DOI:
10.1039/D2RA02211G
(Paper)
RSC Adv., 2022,
12, 14912-14916
Ruthenium-catalyzed asymmetric hydrogenation of aromatic and heteroaromatic ketones using cinchona alkaloid-derived NNP ligands†
Received
5th April 2022
, Accepted 9th May 2022
First published on 18th May 2022
Abstract
A series of cinchona alkaloid-based NNP ligands, including a new one, have been employed for the asymmetric hydrogenation of ketones. By combining ruthenium complexes, various aromatic and heteroaromatic ketones were smoothly reacted, yielding valuable chiral alcohols with extremely high 99.9% ee. Moreover, a proposed reaction mechanism was discussed and verified by NMR.
Since the well-known failure of using racemic thalidomide, attention has been paid to the manufacture of optically pure compounds as effective components in pharmaceuticals and agrochemicals. Asymmetric hydrogenation of ketones, especially heteroaromatic ketones, has emerged as a popular facile route to approach enantiopure secondary alcohols as essential intermediates for the construction of biologically active molecules.1–4 Knowles et al.5 pioneered the production of enantioenriched chiral compounds in 1968, and Noyori and co-workers6–8 laid the cornerstone of asymmetric hydrogenation in 1990s. Subsequently, numerous catalytic systems have been developed. Ru-BICP-chiral diamine-KOH was developed and proved to be effective for asymmetric hydrogenation of aromatic ketones by Xumu Zhang.9 Cheng-yi Chen reported asymmetric hydrogenation of ketone using trans-RuCl2[(R)-xylbinap][(R)-daipen] and afforded secondary alcohol in 92–99% ee.10 Mark J. Burk and Antonio Zanotti-Gerosa disclosed Phanephos-ruthenium-diamine complexes catalyzing the asymmetric hydrogenation of aromatic and heteroaromatic ketones with high activity and excellent enantioselectivity.11 Qi-Lin Zhou et al. designed and synthesized chiral spiro diphosphines as a new chiral scaffold applied in the asymmetric hydrogenation of simple ketones with extremely high activity and up to 99.5% ee.12–15 Similarly, Kitamura and co-workers have developed a set of tridentate binan-Py-PPh2 ligands for the asymmetric hydrogenation of ketones affording excellent results.16 Recently, chiral diphosphines and tridentate ligands based on ferrocene have been developed for the asymmetric hydrogenation of carbonyl compound with a remarkable degree of success.17–21 Despite many ligands for asymmetric hydrogenation of ketones have been reported, expensive reagent and multistep complicated reactions were employed to synthesize most of them.22–24 In light of increasing industrial demand, easily obtained, cheap and practical chiral ligands are still highly desirable. In addition to chiral ligands, the selection of metals was essential for asymmetric hydrogenation.25–27 Although Mn,28–30 Fe,31–34 Co,35–37 Ni38,39 and Cu40,41 metals were proved to be effective for asymmetric hydrogenation in recent years, Rh,42–44 Ir45,46 and especially Ru remained the most preferred metals. Ruthenium47–51 was chosen owing to its superior performances in terms of low price, selectivity and activity. Takeshi Ohkuma,52 Hanmin Huang53,54 and Johannes G. de Vries55 all successfully used ruthenium catalysts for asymmetric hydrogenation of ketones. Admittedly, there is a continuing interest in the development of cheaper, simpler and more efficient catalysts for the asymmetric hydrogenation of ketones under mild conditions to access corresponding secondary alcohols. Recently, we developed new NNP chiral ligands derived from cinchona alkaloid for the asymmetric hydrogenation of various ketones in extremely excellent results using a iridium catalytic system.56 Prompted by these encouraging results, we were interested in exploring a ruthenium-catalyzed asymmetric hydrogenation of ketones with NNP chiral ligands derived from cinchona alkaloid. Here, we showed that changing from iridium to ruthenium, with the same simple synthetic ligands, delivered a catalyst catalyzed asymmetric hydrogenation of ketones to give the industrially important chiral alcohols with up to 99.9% ee. Although the catalytic activity of ruthenium catalyst was not as high as that of the iridium catalyst, the enantioselectivity could be maintained, and even showed higher enantioselectivity in the hydrogenation of some substrates.
Chiral tridentate ligand NNP (L1–L10) were synthesized and characterized as reported in our previous publication. With tridentate ligands in hand, we began to evaluate the catalytic performance in benzylidene-bis(tricyclohexylphosphine) dichlororuthenium-catalyzed asymmetric hydrogenation of acetophenone employed as a standard substrate (Table 1). Initially, asymmetric hydrogenation of acetophenone catalyzed by NNP L5 was carried out in different solvents such as MeOH, EtOH, n-PrOH, i-PrOH and n-butanol (Fig. 2). MeOH was found to be a better one as the conversion and enantioselectivity were 99.9% and 98.2%, respectively. Bases screening showed that Ba(OH)2 was superior to the others, giving >99.9% conversion and 98.8% ee in the present catalytic system (Fig. 1). Ligand screening revealed that the configuration of chiral centers of cinchona alkaloids of the ligand markedly affected the catalytic performance. NNP ligands derived from cinchonine and quinidine appeared to benefit both the reaction rate and enantioselectivity, while those derived from cinchonidine and quinine had the opposite effect. Further, different NNP ligands that bearing different substituents on the phenyl rings were evaluated. Similar to our previous research, ligands with electron-withdonating substituents showed better catalytic performance than those with electron-withdrawing substituents. However, it was noted that the more electron-withdonating substituents furnished lower activity but same enantioselectivity. The optimal ligand L5 derived from quinidine with one methoxy group on benzene ring provided the corresponding chiral alcohol with 99.9% conversion and 98.8% ee. Considering that L3 derived from cinchonine had similar catalytic performance to L4 derived from quinidine, new ligand L10 similar to L5 with one methoxy group on benzene ring was synthesized and applied to the asymmetric hydrogenation of template substrate. 99.6% conversion and 97.6% ee was obtained. Hence, L5 was employed as better ligand in subsequent experiments.
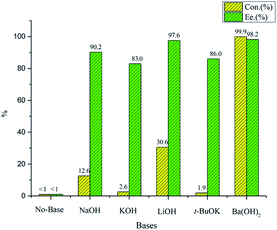 |
| Fig. 1 The effect of different bases for the asymmetric hydrogenation of acetophenone (substrate/Ru/L5 = 500/1/2, ketones: 0.429 mol L−1, base: 0.15 mol L−1, MeOH: 2 mL, 30 °C, 6 MPa, 2 h.). | |
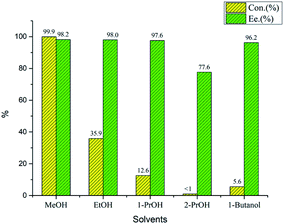 |
| Fig. 2 The effect of different solvents for the asymmetric hydrogenation of acetophenone. (substrate/Ru/L5 = 1000/1/2, ketones: 0.858 mol L−1, Ba(OH)2: 0.15 mol L−1, solvent: 2 mL, 30 °C, 6 MPa, 2 h.). | |
In order to evaluate the general applicability of this method, we have surveyed the substrate scope. As can be discerned from the data in Fig. 3, most of aryl alkyl ketones P1–P21 were hydrogenated with very high enantioselectivities (97.1–99.9% ee). Under the conditions employed, the electron effect and steric hindrance seemed to have no significant impact on the enantioselectivities of asymmetric hydrogenation. However, the activities were slightly affected by steric hindrance, especially ortho-substituted group. Significantly, Ru/L5 showed high enantioselectivity 98.2% in the hydrogenation of [3,5-bis(trifluoromethyl)phenyl]ethanone and its corresponding enantiopure alcohol P21 was key chiral intermediates for the NK-1 receptor antagonist aprepitant.57,58 Additionally, chiral heteroaromatic alcohols containing nitrogen, oxygen or sulfur in the heterocyclic ring were considerable organic synthetic intermediate in pharmaceuticals and organic synthesis.59–61 Nevertheless, due to the coordination ability of the heteroaromatic moiety, the asymmetric hydrogenation of heteroaromatic ketones has been less investigated. Surprisingly, the protocol was found to be very effective for asymmetric hydrogenation of various heteroaromatic ketones P22–P35. The substrates were all well reduced smoothly to afford the corresponding chiral alcohol with 97.1–99.9% ee. Notably, meta- and para-acetyl pyridines, generally as a challenging hydrogenation substrates62–64 owe to stronger coordination ability, were also hydrogenated with up to 97.2% ee (P33 and P34). Gratifyingly, 97.4 ee was obtained when acetonaphthone employed (P36). Benzo-fused seven-membered cyclic ketone proceeded well to afford the corresponding chiral alcohols with 99.6% ee (P37). To further explore substrate scope, we checked the effectiveness of method for asymmetric hydrogenation of unsaturated ketones. Although, both substrates were hydrogenated with high yield, only medium enantioselectivity 73.8 and 78.3% ee were given, respectively.
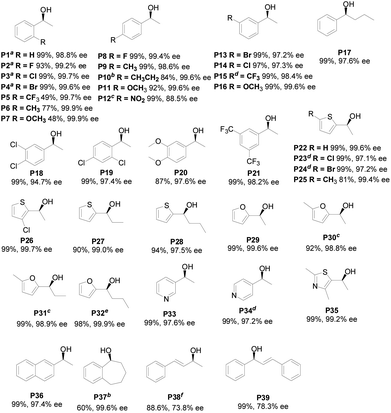 |
| Fig. 3 Asymmetric hydrogenation of ketones catalyzed by Ru/L5. (Substrate/Ru/L5 = 200/1/2, ketones: 0.171 mol L−1, Ba(OH)2: 0.15 mol L−1, MeOH: 2 mL, 30 °C, 6 MPa, 2 h, isolated yield, ee was determined by GC or HPLC on chiral stationary phase (see the ESI†); asubstrate/Ru/L5 = 2000/1/2; bsubstrate/Ru/L5 = 100/1/2, 25 °C; csubstrate/Ru/L5 = 50/1/2, 25 °C, 24 h; dsubstrate/Ru/L5 = 25 °C; esubstrate/Ru/L5 = 50/1/2, 4 h; fEtOH). | |
Table 1 The effect of different ligand for the asymmetric hydrogenation of acetophenonea
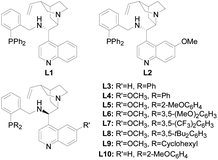
|
Entry |
Ligands |
Con./% |
ee/% |
Config |
Substrate/Ru/L = 2000/1/2, ketones: 1.715 mol L−1, Ba(OH)2: 0.15 mol L−1, MeOH: 2 mL, 30 °C, 6 MPa, 2 h. |
1 |
L1 |
47.5 |
78.2 |
R |
2 |
L2 |
56.1 |
77.8 |
R |
3 |
L3 |
>99 |
94.0 |
S |
4 |
L4 |
80.8 |
97.0 |
S |
5 |
L5 |
>99 |
98.8 |
S |
6 |
L6 |
54.2 |
98.0 |
S |
7 |
L7 |
2.1 |
84.2 |
S |
8 |
L8 |
91.1 |
98.0 |
S |
9 |
L9 |
36.5 |
92.8 |
S |
10 |
L10 |
>99 |
97.6 |
S |
To understand the mechanism of the reaction, NMR was introduced to investigated active species. Single peak at δ = 19.91 ppm belonging to phenyl vinyl group of the complex disappeared in the 1H NMR spectrum when the complex was mixed with the ligand (Fig. S1, ESI†). In the meantime, 31P NMR spectrum of the mixture exhibited new singlet at δ = 55.71 ppm (s) with the signal of complex disappearing (Fig. S2, ESI†). These maybe indicated the formation of ruthenium complex A. Subsequently, a new weak signal was generated in the 31P NMR spectrum with the introduction of hydrogen and base (Fig. S3, ESI†). These may indicate the formation of ruthenium hydride complexes. Meanwhile, the 1H NMR spectrum exhibited several weak signals below 0 ppm (Fig. S4, ESI†). These data also verified the formation of ruthenium hydride complexes. Reference to relevant literature,65–67 the proposed catalytic cycle for the asymmetric hydrogenation of ketones with the ruthenium complex was shown in Scheme 1. First, the ruthenium complex reacted with ligands to form complex A. In the presence of base and hydrogen, the complex A lost two chlorine atoms to transform into dihydride complex B. Then, a hydridic Ru–H and a protic N–H unit were transferred from dihydride B to the carbonyl group of the ketones through the transition state TS to produce chiral alcohol. And the ruthenium complex lost two hydrogen atoms to form complex C. Finally, dihydride B was regenerated in hydrogen atmosphere. Compared with the reported iridium catalytic system with the same chiral ligands, the hydrogenation activity of the ruthenium catalytic system decreased significantly although maintained high enantioselectivity. The result indicates that the selection of metals was as important as chiral ligands for asymmetric hydrogenation.
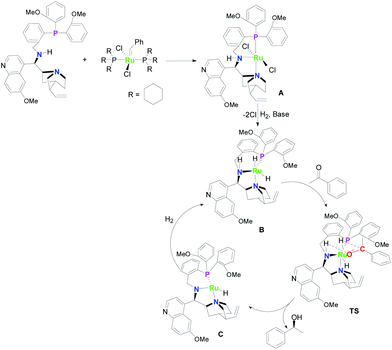 |
| Scheme 1 Proposed mechanism for the asymmetric hydrogenation. | |
Conclusions
Herein, we reported the cinchona alkaloid-based NNP ligands for the asymmetric hydrogenation of ketones. Through the optimization of steric and electronic properties of NNP ligands, L5 derived from quinidine with a methoxy group on benzene ring was proved to be conducive to prepare chiral alcohols. Most of substrates, even heteroaromatic ketone, were converted to corresponding chiral alcohols in excellent enantioselectivities. Moreover, NMR was introduced to investigated active species and some new weak signals were detected. Based on the excellent results achieved in asymmetric hydrogenation, we will continue to exploring the application range of NNP ligands toward a broad implementation in asymmetric catalysis.
Author contributions
Chun Li and Lin Zhang contributed to the conception and design of the experiments. Ling Zhang and Qian Chen performed the experiments and analyzed the data. Linlin Li and Jian Jiang synthesized several ligands and substrates. Hao Sun carried out the GC and HPLC analysis of product. Li Li and Ting Liu guided instrumental analysis. Chun Li directed the project. Chun Li and Lin Zhang co-wrote the manuscript.
Conflicts of interest
There are no conflicts of interest to declare.
Acknowledgements
Research reported in this publication was supported by the National Natural Science Foundation of China (21601039, 21562010) and Guizhou Provincial Science and Technology Projects (ZK[2022]358).
Notes and references
- H. Ooka, N. Arai, K. Azuma, N. Kurono and T. Ohkuma, J. Org. Chem., 2008, 73, 9084 CrossRef CAS PubMed.
- W. Li, X. F. Sun, L. Zhou, G. H. Hou, S. C. Yu and X. M. Zhang, J. Org. Chem., 2009, 74, 1397 CrossRef CAS PubMed.
- T. Ohkuma, M. Koizumi, M. Yoshida and R. Noyori, Org. Lett., 2000, 2, 1749 CrossRef CAS PubMed.
- S. Lühr, J. Holz and A. Börner, ChemCatChem, 2011, 3, 1708 CrossRef.
- W. S. Knowles and M. J. Sabacky, Chem. Commun., 1968, 1445 RSC.
- T. Ohkuma, H. Ooka, S. Hashiguchi, T. Ikariya and R. Noyori, J. Am. Chem. Soc., 1995, 117, 2675 CrossRef CAS.
- H. Doucet, T. Ohkuma, K. Murata, T. Yokozawa, M. Kozawa, E. Katayama, F. Anthony, T. Ikariya and R. Noyori, Angew. Chem., Int. Ed., 1998, 37, 1703 CrossRef CAS PubMed.
- T. Ohkuma, M. Koizumi, H. Doucet, T. Pham, M. Kozawa, K. Murata, E. Katayama, T. Yokozawa, T. Ikariya and R. Noyori, J. Am. Chem. Soc., 1998, 120, 13529 CrossRef CAS.
- P. Cao and X. M. Zhang, J. Org. Chem., 1999, 64, 2127 CrossRef CAS PubMed.
- C. Y. Chen, R. A. Reamer, J. R. Chilenski and C. J. McWilliams, Org. Lett., 2003, 5, 5039 CrossRef CAS.
- M. J. Burk, W. Hems, D. Herzberg, C. Malan and A. Zanotti-Gerosa, Org. Lett., 2000, 2, 4173 CrossRef CAS PubMed.
- J. H. Xie, L. X. Wang, Y. Fu, S. F. Zhu, B. M. Fan, H. F. Duan and Q. L. Zhou, J. Am. Chem. Soc., 2003, 125, 4404 CrossRef CAS PubMed.
- J. B. Xie, J. H. Xie, X. Y. Liu, W. L. Kong, S. Li and Q. L. Zhou, J. Am. Chem. Soc., 2010, 132, 4538 CrossRef CAS PubMed.
- J. B. Xie, J. H. Xie, X. L. Liu, Q. Q. Zhang and Q. L. Zhou, Chem.–Asian J., 2011, 6, 899 CrossRef CAS PubMed.
- F. H. Zhang, F. J. Zhang, M. L. Li, J. H. Xie and Q. L. Zhou, Nat. Catal., 2020, 3, 621 CrossRef CAS.
- T. Yamamura, H. Nakatsuka, S. Tanaka and M. Kitamura, Angew Chem. Int. Ed., 2013, 52, 9313 CrossRef CAS PubMed.
- Z. Q. Liang, T. L. Yang, G. X. Gu, L. Dang and X. M. Zhang, Chin. J. Chem., 2018, 36, 851 CrossRef CAS.
- C. Qin, C. J. Hou, H. Z. Liu, Y. J. Liu, D. Z. Huang and X. P. Hu, Tetrahedron Lett., 2018, 59, 719 CrossRef CAS.
- W. L. Wu, S. D. Liu, M. Duan, X. F. Tan, C. Y. Chen, Y. Xie, Y. Lan, X. Q. Dong and X. M. Zhang, Org. Lett., 2016, 18, 2938 CrossRef CAS.
- J. F. Yu, M. Duan, W. L. Wu, X. T. Qi, P. Xue, Y. Lan, X. Q. Dong and X. M. Zhang, Chem.–Eur. J., 2016, 23, 970 CrossRef.
- J. F. Yu, J. Long, Y. H. Yang, W. L. Wu, P. Xue, L. W. Chung, X. Q. Dong and X. M. Zhang, Org. Lett., 2017, 19, 690 CrossRef CAS PubMed.
- R. H. Morris, Chem. Soc. Rev., 2009, 38, 2282 RSC.
- A. Bartoszewicz, N. Ahlsten and B. Martín-Matute, Chem.–Eur. J., 2013, 19, 7274 CrossRef CAS PubMed.
- R. Malacea, R. Poli and E. Manoury, Coord. Chem. Rev., 2010, 254, 729 CrossRef CAS.
- W. Tang and X. M. Zhang, Chem. Rev., 2003, 103, 3029 CrossRef CAS.
- J. H. Xie and Q. L. Zhou, Acc. Chem. Res., 2008, 41, 581 CrossRef CAS PubMed.
- M. Garbe, Z. H. Wei, B. Tannert, A. Spannenberg, H. J. Jiao, S. Bachmann, M. Scalone, K. Junge and M. Beller, Adv. Synth. Catal., 2019, 361, 1913 CrossRef CAS.
- L. L. Zhang, Y. T. Tang, Z. B. Han and K. L. Ding, Angew. Chem., Int. Ed., 2019, 58, 4973 CrossRef CAS PubMed.
- L. Y. Zeng, H. X. Yang, M. L. Zhao, J. L. Wen, J. H. R. Tucker and X. M. Zhang, ACS Catal., 2020, 10, 13794 CrossRef CAS.
- R. Huber, A. Passera and A. Mezzetti, Organometallics, 2018, 37, 396 CrossRef CAS.
- Y. Y. Li, S. L. Yu, X. F. Wu, J. L. Xiao, W. Y. Shen, Z. R. Dong and J. X. Gao, J. Am. Chem. Soc., 2014, 136, 4031 CrossRef CAS PubMed.
- R. Hodgkinson, A. D. Grosso, G. Clarkson and M. Wills, Dalton Trans., 2016, 45, 3992 RSC.
- P. O. Lagaditis, P. E. Sues, J. F. Sonnenberg, K. Y. Wan, A. J. Lough and R. H. Morris, J. Am. Chem. Soc., 2014, 136, 1367 CrossRef CAS PubMed.
- S. A. M. Smith, P. O. Lagaditis, A. Lüpke, A. J. Lough and R. H. Morris, Chem.–Eur. J., 2017, 23, 7212 CrossRef CAS.
- M. R. Friedfeld, M. Shelvin, J. M. Hoyt, S. W. Krska, M. T. Tudge and P. J. Chirik, Science, 2013, 342, 1076 CrossRef CAS.
- M. R. Friedfeld, H. Zhong, R. T. Ruck, M. Shelvin and P. J. Chirik, Science, 2018, 360, 888 CrossRef CAS PubMed.
- J. H. Chen, C. H. Chen, C. L. Ji and Z. Lu, Org. Lett., 2016, 18, 1594 CrossRef CAS PubMed.
- Y. Hamada, Y. Koseki, T. Fujii, T. Maeda, T. Hibino and K. Makino, Chem. Commun., 2008, 6206 RSC.
- T. Hibino, K. Makino, T. Sugiyama and Y. Hamada, ChemCatChem, 2009, 1, 237 CrossRef CAS.
- H. Shimizu, D. Igarashi, W. Kuriyama, Y. Yusa, N. Sayo and T. Saito, Org. Lett., 2007, 9, 1655 CrossRef CAS PubMed.
- K. Junge, B. Wendt, D. Addis, S. Zhou, S. Das, S. Fleischer and M. Beller, Chem.–Eur. J., 2011, 17, 101 CrossRef CAS.
- D. Liu and X. M. Zhang, Eur. J. Org. Chem., 2005, 646 CrossRef CAS.
- H. L. Yang, N. N. Huo, P. Yang, H. Pei, H. Lv and X. M. Zhang, Org. Lett., 2015, 17, 4144 CrossRef CAS.
- W. J. Zhang, S. H. Ruan, W. Y. Shen, Z. Wang, D. L. An, Y. Y. Li and J. X. Gao, Catal. Commun., 2019, 119, 153 CrossRef CAS.
- Z. R. Dong, Y. Y. Li, J. S. Chen, B. Z. Li, Y. Xing and J. X. Gao, Org. Lett., 2005, 7, 1043 CrossRef CAS PubMed.
- F. Ling, S. F. Nian, J. C. Chen, W. J. Luo, Z. Wang, Y. P. Lv and W. H. Zhong, J. Org. Chem., 2018, 83, 10749 CrossRef CAS PubMed.
- M. M. Sheeba, M. M. Tamizh, L. J. Farrugia, A. Endo and R. Karvembu, Organometallics, 2014, 33, 540 CrossRef CAS.
- W. Y. Shen, Y. Y. Li, Z. R. Dong and J. X. Gao, Synthesis, 2009, 14, 2413 Search PubMed.
- D. E. Karakaş, M. Aydemir, F. Durap and A. Baysal, Inorg. Chim. Acta, 2018, 471, 430 CrossRef.
- Y. Yuki, T. Touge, H. Nara, K. Matsumura, M. Fujiwhara, Y. Kayaki and T. Ikariya, Adv. Synth. Catal., 2017, 359, 1 CrossRef.
- A. D. Grosso, G. J. Clarkson and M. Wills, Inorg. Chim. Acta, 2019, 496, 119043 CrossRef.
- N. Utsumi, N. Arai, K. Kawaguchi, T. Katayama, T. Yasuda, K. Murata and T. Ohkuma, ChemCatChem, 2018, 10, 3955 CrossRef CAS.
- H. Zhou and H. . , M. Huang, ChemCatChem, 2013, 5, 2253 CrossRef CAS.
- Q. M. Zhu, D. J. Shi, C. G. Xia and H. M. Huang, Chem.–Eur. J., 2011, 17, 7760 CrossRef CAS PubMed.
- M. Cettolin, P. Puylaert, L. Pignataro, S. Hinze, C. Gennari and J. G. de Vries, ChemCatChem, 2017, 9, 3125 CrossRef CAS.
- L. Zhang, L. Zhang, Q. Chen, L. Li, J. Jiang, H. Sun, C. Zhao, Y. Yang and C. Li, Org. Lett., 2022, 24, 415 CrossRef CAS PubMed.
- D. Alsafadi, S. Alsalman and F. Paradisi, Org. Biomol. Chem., 2017, 15, 9169 RSC.
- A. Li, L. Ye, X. Yang, C. Yang, J. Gu and H. Yu, Chem. Commun., 2016, 52, 6284 RSC.
- E. Buitrago, H. Lundberg, H. Andersson, P. Ryberg and H. Adolfsson, ChemCatChem, 2012, 4, 2082 CrossRef CAS.
- S. W. Krabbe, M. A. Hatcher, R. K. Bowman, M. B. Mitchell, M. S. McClure and J. S. Johnson, Org. Lett., 2013, 15, 4560 CrossRef CAS PubMed.
- D. Liu, W. Gao, C. Wang and X. Zhang, Angew. Chem., Int. Ed., 2005, 44, 1687 CrossRef CAS PubMed.
- M. Coll, O. Pàmies and M. Diéguez, Adv. Synth. Catal., 2014, 356, 2293 CrossRef CAS.
- J. Margalef, T. Slagbrand, F. Tinnis, H. Adolfsson, M. Diéguez and O. Pàmies, Adv. Synth. Catal., 2016, 358, 4006 CrossRef CAS.
- J. Wu, J.-X. Ji, R. Guo, C.-H. Yeung and A. S. C. Chan, Chem.–Eur. J., 2003, 9, 2963 CrossRef CAS.
- K. Abdur-Rashid, S. E. Clapham, A. Hadzovic, J. N. Harvey, A. J. Lough and R. H. Morris, J. Am. Chem. Soc., 2002, 124, 15104 CrossRef CAS PubMed.
- R. Abbel, K. Abdur-Rashid, M. Faatz, A. Hadzovic, A. J. Lough and R. H. Morris, J. Am. Chem. Soc., 2005, 127, 1870 CrossRef CAS PubMed.
- M. Zimmer-De Iuliis and R. H. Morris, J. Am. Chem. Soc., 2009, 131, 11263 CrossRef CAS PubMed.
|
This journal is © The Royal Society of Chemistry 2022 |