve as a millimeter-wave absorber.52 To enhance the millimeter-wave absorption, we investigate the effect of particle size on the millimeter-wave absorption properties of ε-GaxFe2−xO3 nanoparticles. In the present work, ε-GaxFe2−xO3 with different nanoparticle sizes are prepared by changing the sintering temperature in the range of 1050–1150 °C. Then the magnetic properties and millimeter-wave absorption are measured. In addition, Faraday rotation and Faraday ellipticity are also measured from the viewpoint of application for millimeter wave devices such as isolators and circulators. Finally, the particle size effect is discussed.
Results and discussion
Materials, crystal structure, and morphology
The samples were prepared by the sol–gel method according to the literature.52 Iron nitrate and gallium nitrate were dissolved in water with a ratio of Ga
:
Fe = 0.46
:
1.54, and ammonia and tetraethyl orthosilicate were added successively to obtain precursors. The precursors were sintered in air for 4 hours. To etch the silica matrix, the sintered samples were heated with a sodium hydroxide aqueous solution at 60 °C for 1 day. Three samples were prepared at different sintering temperatures: 1050 °C (1), 1100 °C (2), and 1150 °C (3).
Elemental analyses with inductively coupled plasma mass spectroscopy indicates that the formulas of the obtained samples are ε-GaxFe2−xO3, where x = 0.43(1) for 1, 0.45(1) for 2, and 0.45(2) for 3. The powder X-ray diffraction (PXRD) patterns show that all samples have an isomorphic structure of ε-Fe2O3 (orthorhombic structure, space group of Pna21) (Fig. S1 and Table S1†). The crystal structure has four non-equivalent metal sites (A–D sites) (Fig. 1a). The Ga ion tends to substitute the D site. The crystalline size evaluated from the PXRD patterns using the FP method (dFP) increases as the sintering temperature increases: 16.9(1) nm for 1, 28.8(2) nm for 2, and 41.4(1) nm for 3. TEM images confirm that the obtained samples are composed of nanoparticles (Fig. S2†). The particle size evaluated from the TEM images also becomes larger as the sintering temperature increases: 15 ± 6 nm (1), 23 ± 11 nm (2), and 33 ± 18 nm (3) (Fig. 1b).
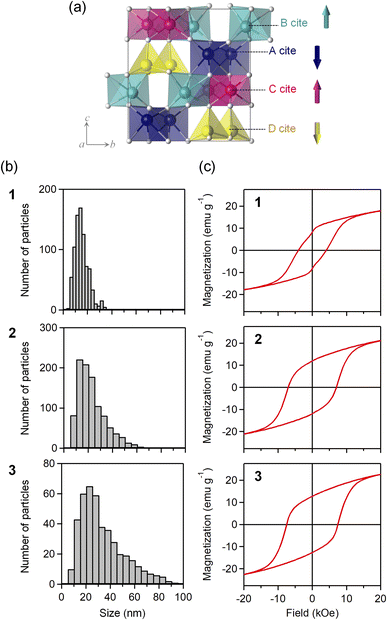 |
| Fig. 1 (a) Crystal structure of ε-GaxFe2−xO3. Arrows indicate the sublattice magnetization directions of ε-GaxFe2−xO3. (b) The distribution of particle size measured from the TEM images. (c) Magnetic hysteresis loops measured at 300 K. | |
Magnetic properties
The magnetic hysteresis was measured at 300 K using a superconducting quantum interference device (SQUID) magnetometer. The coercive fields are 4.1 (1), 7.1 (2), and 7.5 kOe (3) (Fig. 1c). The magnetization values at 5 tesla are 21.5 (1), 25.3 (2), and 26.7 emu g−1 (3). The magnetism of ε-GaxFe2−xO3 is classified as a ferrimagnet, where the sublattice magnetizations at the B and C sites are positive, while the sublattice magnetizations at the A and D sites are negative.71–76 The D site has a smaller sublattice magnetization than those at the other sites, resulting in the appearance of ferrimagnetism.
Millimeter-wave absorption
The millimeter-wave absorption was measured by using a terahertz time-domain spectroscopy (THz-TDS) system. To measure millimeter-wave absorption, a THz pulse was irradiated to the pellet-formed sample and a transmitted THz pulse and reflected pulse were obtained (Fig. S3†). Powder-formed samples were compressed into pellets (diameter: 13 mm ϕ) with a thickness of 1.02 (1), 0.98 (2), and 1.00 mm (3). The filling ratios were 56.8 (1), 59.5 (2), and 59.2 vol% (3). Fig. 2 shows the millimeter-wave absorption spectra. All samples have absorption peaks due to the natural resonance at 78.7 (1), 78.2 (2), 77.7 GHz (3). The millimeter-wave absorption increases with increasing the particle size: 4.6 (1), 7.9 (2), and 9.4 dB (3). The absorption peak area also increased as 38 (1), 52 (2), and 56 dB GHz (3).
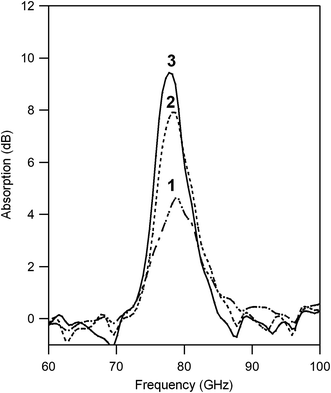 |
| Fig. 2 Millimeter-wave absorption spectra for 1 (dashed line), 2 (dotted line), and 3 (solid line). | |
Faraday rotation and ellipticity in the millimeter-wave region
To measure the Faraday rotation and ellipticity in the millimeter-wave region, wire grid polarizers were introduced in the THz-TDS system.47,53,57 Polarizers were placed after the emitter and before the detector to precisely define the polarization of the THz pulse as horizontal. The vertical and horizontal components of the transmitted THz pulses were obtained as the difference or the sum of the transmitted THz pulses with the polarizers set as 45° and −45°. The rotation angle and ellipticity of the transmitted light were obtained from the vertical and horizontal components. Prior to the measurements, the pellet samples were magnetized by placing the pellet under a magnetic field of 8 tesla.
Fig. 3a shows the rotation angle and ellipticity in the millimeter-wave region, where the incident THz pulse was irradiated from the S-pole of the magnetized pellet sample. The rotation angle spectrum shows the dispersive shape centered at the peak frequency of ellipticity. The rotation angle increases as the particle size becomes larger: 9.1° (1), 14.1° (2), and 18.4° (3). The Faraday ellipticity shows the peak at the same frequency as the absorption peak frequency. The ellipticity increases with increasing the particle size: 0.27 (1), 0.39 (2), and 0.52 (3). Irradiating the incident THz pulse from the opposite pole, N-pole, the signs of rotation angle and ellipticity change (Fig. 3b and c).
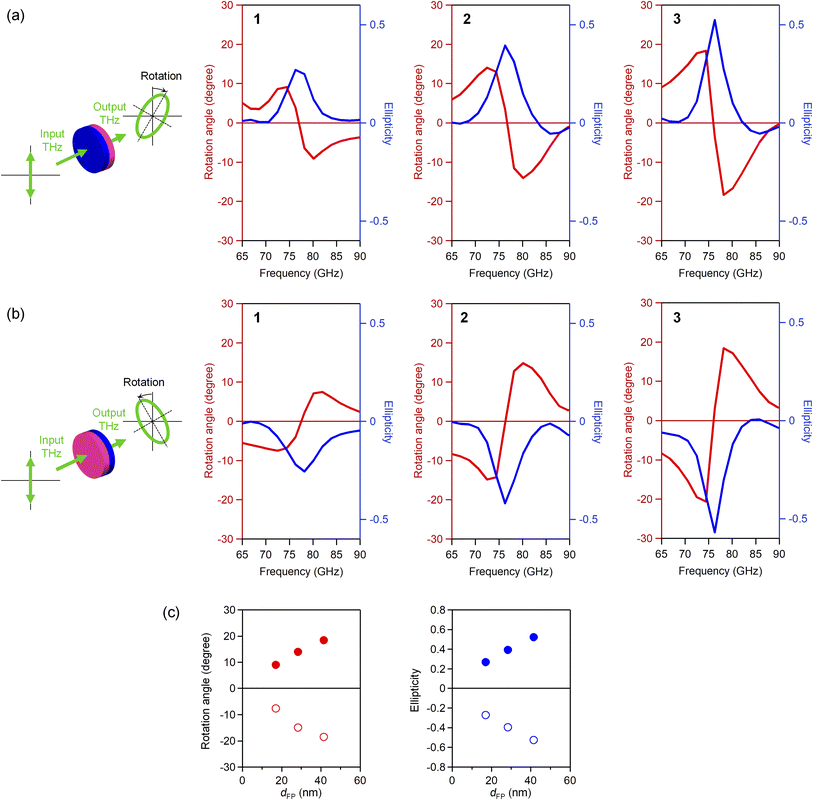 |
| Fig. 3 (a) and (b) Faraday rotation angle (red) and Faraday ellipticity (blue) measured by THz-TDS. The incident THz pulse was irradiated from S-pole (a) and N-pole (b) of the magnetized pellet sample. Left figures show the schematic illustration of the millimeter wave rotation. (c) Faraday rotation angle and Faraday ellipticity versus the particle size, measured by irradiating THz pulse from the S-pole side (close circles) and the N-pole side (open circles) of the magnetized pellets. | |
Mechanism of particle size effect
Here, we consider the particle size effect on millimeter-wave absorption. The plot of the absorption versus the particle size shows that the absorption decreases as the particle size decreases (Fig. 4). We assume that this decrease is due to the loss of ferromagnetism of the iron atoms near the surface. The TEM images show that the present materials are composed of nanoparticles. The volume of the core and the total volume of the particle are expressed as 4π(d/2 − ds)3/3 and 4π(d/2)3/3, respectively, where ds shows the thickness from the surface.
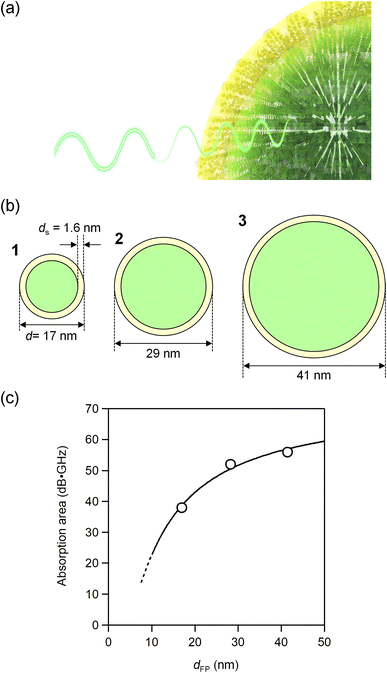 |
| Fig. 4 (a) Schematic illustration of millimeter wave absorption. The core (green) absorbs millimeter waves but the surface (yellow) does not. (b) Schematic illustration of the nanoparticles of 1–3 showing the thickness from the surface ds. (c) Absorption peak area versus the particle size (open circles) and the fitted line by eqn (1) (solid line). | |
Therefore, the ratio of the core volume to the total particle volume is {4π(d/2 − ds)3/3}/{4π(d/2)3/3}. Assuming that the core contributes to absorption, the absorption area (A) is described by
|
A = A∞{4π(d/2 − ds)3/3}/{4π(d/2)3/3},
| (1) |
where
A∞ is the absorption area without the surface effects. The particle size dependence of the absorption is well fitted by
eqn (1), indicating that the ferromagnetism near the surface layer is lost. Hence, millimeter-waves cannot be absorbed at the surface. The fitted value of
ds = 1.6 nm almost corresponds to one unit cell of the crystal structure. The value of
A∞ = 73 dB GHz is the intrinsic absorption of the present series with a filling ratio of 60 vol%, which corresponds to 120 dB GHz mm
−1. Therefore, a larger particle size may realize a larger absorption as a millimeter-wave absorber and a larger Faraday rotation and ellipticity effect in the millimeter-wave region.
Conclusions
Herein the millimeter-wave absorption, rotation, and ellipticity of ε-GaxFe2−xO3 with various particle sizes were investigated. Increasing the particle size improves the millimeter-wave absorption and rotation properties of ε-GaxFe2−xO3. Analysis of the particle size dependence indicates that ferromagnetism near the surface layer is lost, inhibiting the absorption of millimeter-waves. The thickness of 1.6 nm almost corresponds to one unit cell of the crystal structure. Therefore, a larger particle size may achieve a larger absorption for an absorber as well as a larger Faraday rotation and ellipticity effect in the millimeter-wave region.
Conflicts of interest
There are no conflicts to declare.
Acknowledgements
This work was supported in part by a Grant-in-Aid for Scientific Research (A) from the Japan Society for the Promotion of Science (JSPS) (Grant Number 20H00369). S. S. acknowledges the support of Materials Education program for the Future Leaders in Research, Industry, and Technology (MERIT). We recognize the Cryogenic Research Center at The University of Tokyo, DOWA Technofund, and the Center for Nano Lithography & Analysis at The University of Tokyo.
Notes and references
- J. Hasch, E. Topak, R. Schnabel, T. Zwick, R. Weigel and C. Waldschmidt, IEEE Trans. Microwave Theory Tech., 2012, 60, 845–860 Search PubMed.
- S. Patole, M. Torlak, D. Wang and M. Ali, IEEE Signal Processing Magazine, 2017, 34, 22–35 Search PubMed.
- K. Yoneda, N. Suganuma, R. Yanase and M. Aldibaja, IATSS Research, 2019, 43, 253–262 CrossRef.
- D. Barnes, M. Gadd, P. Murcutt, P. Newman and I. Posner, Proceedings of IEEE International Conference on Robotics and Automation, 2020, 6433–6438 Search PubMed.
- S. Geetha, K. K. Satheesh Kumar, C. R. K. Rao, M. Vijayan and D. C. Trivedi, J. Appl. Polym. Sci., 2009, 112, 2073–2086 CrossRef CAS.
- C. M. Watts, X. Liu and W. J. Padilla, Adv. Mater., 2012, 24, OP98–OP120 CAS.
- M. Pardavi-Horvath, J. Magn. Magn. Mater., 2000, 215–216, 171–183 CrossRef CAS.
- J. D. Adam, L. E. Davis, G. F. Dionne, E. F. Schloemann and S. N. Stitzer, IEEE Trans. Microwave Theory Tech., 2002, 50, 721–737 CrossRef CAS.
- R. K. Selvan, V. Krishnan, C. O. Augustin, H. Bertagnolli, C. S. Kim and A. Gedanken, Chem. Mater., 2008, 20, 429–439 CrossRef CAS.
- V. G. Harris, IEEE Trans. Magn., 2012, 48, 1075–1104 CAS.
- X. Huang, J. Zhang, M. Lai and T. Sang, J. Alloys Compd., 2015, 627, 367–373 CrossRef CAS.
- X. Yang, Z. Zhou, T. Nan, Y. Gao, G. M. Yang, M. Liu and N. X. Sun, J. Mater. Chem. C, 2016, 4, 234–243 RSC.
- A. Houbi, Z. A. Aldashevich, Y. Atassi, Z. B. Telmanovna, M. Saule and K. Kubanych, J. Magn. Magn. Mater., 2021, 529, 167839 CrossRef CAS.
- D. Liu, Y. Du, P. Xu, N. Liu, Y. Wang, H. Zhao, L. Cui and X. Han, J. Mater. Chem. C, 2019, 7, 5037–5046 RSC.
- J. Shen, Y. Yao, Y. Liu and J. Leng, J. Mater. Chem. C, 2016, 4, 7614–7621 RSC.
- B. D. Cullity and C. D. Graham, Introduction to Magnetic Materials, Wiley-IEEE Press, New Jersey, 2nd edn, 2008 Search PubMed.
- A. Goldman, Modern Ferrite Technology, Springer, New York, 2nd edn, 2006 Search PubMed.
- J. Jin, S. Ohkoshi and K. Hashimoto, Adv. Mater., 2004, 16, 48–51 CrossRef CAS.
- E. Tronc, C. Chanéac, J. P. Jolivet and J. M. Grenèche, J. Appl. Phys., 2005, 98, 053901 CrossRef.
- J. Jin, K. Hashimoto and S. Ohkoshi, J. Mater. Chem., 2005, 15, 1067–1071 RSC.
- S. Sakurai, J. Jin, K. Hashimoto and S. Ohkoshi, J. Phys. Soc. Jpn., 2005, 74, 1946–1949 CrossRef CAS.
- Y.-C. Tseng, N. M. Souza-Neto, D. Haskel, M. Gich, C. Frontera, A. Roig, M. van Veenendaal and J. Nogués, Phys. Rev. B: Condens. Matter Mater. Phys., 2009, 79, 094404 CrossRef.
- J. Tuček, R. Zbořil, A. Namai and S. Ohkoshi, Chem. Mater., 2010, 22, 6483–6505 CrossRef.
- M. Gich, I. Fina, A. Morelli, F. Sánchez, M. Alexe, J. Gàzquez, J. Fontcuberta and A. Roig, Adv. Mater., 2014, 26, 4645–4652 CrossRef CAS.
- G. Carraro, A. Gasparotto, C. Maccato, V. Gombac, F. Rossi, T. Montini, D. Peeters, E. Bontempi, C. Sada, D. Barreca and P. Fornasiero, RSC Adv., 2014, 4, 32174–32179 RSC.
- S. Bhattacharya, A. Roychowdhury, D. Das and S. Nayar, RSC Adv., 2015, 5, 89488–89497 RSC.
- S. Ohkoshi, A. Namai, K. Imoto, M. Yoshikiyo, W. Tarora, K. Nakagawa, M. Komine, Y. Miyamoto, T. Nasu, S. Oka and H. Tokoro, Sci. Rep., 2015, 5, 14414 CrossRef CAS.
- J. López-Sánchez, A. Muñoz-Noval, A. Serrano, M. Abuín, J. de la Figuera, J. F. Marco, L. Pérez, N. Carmona and O. Rodríguez de la Fuente, RSC Adv., 2016, 6, 46380–46387 RSC.
- S. Ohkoshi, A. Namai, T. Yamaoka, M. Yoshikiyo, K. Imoto, T. Nasu, S. Anan, Y. Umeta, K. Nakagawa and H. Tokoro, Sci. Rep., 2016, 6, 27212 CrossRef CAS PubMed.
- J. L. García-Muñoz, A. Romaguera, F. Fauth, J. Nogués and M. Gich, Chem. Mater., 2017, 29, 9705–9713 CrossRef.
- K. T. Chan, J. R. Morales, Y. Kodera and J. E. Garay, J. Mater. Chem. C, 2017, 5, 7911–7918 RSC.
- V. Ukleev, S. Suturin, T. Nakajima, T. Arima, T. Saerbeck, T. Hanashima, A. Sitnikova, D. Kirilenko, N. Yakovlev and N. Sokolov, Sci. Rep., 2018, 8, 8741 CrossRef PubMed.
- J. A. Sans, V. Monteseguro, G. Garbarino, M. Gich, V. Cerantola, V. Cuartero, M. Monte, T. Irifune, A. Muñoz and C. Popescu, Nat. Commun., 2018, 9, 4554 CrossRef CAS.
- S. Ohkoshi, K. Imoto, A. Namai, M. Yoshikiyo, S. Miyashita, H. Qiu, S. Kimoto, K. Kato and M. Nakajima, J. Am. Chem. Soc., 2019, 141, 1775–1780 CrossRef CAS PubMed.
- J. Yuan, A. Balk, H. Guo, Q. Fang, S. Patel, X. Zhao, T. Terlier, D. Natelson, S. Crooker and J. Lou, Nano Lett., 2019, 19, 3777–3781 CrossRef CAS PubMed.
- J. López-Sánchez, A. Serrano, A. del Campo, M. Abuín, E. Salas-Colera, A. Muñoz-Noval, G. R. Castro, J. de la Figuera, J. F. Marco, P. Marín, N. Carmona and O. Rodríguez de la Fuente, RSC Adv., 2019, 9, 17571–17580 RSC.
- J. Ma, Y. Wang and K. Chen, Adv. Powder Technol., 2019, 30, 3021–3027 CrossRef CAS.
- H. Tokoro, J. Fukui, K. Watanabe, M. Yoshikiyo, A. Namai and S. Ohkoshi, RSC Adv., 2020, 10, 39611–39616 RSC.
- Y. Gu, M. Yoshikiyo, A. Namai, D. Bonvin, A. Martinez, R. Piñol, P. Téllez, N. J. O. Silva, F. Ahrentorp, C. Johansson, J. Marco-Brualla, R. Moreno-Loshuertos, P. Fernández-Silva, Y. Cui, S. Ohkoshi and A. Millán, RSC Adv., 2020, 10, 28786–28797 RSC.
- S. Ohkoshi, M. Yoshikiyo, K. Imoto, K. Nakagawa, A. Namai, H. Tokoro, Y. Yahagi, K. Takeuchi, F. Jia, S. Miyashita, M. Nakajima, H. Qiu, K. Kato, T. Yamaoka, M. Shirata, K. Naoi, K. Yagishita and H. Doshita, Adv. Mater., 2020, 32, 2004897 CrossRef CAS PubMed.
- Y. Kusano, H. Nakata, Z. Peng, R. S. S. Maki, T. Ogawa and M. Fukuhara, ACS Appl. Mater. Interfaces, 2021, 13, 38491–38498 CrossRef CAS PubMed.
- Y. Gu, N. J. O. Silva, M. Yoshikiyo, A. Namai, R. Piñol, G. Maurin-Pasturel, Y. Cui, S. Ohkoshi, A. Millán and A. Martínez, Chem. Commun., 2021, 57, 2285–2288 RSC.
- A. Philip, Y. Zhou, G. C. Tewari, S. van Dijken and M. Karppinen, J. Mater. Chem. C, 2022, 10, 294–300 RSC.
- H. Tokoro, K. Nakabayashi, S. Nagashima, Q. Song, M. Yoshikiyo and S. Ohkoshi, Bull. Chem. Soc. Jpn., 2022, 95, 538–552 CrossRef CAS.
- E. Gorbachev, M. Soshnikov, M. Wu, L. Alyabyeva, D. Myakishev, E. Kozlyakova, V. Lebedev, E. Anokhin, B. Gorshunov, O. Brylev, P. Kazin and L. Trusov, J. Mater. Chem. C, 2021, 9, 6173–6179 RSC.
- A. Namai, S. Sakurai, M. Nakajima, T. Suemoto, K. Matsumoto, M. Goto, S. Sasaki and S. Ohkoshi, J. Am. Chem. Soc., 2009, 131, 1170–1173 CrossRef CAS PubMed.
- A. Namai, M. Yoshikiyo and S. Ohkoshi, IEEE Magn. Lett., 2016, 7, 5506704 Search PubMed.
- Y. Hamasaki, T. Shimizu, S. Yasui, T. Shiraishi, A. Akama, T. Kiguchi, T. Taniyama and M. Itoh, J. Appl. Phys., 2017, 122, 015301 CrossRef.
- L. Corbellini, C. Lacroix, D. Ménard and A. Pignolet, Scr. Mater., 2017, 140, 63–66 CrossRef CAS.
- K. Knížek, M. Pashchenko, P. Levinský, O. Kaman, J. Houdková, P. Jiříček, J. Hejtmánek, M. Soroka and J. Buršík, J. Appl. Phys., 2018, 124, 213904 CrossRef.
- L. Kubíčková, O. Kaman, P. Veverka, V. Herynek, P. Brázda, M. Vosmanská, T. Kmječ, P. Dvořák, D. Kubániová and J. Kohout, Colloids Surf., A, 2020, 589, 124423 CrossRef.
- S. Ohkoshi, S. Kuroki, S. Sakurai, K. Matsumoto, K. Sato and S. Sasaki, Angew. Chem., Int. Ed., 2007, 46, 8392–8395 CrossRef CAS PubMed.
- M. Nakajima, A. Namai, S. Ohkoshi and T. Suemoto, Opt. Express, 2010, 18, 18260–18268 CrossRef CAS PubMed.
- T. Katayama, S. Yasui, Y. Hamasaki and M. Itoh, Appl. Phys. Lett., 2017, 110, 212905 CrossRef.
- L. Kubíčková, O. Kaman, P. Veverka, V. Herynek, P. Brázda, K. Bernášek, M. Veverka and J. Kohout, J. Alloys Compd., 2021, 856, 158187 CrossRef.
- S. Sakurai, S. Kuroki, H. Tokoro, K. Hashimoto and S. Ohkoshi, Adv. Funct. Mater., 2007, 17, 2278–2282 CrossRef CAS.
- M. Yoshikiyo, A. Namai, M. Nakajima, K. Yamaguchi, T. Suemoto and S. Ohkoshi, J. Appl. Phys., 2014, 115, 172613 CrossRef.
- A. Namai, M. Yoshikiyo, K. Yamada, S. Sakurai, T. Goto, T. Yoshida, T. Miyazaki, M. Nakajima, T. Suemoto, H. Tokoro and S. Ohkoshi, Nat. Commun., 2012, 3, 1035 CrossRef PubMed.
- A. Namai, M. Yoshikiyo, S. Umeda, T. Yoshida, T. Miyazaki, M. Nakajima, K. Yamaguchi, T. Suemoto and S. Ohkoshi, J. Mater. Chem. C, 2013, 1, 5200–5206 RSC.
- S. Ohkoshi, K. Imoto, A. Namai, S. Anan, M. Yoshikiyo and H. Tokoro, J. Am. Chem. Soc., 2017, 139, 13268–13271 CrossRef CAS.
- S. Yasui, T. Katayama, T. Osakabe, Y. Hamasaki, T. Taniyama and M. Itoh, J. Ceram. Soc. Jpn., 2019, 127, 474–477 CrossRef CAS.
- S. Tsukamoto, Y. Oki, K. Imoto, A. Namai, M. Yoshikiyo and S. Ohkoshi, Advanced Photonics Research, 2022, 3, 2100319 CrossRef.
- P. Goyal and N. K. Prasad, IEEE Trans. Magn., 2016, 52, 2300906 Search PubMed.
- I. Ahamed, R. Skomski and A. Kashyap, AIP Adv., 2019, 9, 035231 CrossRef.
- Z. Ma, A. Romaguera, F. Fauth, J. Herrero-Martín, J. L. García-Muñoz and M. Gich, J. Magn. Magn. Mater., 2020, 506, 166764 CrossRef CAS.
- M. Polášková, O. Malina, J. Tuček and P. Jakubec, Nanoscale, 2022, 14, 5501–5513 RSC.
- T. Katayama, S. Yasui, Y. Hamasaki, T. Osakabe and M. Itoh, J. Mater. Chem. C, 2017, 5, 12597–12601 RSC.
- A. Namai, K. Ogata, M. Yoshikiyo and S. Ohkoshi, Bull. Chem. Soc. Jpn., 2020, 93, 20–25 CrossRef CAS.
- R. Kinugawa, K. Imoto, Y. Futakawa, S. Shimizu, R. Fujiwara, M. Yoshikiyo, A. Namai and S. Ohkoshi, Adv. Eng. Mater., 2021, 23, 2001473 CrossRef CAS.
- M. Yoshikiyo, Y. Futakawa, R. Shimoharai, Y. Ikeda, J. MacDougall, A. Namai and S. Ohkoshi, Chem. Phys. Lett., 2022, 803, 139821 CrossRef CAS.
- M. Gich, C. Frontera, A. Roig, E. Taboada, E. Molins, H. R. Rechenberg, J. D. Ardisson, W. A. A. Macedo, C. Ritter, V. Hardy, J. Sort, V. Skumryev and J. Nogués, Chem. Mater., 2006, 18, 3889–3897 CrossRef CAS.
- S. Ohkoshi, A. Namai and S. Sakurai, J. Phys. Chem. C, 2009, 113, 11235–11238 CrossRef CAS.
- J. Tucek, S. Ohkoshi and R. Zboril, Appl. Phys. Lett., 2011, 99, 253108 CrossRef.
- M. Yoshikiyo, K. Yamada, A. Namai and S. Ohkoshi, J. Phys. Chem. C, 2012, 116, 8688–8691 CrossRef CAS.
- I. Ahamed, N. Seriani, R. Gebauer and A. Kashyap, RSC Adv., 2020, 10, 27474–27480 RSC.
- Y. V. Knyazev, A. I. Chumakov, A. A. Dubrovskiy, S. V. Semenov, I. Sergueev, S. S. Yakushkin, V. L. Kirillov, O. N. Martyanov and D. A. Balaev, Phys. Rev. B, 2020, 101, 094408 CrossRef CAS.
|
This journal is © The Royal Society of Chemistry 2022 |