DOI:
10.1039/D2RA02403A
(Paper)
RSC Adv., 2022,
12, 22197-22201
Effect of water-soluble fullerenes on macrophage surface ultrastructure revealed by scanning ion conductance microscopy†
Received
14th April 2022
, Accepted 31st July 2022
First published on 10th August 2022
Abstract
C60-fullerenes have unique potential in antiviral, drug delivery, photodynamic therapy and other biomedical applications. However, little is known about their effects on macrophage surface morphology and ultrastructure. Here by using contact-free scanning ion conductance microscopy (SICM), we investigated the effects of two water-soluble fullerenes on the surface ultrastructure and function of macrophages. The results showed that these fullerenes would be a promising phagocytosis inhibitor and SICM would be an excellent tool to study the morphological information of adhesive and fragile samples.
Introduction
C60-fullerene was discovered by Richard Smalley, Robert Curl and Harold Kroto in 1985 and it is a novel carbon allotrope with a spherical structure.1,2 During the last few years, due to their unique physical structure and chemical properties,3,4 C60-fullerenes and their derivatives have been considered important ingredients for cosmetics and photoelectric materials, and they also have many advantages and potential in the field of biomedicine, such as antiviral, drug delivery and photodynamic therapy applications.5–8 As an important molecule widely used in various biological activities, evaluating its cytotoxicity and biocompatibility to the immune system has become a very meaningful research topic in recent years.9,10 Up to now, research on cytotoxicity and biocompatibility of some C60-fullerenes mainly focused on detecting the release of inflammatory cytokines and cell viability in cultured macrophages.11–13 For example, Park et al. found that carbon fullerenes (C60s) could induce an increase in pro-inflammatory cytokines such as interleukin-1, tumor necrosis factor-α and interleukin-6 in the lung of mice.14 Sayes et al. found that water-soluble nano-C60 colloidal suspension could disrupt normal cellular function through lipid peroxidation.15 However, little is known about effects of C60-fullerenes and its derivatives on the surface morphology and ultrastructural changes of macrophages. In addition, changes in cell morphology and surface ultrastructure are not only a reflection of the cytotoxicity and biocompatibility of nanomaterials, but also some cellular functions, e.g., the phagocytosis of macrophage. For example, we can know whether the macrophage have an inflammatory reaction from its surface morphology,16,17 and also it has been proved that cytoskeletal rearrangement and morphological changes can affect the phagocytic function of macrophages.18,19
The morphology of macrophages can be studied by using different imaging techniques, such as optical microscopy, atomic force microscopy (AFM), electron microscopy, etc. Due to the optical diffraction limit of traditional optical microscopy, the most common methods to study the surface ultrastructure of macrophage in nanoscale are AFM and electron microscopy. For example, AFM imaging was used to reveal the distinct features of macrophage ultrastructure for the different stages of phagocytosis against cancer cells.20 Shvedova et al. studied the ultrastructural and morphological changes of human epidermal keratinocytes after treated with single-wall carbon nanotubes (SWCNTs) by scanning electron microscopy (SEM) and transmission electron microscopy (TEM),21 Fiorito et al. revealed the changes of macrophage surface morphology after being treated with C60-fullerenes and SWCNTs by SEM.17 However, because of the contact scanning mode of AFM and extremely severe imaging conditions of electron microscopy, such as high vacuum conditions, electron beam excitation and freeze-dehydrated sample preparation, they all have potential damage to the surface ultrastructure of biological samples.22 Therefore, a contact-free imaging technology with nanoscale resolution under physiological conditions is needed. Scanning ion conductance microscopy (SICM), an excellent combination of scanning probe microscopy (SPM) and patch-clamp technique, has been invented since 1989.23 In the past few years, owing to the continuous improvements in the feedback control system, SICM has emerged as a powerful tool to image and analyze fragile, adhesive or sensitive surfaces, such as living cell membrane, with nanometer scale resolution.24–27 For example, SICM can be used for long-term microvilli dynamics imaging with high image quality while AFM cannot.28 Novak et al. tracked the dynamic interactions between individual carboxyl-modified nanoparticles and cell membrane structures at nanoscale using a high-speed SICM.29 In addition, SICM also was used to evaluate the cytotoxicity and efficiency of many nanomaterials as drug delivery carriers by imaging the detailed cellular morphological information, e.g., Lee et al. studied the morphological changes of A549 cells after treatment with doxorubicin-loaded DNA-wrapped halloysite nanotubes (HDD), they found that the actin filaments were disrupted into separated dot structures because of the release of doxorubicin from HDD.30 Therefore, the contact-free SICM imaging technology provides an excellent method for studying the effects of fullerenes on the morphology and ultrastructure of macrophages under physiological conditions. In this work, we studied the surface ultrastructure of THP-1 macrophages before and after polyhydroxylated (C60–OH) or cationic (C60–Por) water-soluble fullerenes treatment by SICM under physiological conditions. Results indicate that the water-soluble fullerenes can affect the phagocytic function of macrophages by affecting their surface ultrastructure.
Results and discussion
Changes in macrophage surface ultrastructure such as membrane ruffles are closely related to macropinocytosis and phagocytosis, which in turn affect macrophage uptake and destruction of microbial pathogens. Therefore, it is important to use a non-contact and non-invasive method to characterize the adhesive and fragile ultrastructure on the surface of macrophages.31 Here, for the first time, we imaged the surface ultrastructure of THP-1 differentiated macrophage using the contact-free SICM at nanoscale resolution under physiological conditions. Fig. 1A shows the schematic diagram of the SICM device with a nanopipette filled and immersed in physiological buffer above a biological sample. It is noted that THP-1 cells were incubated with PMA for 72 hours to induce differentiation and attachment before SICM imaging. By imaging the whole cell in a large scale, the cell surface exhibited many blurred structural details (Fig. 2A). Further small-scale imaging showed that there were many flat sheet-like membrane ruffles and a few circular ruffles on the dorsal membrane (Fig. 2B). As might be expected, almost all the membrane ruffles in SICM imaging appeared more intact while part of them seemed fractured in SEM imaging.17,32 Therefore, studying the intact forms of these ruffles by contact-free SICM imaging might contribute to improving our understanding of phagocytosis and macropinocytosis mechanism.
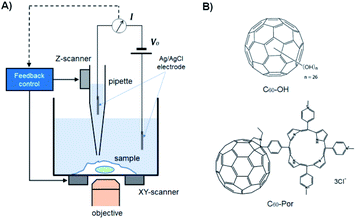 |
| Fig. 1 (A) Schematic diagram of the SICM device with a nanopipette filled and immersed in physiological buffer above a biological sample. (B) Molecular structure of the hydroxyl and porphyrin-modified water-soluble fullerenes used in this study. | |
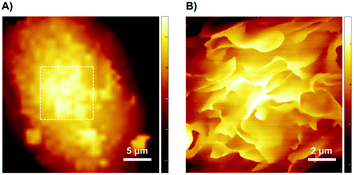 |
| Fig. 2 SICM imaging of THP-1 differentiated macrophage surface ultrastructure. (A) The cell surface morphology of a large scale. (B) Further small-scale imaging showed the height topography on the dorsal membrane of macrophage. Region: 10 × 10 μm, pixel: 256 × 256. | |
In view of the great potential of C60-fullerene derivatives in biomedical research, and the close relationship between macrophage surface morphology and cell function.33,34 Therefore, investigating the effects of C60-fullerene derivatives on the macrophage surface morphology and ultrastructure is of great significance. Here we observed the ultrastructure changes of THP-1 macrophage by SICM imaging after 48 hours of treatment with water-soluble fullerenes C60–OH or C60–Por (the molecular structures of the two are shown in Fig. 1B, and their hydration diameter was characterized by dynamic light scattering in ESI Fig. S1†). As shown in Fig. 3A and B, both the cells treated with 0.01 mg mL−1 C60–OH or C60–Por showed surface actin cytoskeleton rearrangement significantly (a higher concentration of 0.1 mg mL−1 was also studied in the ESI Fig. S2†). The sheet-like membrane ruffles become smaller and reduced after treated with C60–OH, but more circular ruffles and blebs emerged. Contrary to these changes, the sheet-like ruffles become thickening and increased after C60–Por treatment. In addition, we analyzed the length distribution of the membrane ruffles before and after water-soluble C60-fullerenes treatment (Fig. 3C). The length of the membrane ruffles is about 7 μm before C60-fullerenes treatment, but reduced to about 5 μm after C60–Por treatment and about 2.5 μm after C60–OH treatment. Taking these significant changes into account, we performed a cytotoxicity assay on the two water-soluble C60-fullerenes. We evaluated the cytotoxicity of C60–OH and C60–Por against THP-1 macrophage by Cell Counting Kit-8 (CCK-8) assay. The result showed that more than 90% of the cells survived, so these two water-soluble C60-fullerenes did not cause any statistically significant cytotoxicity (Fig. 3D). It is noted that the treatment concentrations and incubation times of water-soluble C60-fullerenes in this study were based on the results of our previous study.35,36 Therefore, the significant changes of surface membrane ruffles might be attributed to the activation of phagocytosis, rather than the cytotoxicity of C60–OH and C60–Por.
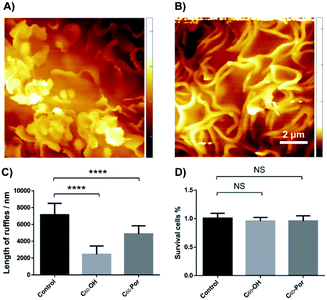 |
| Fig. 3 The surface topography of THP-1 macrophages treated with different water-soluble C60-fullerenes. (A) C60–OH treatment. (B) C60–Por treatment. (C) Length distribution of the surface membrane ruffles after different treatment. (D) Cytotoxicity of different C60-fullerenes. | |
To study whether these surface actin cytoskeleton rearrangements were reversible or not, we removed the C60-fullerenes and kept the THP-1 macrophages continuing to be cultured in normal medium for 48 hours. The SICM imaging showed that both the membrane ruffles could restore basically (Fig. 4). The surface membrane ruffles after treated with C60–OH restored to large and sheet-like (Fig. 4A). As shown in Fig. 4B, the membrane ruffles of C60–Por treated macrophages restored to sheet-like and showed a dramatic decrease.
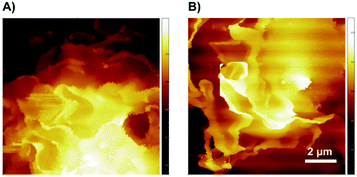 |
| Fig. 4 Recoveries of the macrophage surface ultrastructure after different water-soluble C60-fullerenes treatment. (A) Recovery after C60–OH treatment. (B) Recovery after C60–Por treatment. Region: 10 × 10 μm, pixel: 128 × 128. | |
Since the intracellular cytoskeleton plays an equally important role in the regulation of macropinocytosis and phagocytosis as well as surface ultrastructure, here we study whether the C60–OH and C60–Por fullerenes affect cell morphology through cytoskeletal fluorescence imaging. As shown in Fig. 5, the distribution of F-actin, stained by rhodamine phalloidin, was examined by a laser scanning confocal fluorescence microscope. Before treated with water-soluble C60-fullerenes, PMA-differentiated THP-1 macrophages were adherent growth and they showed a spindle or polygonal morphology (Fig. 5A). The spindle or polygonal morphology could be mainly attributed to the important role of actin cytoskeleton. After C60–OH or C60–Por treatment, although both the cells were still adherent growth, most of them turned round (Fig. 5B and D). It heralded the rearrangement of the macrophage actin cytoskeleton. Then we removed the C60-fullerenes and continued to culture the THP-1 macrophages in normal medium for 48 hours. Most of them restored to spindle or polygonal morphology and its surface ruffles have a certain degree of recovery (Fig. 5C and E). During this process, although we can see some changes in surface folds, these changes are not as pronounced compared to SICM imaging.
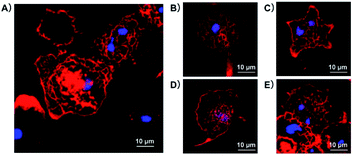 |
| Fig. 5 Morphological changes of THP-1 macrophages after different water-soluble C60-fullerenes treatment by using a confocal microscope. (A) Control. (B) Treat with C60–OH. (C) Recovery after C60–OH treatment. (D) Treat with C60–Por. (E) Recovery after C60–Por treatment. It is noted that the red actin was stained by rhodamine phalloidin, the cell nucleus was stained by DAPI. | |
As mentioned above, the cellular morphology and surface ultrastructure of THP-1 macrophages changed significantly after water-soluble C60–OH or C60–Por treatment, and these changes were closely related to phagocytic function. Therefore, studying the changes of macrophage phagocytosis before and after C60-fullerenes treatment is beneficial to understand the impact on its immune function. In our experiment, THP-1 macrophages were exposed to fluorescent beads with a diameter of 2 μm overnight after C60–OH or C60–Por treatment (Fig. 6A). The total fluorescence intensity of individual cells was then analyzed, and the statistical results showed that both C60–OH and C60–Por treatments significantly reduced phagocytosis (Fig. 6B).
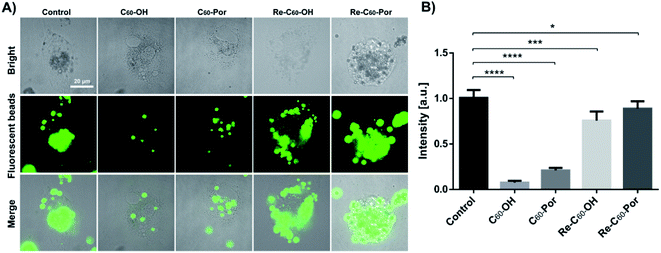 |
| Fig. 6 The phagocytosis of THP-1 macrophage before and after treated with water-soluble fullerenes. (A) From left to right are the control, C60–OH treatment, C60–Por treatment, and recovery from C60–OH and C60–Por treatment respectively. The top row shows the bright field of THP-1 macrophages under different treatments. The middle row is the confocal images of 2 μm diameter fluorescent beads engulfed by macrophage. The bottom row is the overlap of bright fields and fluorescent fields. Scale bar, 20 μm. (B) The histograms show the corresponding average fluorescence intensity of about 30 cells each group. | |
In view of the above results found that the surface ultrastructure and morphology have been restored to a certain extent, here we want to know whether the phagocytic function has been restored or not. THP-1 macrophages continued to be cultured in normal medium for 48 hours after C60-fullerenes treatments, and then exposed to fluorescent beads overnight (Fig. 6A). The statistical results showed that the phagocytosis of both is increased noticeably (Fig. 6B). This is consistent with the previous results that the cellular morphology and surface ultrastructure cannot be fully restored. Therefore, we speculate that these two water-soluble fullerenes affect phagocytosis by affecting the rearrangement of surface membrane ruffles and intracellular actin cytoskeleton.
Conclusions
In conclusion, considering the wide application of fullerene derivatives in biomedicine, we hope to study their effects on the immune system by characterizing the relationship between the surface ultrastructure and function of immune cells. In this work, by using the contact-free SICM, we achieved the surface ultrastructure of macrophage under physiological conditions for the first time. Compared with the previous electron microscopy imaging results, we observed a lot of more intact sheet-like membrane ruffles. In addition, we found that the sheet-like surface membrane ruffles appeared varying degrees of shortening and thickening after treatment with C60–OH or C60–Por. And these changes could be restored basically when we removed the C60-fullerenes derivatives and continued to culture in normal medium for 48 hours. Given that the membrane ruffles are shortened to varying degrees and the fluorescence intensity of F-actin on the membrane surface decreases after C60–OH and C60–Por treatment, we believe that the fullerenes induce cell membrane ruffle rearrangement by reducing F-actin aggregation or inhibiting F-actin protein expression. Further confocal imaging showed that most of the macrophage morphology turned round and the phagocytosis reduced significantly. Based on the negligible cytotoxicity of C60–OH and C60–Por, we could surmise that these two water-soluble C60-fullerenes affected the phagocytosis of macrophage by affecting the surface membrane ruffles and intracellular actin cytoskeleton. And these effects were reversible to a certain degree.
Given the fact that membrane ruffles serve as a critical role in the initial capture of particulate targets prior to phagocytosis or micropinocytosis for activated macrophages, the membrane ruffle rearrangement is instrumental for the uptake of both soluble and particulate antigen by macrophages.37,38 Therefore, C60–OH and C60–Por would be a promising inhibitor of phagocytosis in studying the endocytic mechanism. And this work also demonstrated that the contact-free SICM would be an excellent tool to study the morphological information of adhesive and fragile materials.
Conflicts of interest
There are no conflicts to declare.
Acknowledgements
This work was supported by the National Natural Science Foundation of China (21735006, 32000873, 22077124 and 91939301); the China Postdoctoral Science Foundation (2019M650718); the Chinese Academy of Sciences.
Notes and references
- H. W. Kroto, J. R. Heath, S. C. O'Brien, R. F. Curl and R. E. Smalley, Nature, 1985, 318, 162–163 CrossRef CAS.
- H. W. Kroto, A. Allaf and S. Balm, Chem. Rev., 1991, 91, 1213–1235 CrossRef CAS.
- D. M. Guldi and M. Prato, Acc. Chem. Res., 2000, 33, 695–703 CrossRef CAS PubMed.
- M. Mikawa, H. Kato, M. Okumura, M. Narazaki, Y. Kanazawa, N. Miwa and H. Shinohara, Bioconjugate Chem., 2001, 12, 510–514 CrossRef CAS PubMed.
- O. A. Kraevaya, A. V. Novikov, A. F. Shestakov, E. S. Ershova, E. A. Savinova, L. V. Kameneva, N. N. Veiko, D. Schols, J. Balzarini, S. V. Kostyuk and P. A. Troshin, Chem. Commun., 2020, 56, 10203–10206 RSC.
- H. Ma, X. Zhang, Y. Yang, S. Li, J. Huo, Y. Liu, M. Guan, M. Zhen, C. Shu, J. Li and C. Wang, Langmuir, 2021, 37, 2740–2748 CrossRef CAS PubMed.
- M. D. Esrafili and A. A. Khan, RSC Adv., 2022, 12, 3948–3956 RSC.
- X. Zhang, W. Zhou, Y. Liu, L. Jin, J. Huo, Y. Yang, S. Li, H. Ma, J. Li, M. Zhen, J. Li and C. Wang, Nano Res., 2022, 15, 3346–3355 CrossRef CAS.
- C. M. Sayes, J. D. Fortner, W. Guo, D. Lyon, A. M. Boyd, K. D. Ausman, Y. J. Tao, B. Sitharaman, L. J. Wilson and J. B. Hughes, Nano Lett., 2004, 4, 1881–1887 CrossRef CAS.
- Y. Morimoto, M. Horie, N. Kobayashi, N. Shinohara and M. Shimada, Acc. Chem. Res., 2012, 46, 770–781 CrossRef PubMed.
- S. Fiorito, A. Serafino, F. Andreola, A. Togna and G. Togna, J. Nanosci. Nanotechnol., 2006, 6, 591–599 CrossRef CAS PubMed.
- S. T. Stern and S. E. McNeil, Toxicol. Sci., 2008, 101, 4–21 CrossRef CAS PubMed.
- M. Lehto, T. Karilainen, T. Róg, O. Cramariuc, E. Vanhala, J. Tornaeus, H. Taberman, J. Jänis, H. Alenius and I. Vattulainen, PLoS One, 2014, 9, e114490 CrossRef PubMed.
- E.-J. Park, H. Kim, Y. Kim, J. Yi, K. Choi and K. Park, Toxicol. Appl. Pharmacol., 2010, 244, 226–233 CrossRef CAS PubMed.
- C. M. Sayes, A. M. Gobin, K. D. Ausman, J. Mendez, J. L. West and V. L. Colvin, Biomaterials, 2005, 26, 7587–7595 CrossRef CAS PubMed.
- S. Yamago, H. Tokuyama, E. Nakamura, K. Kikuchi, S. Kananishi, K. Sueki, H. Nakahara, S. Enomoto and F. Ambe, Chem. Biol., 1995, 2, 385–389 CrossRef CAS PubMed.
- S. Fiorito, A. Serafino, F. Andreola and P. Bernier, Carbon, 2006, 44, 1100–1105 CrossRef CAS.
- K. Ohsawa, Y. Imai, H. Kanazawa, Y. Sasaki and S. Kohsaka, J. Cell Sci., 2000, 113, 3073–3084 CrossRef CAS PubMed.
- M. Szabo, K. Dulka and K. Gulya, Brain Res. Bull., 2016, 120, 41–57 CrossRef CAS PubMed.
- M. Li, L. Liu, N. Xi, Y. Wang, X. Xiao and W. Zhang, Langmuir, 2014, 30, 1609–1621 CrossRef CAS PubMed.
- A. Shvedova, V. Castranova, E. Kisin, D. Schwegler-Berry, A. Murray, V. Gandelsman, A. Maynard and P. Baron, J. Toxicol. Environ. Health, Part A, 2003, 66, 1909–1926 CrossRef CAS PubMed.
- R. Tantra and A. Knight, Nanotoxicology, 2011, 5, 381–392 CrossRef CAS PubMed.
- P. Hansma, B. Drake, O. Marti, S. Gould and C. Prater, Science, 1989, 243, 641 CrossRef CAS PubMed.
- Y. E. Korchev, C. L. Bashford, M. Milovanovic, I. Vodyanoy and M. J. Lab, Biophys. J., 1997, 73, 653–658 CrossRef CAS PubMed.
- A. I. Shevchuk, G. I. Frolenkov, D. Sánchez, P. S. James, N. Freedman, M. J. Lab, R. Jones, D. Klenerman and Y. E. Korchev, Angew. Chem., 2006, 118, 2270–2274 CrossRef.
- P. Novak, C. Li, A. I. Shevchuk, R. Stepanyan, M. Caldwell, S. Hughes, T. G. Smart, J. Gorelik, V. P. Ostanin and M. J. Lab, Nat. Methods, 2009, 6, 279–281 CrossRef CAS PubMed.
- V. O. Nikolaev, A. Moshkov, A. R. Lyon, M. Miragoli, P. Novak, H. Paur, M. J. Lohse, Y. E. Korchev, S. E. Harding and J. Gorelik, Science, 2010, 327, 1653–1657 CrossRef CAS PubMed.
- J. Seifert, J. Rheinlaender, P. Novak, Y. E. Korchev and T. E. Schäffer, Langmuir, 2015, 31, 6807–6813 CrossRef CAS PubMed.
- P. Novak, A. Shevchuk, P. Ruenraroengsak, M. Miragoli, A. J. Thorley, D. Klenerman, M. J. Lab, T. D. Tetley, J. Gorelik and Y. E. Korchev, Nano Lett., 2014, 14, 1202–1207 CrossRef CAS PubMed.
- Y. Lee, G.-E. Jung, S. J. Cho, K. E. Geckeler and H. Fuchs, Nanoscale, 2013, 5, 8577–8585 RSC.
- J. A. Swanson, Nat. Rev. Mol. Cell Biol., 2008, 9, 639–649 CrossRef CAS PubMed.
- H. R. Petty, D. G. Hafeman and H. M. McCONNELL, J. Cell Biol., 1981, 89, 223–229 CrossRef CAS PubMed.
- T. Da Ros and M. Prato, Chem. Commun., 1999, 8, 663–669 RSC.
- S. Ghrebi, D. W. Hamilton, J. Douglas Waterfield and D. M. Brunette, J. Biomed. Mater. Res., Part A, 2013, 101, 2118–2128 CrossRef PubMed.
- C. Zhou, Q. Liu, W. Xu, C. Wang and X. Fang, Chem. Commun., 2011, 47, 2982–2984 RSC.
- S. Dang, Q. Liu, X. Zhang, K. He, C. Wang and X. Fang, J. Nanosci. Nanotechnol., 2012, 12, 4478–4484 CrossRef CAS PubMed.
- R. S. Flannagan, R. E. Harrison, C. M. Yip, K. Jaqaman and S. Grinstein, J. Cell Biol., 2010, 191, 1205–1218 CrossRef CAS PubMed.
- D. Schlam and J. Canton, Small GTPases, 2017, 8, 65–70 CrossRef CAS PubMed.
|
This journal is © The Royal Society of Chemistry 2022 |
Click here to see how this site uses Cookies. View our privacy policy here.