DOI:
10.1039/D2RA02165J
(Paper)
RSC Adv., 2022,
12, 15420-15426
Research on the stability of luminescence of CsPbBr3 and Mn:CsPbBr3 PQDs in polar solution
Received
4th April 2022
, Accepted 15th April 2022
First published on 20th May 2022
Abstract
Mn:CsPbBr3 PQDs are achieved by hot injection method. As the amount of Mn doping is gradually increased, the photoluminescence (PL) spectra shows a slight blue shift. Mn-doped PQDs exhibit higher quantum efficiency of 83.9% and longer lifetimes of 267 ns. The stability test was performed to assess the susceptibility of the PQDs to polar solutions. It was figured out that although the stabilities of CsPbBr3 PQDs and Mn-doped PQDs decreased as the polarity of solution increased, Mn-doped PQDs still maintained higher PL intensity than undoped PQD. Notably, 73% PL intensity of Mn:PQDs was maintained which is nearly three times as much as undoped PQDs in water. We found polarity would induce drastic degradation of CsPbBr3 QDs. The steady-state spectroscopy, transmission electron microscopy (TEM), and X-ray diffraction (XRD) verified that CsPbBr3 QDs tend to aggregate to form larger particles under continuous light soaking. Our work reveals the main origin of instability in CsPbBr3 QDs and provides reference to engineering such QDs towards optimal device application.
1. Introduction
The low cost, high photoluminescence quantum yields (PLQYs), and narrow and adjustable emission spectra of perovskite quantum dots (PQDs) make them attractive emitting materials for illumination and display applications.1–4 In recent years, CsPbX3 PQDs (X = Cl, Br, I) have attracted a great deal of attention from materials scientists as promising optoelectronic materials.5–10 Compared to organic–inorganic hybrid perovskite materials, all-inorganic perovskites have the advantages of simple preparation and good stability.11–16
Despite plenty of advantages, the toxic nature of Pb and limited tolerance of all inorganic perovskites to moisture, ultraviolet (UV) light and the adjacent environment, cannot be ignored.17–22 It is very urgent to figure out some approaches to solve these the worrying issues. CsPbX3 can be doped with heterogeneous metal ions to replace Pb2+, thus reducing the amount of Pb.23–26 Researchers also figured out that the doped ions can bring about new electric, optical, and magnetic properties.27–30 Kim et al. reported Ni2+-doped CsPbBr3 PQDs in 2021. The stability of the Ni:CsPbBr3 PQDs with distinct Ni/Pb ratios against moisture and UV light was tested. Doping with Ni2+ improved the stability of PQDs. Further, the Ni:CsPbBr3 PQDs exhibited compatibility with real-world applications under harsh conditions.31 Zhou et al. successfully doped Zn2+ ions into CsPbBr3 PQDs by ligand-assisted reprecipitation method, exhibiting an 85% enhancement of the PLQYs. In addition, the optimized energy level alignment via Zn doping facilitates the carrier balance in the devices, improving the efficiencies. The obtained CsPbBr3:Zn-based PeLED reaches a high luminance of 3124 cd m−2 and a maximum external quantum efficiency of 0.85%, which are excellent among those of CsPbBr3-based PeLED. The results demonstrate that Zn doping significantly enhances the performance of PeLED, which increases the potential of these inverted PeLEDs connected with n-type TFTs towards practical applications.32 Among them, Mn2+ is an important choice, which can not only reduce Pb content to a certain extent but also reduce photon recycling, improve PLQYs, and expand luminous color gamut. An orange luminescence band due to the d–d transition of Mn2+ is introduced in addition to the intrinsic band-edge excitonic fluorescence. As well established previously, the efficient energy transfer from PQDs to Mn2+ only occurs when the Mn2+ content is high enough.33,34 Mn2+ doping brings many benefits to PQDs, however, it's regrettable that the stability of Mn-doped CsPbBr3 in polar solutions hasn't been studied in preceding researches, which is critical to its application in various conditions.
In this work, we successfully synthesized partially Mn-doped CsPbBr3 by hot injection technique.35–42 Then we demonstrate the Mn:CsPbMnBr3 PQDs with a Mn substitution ratio up to 38%. The introduction of Mn2+ significantly increased the formation energy of Pb, Br, and Cs vacancies.43,44 The PL lifespan was longer with Mn2+ doping, indicating the inhibition of non-radioactive recombination upon doping.45 Besides the reduction of lead content, the as-prepared PQDs maintain the orthorhombic crystalline structure of the CsPbBr3 host and exhibit strong PL emission of Pb-based PQDs.46–49 Mn2+ doping enhances the PLQYs of CsPbBr3 from 52.6% to 83.9%. Finally, three polar solvents of acetone, N,N-dimethylformamide (DMF), water, were selected to analyze the stabilities of CsPbBr3 and Mn:CsPbBr3. According to our experimental results, the Mn-doped CsPbBr3 is superior to its undoped counterpart in terms of stability in polar solutions.
2. Results and discussion
2.1. Structure and composition of Mn-doped PQDs
Mn:CsPbBr3 PQDs with different Mn2+ doping concentrations were prepared by the hot injection method reported previously. Five samples with Mn
:
Pb ratios are 0
:
1, 1
:
1, 2.5
:
1, 3.5
:
1 and 5
:
1, TEM images of the resulting Mn:CsPbBr3 PQDs are shown in Fig. 1a–e, respectively. Five samples with Mn/Pb ratios of 0, 1.25, 2.5, 3.75, and 5 exhibited lattice constants of 5.78 Å, 5.73 Å, 5.65 Å, 5.59 Å, and 5.56 Å, respectively. All the prepared samples exhibited excellent crystallinity, as observed in the highresolution (HR-TEM) image, and the inter-planar distance of the (100) plane decreased from 5.77 Å to 5.56 Å depending on the feed of MnBr2. The lattice shrinkage of the Mn2+ iondoped CsPbBr3 PNCs is mainly due to the synergistic effect of replacing the larger Pb2+ ions (1.19 Å) with smaller Mn2+ ions (0.67 Å). The corresponding HRTEM images of the Mn:CsPbBr3 PQDs are shown in Fig. 1a–e. Elemental mapping and energy dispersive X-ray spectroscopy (EDS) (Fig. 1f) were performed for a typical Mn
:
Pb ratio of 2.5 for Mn-doped CsPbBr3 PQDs. To confirm the integration of guest Mn2+ cations, the chemical composition of Mn:CsPbBr3 PQDs (Mn
:
Pb = 2.5) was analyzed by EDS. The elements Cs, Pb, Mn, and Br were identified in Mn:CsPbBr3 PQDs. The four target elements were evenly distributed throughout the selection region, indicating successful synthesis of Mn:CsPbBr3 PQDs and effective doping of Mn2+.
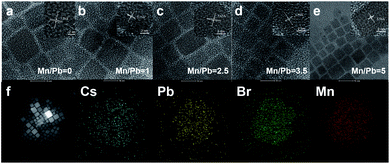 |
| Fig. 1 (a) TEM images of CsPbBr3 and (b)–(e) Mn:CsPbBr3PQDs (Mn : Pb = 1 to 5). (f) Elemental mapping images of the Mn:CsPbBr3 PQDs (Mn : Pb = 2.5). | |
Fig. 2a shows the XPS results, which depict the change in binding energy according to the doping element. The XPS spectrum reveals signals of the Cs, Pb, Br, and Mn elements in the Mn-doped CsPbBr3 PQDs, and the corresponding high-resolution XPS spectrum shows a typical Mn 2P signal (Fig. 2b).
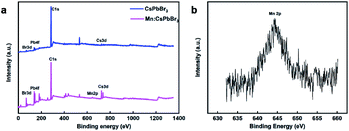 |
| Fig. 2 (a) XPS of Mn:CsPbBr3PQDs (Mn : Pb = 2.5). (b) High-resolution XPS spectra for Mn 2p of Mn:CsPbBr3 PQDs. | |
Selected area electron diffraction (SAED) patterns of the Mn:CsPbBr3 also shows the presence of tetragonal (100) and (200) surfaces (Fig. 3a), confirming that the perovskite structure has not been destroyed. XRD patterns of the CsPbBr3 doped with different concentrations of Mn2+ are shown in Fig. 3b. With increased Mn doping, there was no overall structural change. The orthorhombic CsPbBr3 phase (PDF#18-0364, Fig. 3b) remained. The peak of (200) of the synthesized CsPbBr3 PNCs shifted by 0.23° to the right when the Mn2+ concentration increased, indicating that the amount of Mn2+ dopant in the CsPbBr3 PNCs increased. In addition, as the concentration of Mn increased, the intensity of the main peaks gradually increased. These results provide solid evidence for the efficient replacement of Mn2+ ions in the CsPbBr3 PNC lattice. EDS were conducted to analyze the actual compositions of the Mn:CsPbBr3 PQDs. When the feeding ratios are Mn
:
Pb = 1
:
1, Mn
:
Pb = 2.5
:
1, Mn
:
Pb = 3.5
:
1, and Mn
:
Pb = 5
:
1, the resulting products are CsPb0.961Mn0.039Br3, CsPb0.798Mn0.202Br3, CsPb0.729Mn0.271Br3, and CsPb0.616Mn0.384Br3, respectively (Table 1).
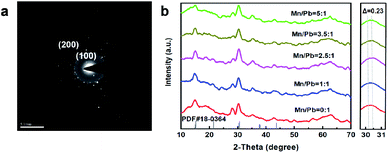 |
| Fig. 3 (a) SAED pattern of Mn-doped CsPbBr3 (Mn : Pb = 2.5). (b) XRD of Mn:CsPbBr3PQDs (Mn : Pb from 0 to 5). | |
Table 1 EDS of Mn:CsPbBr3PQDs (Mn
:
Pb from 1 to 5)
Mn : Pb |
1 : 1 |
2.5 : 1 |
3.5 : 1 |
5 : 1 |
Mn |
0.039 |
0.202 |
0.271 |
0.384 |
Pb |
0.961 |
0.798 |
0.729 |
0.616 |
2.2 Optical properties
Fig. 4 shows the absorbance graph according to the Mn doping concentration. The absorption measurement results demonstrated that light of wavelength 500–513 nm was absorbed by the PQDs. Due to the decreased size of Mn:CsPbBr3 PQDs, their UV-vis absorption spectra show a gradual blue shift with the increase Mn-to-Pb molar feed ratio. Since no new absorption band was observed after Mn doping, it is presumed that Mn doping has a negligible effect on the electronic structure of CsPbBr3 PQDs.
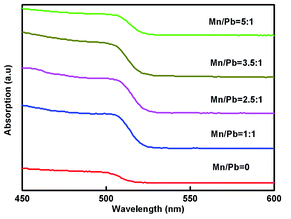 |
| Fig. 4 Absorption of CsPbBr3 and Mn:CsPbBr3 PQDs. | |
Fig. 5a manifests the PL measurements. 508–518 nm marked the wavelength band of emitted light. The average half width proved to be 20.4 nm, which revealed high color purity. With the increasing doping concentration, because of the quantum confinement effect, the emission wavelength was going through a blue shift from 518 to 508 nm. A change in the energy level resulting from doping concentration resulted in a reduction in emission wavelength at the rate of 3–10 nm compared with the undoped sample. The PL intensity was on a gradual increase when substituted concentration of Mn2+ ions was growing larger. Nonetheless, the others PL peaks (Mn
:
Pb = 3.5, 5) was decreasing. Particularly speaking, the PL peak intensity of the sample (Mn
:
Pb = 2.5), when compared with the intensity of the undoped sample, was increasing at the rate of 3.8 times. According to Fig. 5b, the gradual conversion color converted from green to blue; the most striking luminous intensity was shown with the Mn
:
Pb = 2.5. The highest quantum efficiency could be seen at an Mn
:
Pb of 2.5. The sample specialized the quantum efficiency was expressed at a doping ratio of 2.5 (Mn
:
Pb = 2.5, ≈83.9%), which differed by ≈27% with no doping (Mn
:
Pb = 0, ≈56.7%). For Mn
:
Pb = 3.5, however, the quantum efficiency decreased dramatically, indicating that the Mn2+ ion has a very strong effect upon the optical properties of PQDs. From the differences in defect levels offered by the doping material, a proper doping concentration able to gain high quantum efficiency can be attained. These results show that Mn2+ doping forms a Mn–Br ion pair to fill the Pb–Br ion vacancy, which effectively eliminates the non radiative recombination of excitons and improves the luminescence intensity of CsPbBr3 quantum dots. As the Mn doping increasing, Mn–Mn ion pair appears. This phenomenon is observed in Pan's work of Ni2+ ion-doped CsPbClxBr3−x PQDs.51
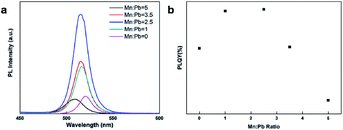 |
| Fig. 5 (a) PL peaks of pristine and Mn:CsPbBr3 PQDs with different Mn : Pb ratios; the insets show the corresponding normalized PL spectra. (b) PLQY with various Mn : Pb feed molar ratios of 0, 1, 2.5, 3.5, 5; the insets show the photograph of the Mn:CsPbBr3 PQDs in hexane solution under an irradiation of a 360 nm UV lamp. | |
The average lifetime for excitonic luminescence of CsPbBr3 PQDs added Mn2+ ions is observed to increase from 43.2 ns to 267.6 ns compared to undoped CsPbBr3 PQDs. The radiative and non-radiative decay rates can also be calculated using PLQY and the average life. The radiative rate decreased slightly from 0.0662 ns−1 to 0.0372 ns−1, and the non radiative recombination, which must be controlled for optical properties, significantly decreased from 0.0506 to 0.0077 ns−1. Therefore, the improvement in fluorescence performance can be attributed to the greatly reduced non-radiative attenuation of the incorporation of Mn:CsPbBr3 PQDs.
For acetone, the PL intensity of the initial reference sample after 2 h was 20% lower than the initial value (Fig. 7a). In contrast, the steady-state PL intensity at 2 h was 11.0%, lower than the initial value of Mn:CsPbBr3 PQDs (Fig. 6b). The decrease in the steady-state PL intensity of CsPbBr3 is more noticeably than that of Mn:CsPbBr3 PQDs in acetone, which indicates that Mn doping enhanced the stability of CsPbBr3 PQDs.
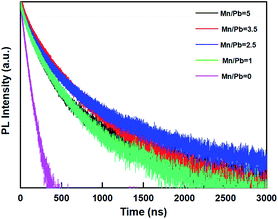 |
| Fig. 6 Time-resolved PL decays of fresh CsPbBr3 and Mn:CsPbBr3 PQDs. | |
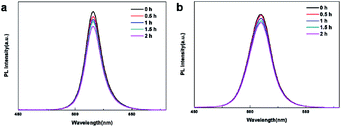 |
| Fig. 7 (a) PL spectra of the pristine CsPbBr3 and (b) Mn:CsPbBr3 PQDs (Mn : Pb = 2.5) recorded at different time intervals in acetone. | |
Fig. 8 shows stability of pristine CsPbBr3 and Mn:CsPbBr3 PQDs (Mn
:
Pb = 2.5) in DMF. The PL intensity of CsPbBr3 PQDs lower than 50% in DMF after 2 h. While PL intensity of Mn:CsPbBr3 PQDs maintain 76%. As the polarity improved, the enhancement of stability of Mn2+ doping is more pronounced.
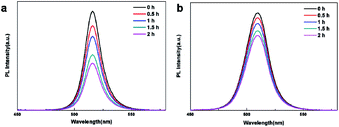 |
| Fig. 8 (a) PL spectra of the pristine CsPbBr3 and (b) Mn:CsPbBr3 PQDs (Mn : Pb = 2.5) recorded at different time intervals in DMF. | |
To investigate the water stability of the pre-synthesized Mn:CsPbBr3 PQDs, the PQD solution was injected with a 5% volume ratio of deionized water (DIW). For comparison, the measurements were performed for pristine CsPbBr3 PQDs at the same concentration as the Mn:CsPbBr3 PQD solution over time. A quantitative study was conducted by recording the PL evolution; the results are summarized in Fig. 9. The corresponding PL spectra of undoped CsPbBr3 PQDs and Mn:CsPbBr3 PQDs were documented at separate time intervals after the addition of DIW. For Mn:CsPbBr3 PQDs (Mn
:
Pb = 2.5), fluorescence quenching was comparatively slow and retained more than 73% of its initial performance, even over longer test periods. The PL spectra of the CsPbBr3 PQDs (Fig. 9a) and Mn:CsPbBr3 PQDs (Fig. 9b) based on the moisture test are shown. As shown in Fig. 9a, a small weakening of the emission intensity for Mn:CsPbBr3 PQDs was confirmed, but strong fluorescence was still observed. However, the CsPbBr3 PQDs lost majority of their brightness in just 1 min of testing, gradually changing fluorescent from green to colorless. The efficiency of CsPbBr3 PQDs decreased owing to the aggregation and decomposition of CsPbBr3 PQDs, and the optical properties of CsPbBr3 PQDs weakened as the half-width broadened. Based on the above results, we speculate that Mn2+ ions occupy the lead site, which makes the structure of PQDs more stable in polar solutions.
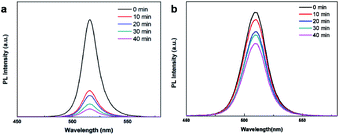 |
| Fig. 9 (a) PL spectra of the pristine CsPbBr3 and (b) Mn2+-substituted CsPbBr3 PQDs (Mn : Pb = 2.5) recorded at different time intervals in water. | |
3. Experimental section
3.1 Materials
All chemicals were used as received without further purification. Cesium carbonate (Cs2CO3, 99.9%, Sigma-Aldrich), oleic acid (OA, 90%, Sigma-Aldrich), 1-octadecene (ODE, 90%, Sigma-Aldrich), PbBr2 (99.9%, Maclin), MnBr2 (99.9%, Maclin), and oleylamine (OAm, 70%, Sigma-Aldrich).
3.2 Synthesis of Cs-oleate
Cs2CO3 (0.05 g), OA (0.5 mL), and ODE (10 mL) were placed in a 100 mL three-necked round-bottom flask. Next, the mixture was heated at 110 °C for 1 h under vacuum to completely remove moisture and oxygen. After that, the reaction temperature was maintained at 120 °C under N2 atmosphere and stirred until all Cs2CO3 reacted with OA to form Cs-oleate. A clear solution was obtained. Notably, Cs-oleate precipitated in the ODE at ambient temperature and had to be preheated to 100 °C before it could be used for the subsequent synthesis of PQDs.
3.3 Synthesis of CsPbX3PQDs
The synthesis method of CsPbX3 PQDs is similar to the approach of Protesescu et al.,50 with a few insignificant modifications. In brief, 10 mL ODE and 0.2 mmol PbBr2 were added to a 250 mL three-neck round-bottom flask and dried under vacuum at 110 °C for 1 h. The flask was purged with N2, and OA (0.5 mL) and OAm (0.5 mL) preheated to 70 °C was injected into the flask. The flask was heated at 120 °C until PbX2 completely reacted with OA and OAm. The temperature was then increased to 150 °C. The Cs-oleate precursor (0.8 mL) was quickly injected into the Pb-oleate. The solution was maintained at 150 °C for several seconds to allow the growth of the PQDs. The solution was cooled by soaking the flask in a cold-water bath.
3.4 Synthesis of Mn:CsPbX3PQDs
The synthesis of the Mn-doped CsPbX3 PQDs was performed in a manner similar to that of the CsPbX3 PQDs, as described above. ODE (5 mL) and PbBr2 were added to a 250 mL three-necked round-bottom flask and dried under vacuum at 110 °C for 1 h. All other steps remained the same.
3.5 Purification
The as-prepared CsPbX3PQD and Mn-doped CsPbX3PQD crude solutions were precipitated by centrifuging at 6000 rpm for 5 min. The colored supernatant was carefully discarded, and the precipitate was mixed with hexane (1.5 mL) using a vortex. The solution was centrifuged at 6000 rpm for 5 min. Finally, the PQDs were dispersed in hexane (1 mL) and centrifuged at 10
000 rpm for 2 min to remove any excess Cs-oleate, larger PQDs, and agglomerates. The precipitate was then collected and redispersed in hexane, resulting in a colloidal dispersion of the CsPbX3 PQDs.
3.6 Characterization
The size, morphology, and crystallinity of the nanoparticles were characterized by field-emission transmission electron microscopy (FE-TEM, JEOL, JEM-F200) in EDS. TEM images were obtained using high-resolution transmission electron microscopy (HR-TEM, FEI, Tecnai 20). The lattice constant was determined from the HR-TEM images using GATAN software. The absorption spectra were obtained using a UV-vis spectrophotometer (Varian, Cary 50). PL excitation and emission spectra were measured using a steady-state spectrofluorometer (PTI, QuantaMaster) with an excitation wavelength of 360 nm. XRD patterns were collected on a powder diffractometer (Bruker New D8-Advance) with monochromatized Cu Kα radiation (λ = 1.5418 Å). XPS was performed using a ULVAC-PHI X-TOOL instrument. PLQY was measured using a spectrofluorometer (JASCO, FP-8500) with an integrating sphere. Time-resolved PL experiments were performed using the TCSPC method. The samples in the solutions were excited using a 360 nm pulse (LDH-P-C-520, Picoquant). The time-resolved PL signals emitted from the samples were resolved using a monochromator and detected by a photomultiplier tube (PMT).
4. Conclusions
In this work, a hot-injection method help achieving CsPbBr3 perovskite QDs which possessing a high Mn-doping ratio. Consequently the properties of Pb-based perovskite QDs were improved. In order to gain their enhanced stability, different Mn
:
Pb ratio of CsPbBr3 PQDs structure was achieved. The Mn substitution ratio reaches 38%, which leads to an increase of 3.8 times of the steady-state PL intensity, the lifetime increase to 267 ns. In comparison to the undoped sample, the quantum efficiency achieved the improved as large of 30.6%. The stability of the Mn:CsPbBr3 PQDs with distinct Mn
:
Pb ratios against polar solutions was tested. Doping with Mn2+ improved the stability of PQDs according to our research.
Conflicts of interest
The authors state that they have no conflicts of interest to declare.
Acknowledgements
This work was supported by the National Natural Science Foundation of China (No. 21671044).
Notes and references
- X. Li, D. Yu, F. Cao, Y. Gu, Y. Wei, Y. Wu, J. Song and H. Zeng, Healing All-Inorganic Perovskite Films via Recyclable Dissolution–Recrystallization for Compact and Smooth Carrier Channels of Optoelectronic Devices with High Stability, Adv. Funct. Mater., 2016, 26, 5903–5912 CrossRef CAS.
- A. J. Nozik, Making the most of photons, Nanophotonics, 2009, 4, 548–549 CAS.
- J. Pan, L. N. Quan, Y. B. Zhao, W. Peng, B. Murali, S. P. Sarmah, M. J. Yuan, L. Sinatra, N. M. Alyami, J. K. Liu, E. Yassitepe, Z. Y. Yang, O. Voznyy, R. Comin, M. N. Hedhili, O. F. Mohammed, Z. H. Lu, D. H. Kim, E. H. Sargent and O. M. Bakr, Highly Efficient Perovskite-Quantum-Dot Light-Emitting Diodes by Surface Engineering, Adv. Mater., 2016, 28, 8718–8725 CrossRef CAS PubMed.
- Z. K. Tan, R. S. Moghaddam, M. L. Lai, P. Docampo, R. Higler, F. Deschler, M. Price, A. Sadhanala, L. M. Pazos, D. Credgington, F. Hanusch, T. Bein, H. J. Snaith and R. H. Friend, Bright light-emitting diodes based on organometal halide perovskite, Nat. Nanotechnol., 2014, 9, 687–692 CrossRef CAS PubMed.
- Q. A. Akkerman, V. D'Innocenzo, S. Accornero, A. Scarpellini, A. Petrozza, M. Prato and L. Manna, Tuning the Optical Properties of Cesium Lead Halide Perovskite Nanocrystals by Anion Exchange Reactions, J. Am. Chem. Soc., 2015, 137, 10276–10281 CrossRef CAS PubMed.
- W. C. He, Q. Q. Zhang, Y. F. Qi, J. Moore, P. Ray, N. Pradhan, X. C. Zhu, F. X. Han, T. Shahbazyan and Q. L. Dai, Synthesis and optical properties of doped CsPbCl3 nanocrystals, J. Nanopart. Res., 2021, 23, 219 CrossRef CAS.
- G. Nedelcu, L. Protesescu, S. Yakunin, M. I. Bodnarchuk, M. J. Grotevent and M. V. Kovalenko, Fast Anion-Exchange in Highly Luminescent Nanocrystals of Cesium Lead Halide Perovskites (CsPbX3, X = Cl, Br, I), Nano Lett., 2015, 15, 5635–5640 CrossRef CAS PubMed.
- X. L. Zhang, B. Xu, J. B. Zhang, Y. Gao, Y. J. Zheng, K. Wang and X. W. Sun, All-Inorganic Perovskite Nanocrystals for High-Efficiency Light Emitting Diodes: Dual-Phase CsPbBr3-CsPb2Br5 Composites, Adv. Funct. Mater., 2016, 26, 4595–4600 CrossRef CAS.
- Y. Z. Zheng, X. Yuan, J. Yang, Q. Y. Li, X. X. Yang, Y. Fan, H. B. Li, H. L. Liu and J. L. Zhao, Cu doping-enhanced emission efficiency of Mn2+ in cesium lead halide perovskite nanocrystals for efficient white light-emitting diodes, J. Lumin., 2020, 227, 117586 CrossRef CAS.
- S. H. Zhou, C. W. Zhou, J. Y. Zhu, H. Huang, F. Hu, Q. L. Ye, J. Q. Zhong, X. X. Yang and H. Y. Mao, Enhanced field emission properties of CsPbBr3 films by thermal annealing and surface functionalization with boron nitride, Appl. Surf. Sci., 2022, 578, 152116 CrossRef CAS.
- R. Yuan, Y. Cheng, S. Liu, L. Ding, H. Zhang, W. Xiang and X. Liang, Multicolour light-emitting diodes based on CsPbX3 (X = Br, I) quantum dots glasses solid materials, Mater. Lett., 2018, 229, 290–292 CrossRef CAS.
- R. Han, Q. Zhao, J. Su, X. Zhou, X. Ye, X. Liang, J. Li, H. Cai, J. Ni and J. Zhang, Role of Methyl Acetate in Highly Reproducible Efficient CsPbI3 Perovskite Quantum Dot Solar Cells, J. Phys. Chem. C, 2021, 125, 8469–8478 CrossRef CAS.
- G. Nedelcu, L. Protesescu, S. Yakunin, M. I. Bodnarchuk, M. J. Grotevent and M. V. Kovalenko, Fast Anion-Exchange in Highly Luminescent Nanocrystals of Cesium Lead Halide Perovskites (CsPbX3, X = Cl, Br, I), Nano Lett., 2015, 15, 5635–5640 CrossRef CAS PubMed.
- F. Yang, C. Wang, Y. Pan, X. Zhou, X. Kong and W. Ji, Surface stabilized cubic phase of CsPbI3 and CsPbBr3 at room temperature, Chin. Phys. B, 2019, 28, 056402 CrossRef CAS.
- J. Chen, D. Liu, M. J. Al-Marri, L. Nuuttila, H. Lehtivuori and K. Zheng, Photo-stability of CsPbBr3 perovskite quantum dots for optoelectronic application, Sci. China Mater., 2016, 59, 719–727 CrossRef CAS.
- H. W. Liu, Z. N. Wu, J. R. Shao, D. Yao, H. Gao, Y. Liu, W. L. Yu, H. Zhang and B. Yang, CsPbxMn1-xCl3 Perovskite Quantum Dots with High Mn Substitution Ratio, ACS Nano, 2017, 11, 2239–2247 CrossRef CAS PubMed.
- W. Yan, J. Shen, Y. Zhu, Y. Gong, J. Zhu, Z. Wen and C. Li, CsPbBr3 quantum dots photodetectors boosting carrier transport via molecular engineering strategy, Nano Res., 2021, 14, 4038–4045 CrossRef CAS.
- F. H. Ye, H. J. Zhang, W. Li, Y. Yan, J. L. Cai, R. S. Gurney, A. J. Pearson, D. Liu and T. Wang, Ligand-Exchange of Low-Temperature Synthesized CsPbBr3 Perovskite toward High-Efficiency Light-Emitting Diodes, Small Methods, 2019, 3, 3120–3126 CrossRef.
- Y. Ahmed, B. Khan, M. Bilal Faheem, K. Huang, Y. Gao and J. Yang, Organic additives in all-inorganic perovskite solar cells and modules: from moisture endurance to enhanced efficiency and operational stability, J. Energy Chem., 2022, 67, 361–390 CrossRef.
- J. K. Nam, S. U. Chai, W. Cha, Y. J. Choi, W. Kim, M. S. Jung, J. Kwon, D. Kim and J. H. Park, Potassium Incorporation for Enhanced Performance and Stability of Fully Inorganic Cesium Lead Halide Perovskite Solar Cells, Nano Lett., 2017, 17, 2028–2033 CrossRef CAS PubMed.
- Y. Gao, C. Luo, C. Yan, W. Li, C. A. Q. Liu and W. Q. Yang, Copper-doping defect-lowered perovskite nanosheets for deep-blue light-emitting diodes, J. Colloid Interface Sci., 2022, 607, 1796–1804 CrossRef CAS PubMed.
- F. Montanarella, K. M. McCall, K. Sakhatskyi, S. Yakunin, P. Trtik, C. Bernasconi, I. Cherniukh, D. Mannes, M. I. Bodnarchuk, M. Strobl, B. Walfort and M. V. Kovalenko, Highly Concentrated, Zwitterionic Ligand-Capped Mn2+:CsPb(BrxCl1-x)3 Nanocrystals as Bright Scintillators for Fast Neutron Imaging, ACS Energy Lett., 2021, 6, 4365–4373 CrossRef CAS PubMed.
- F. Sui, M. Y. Pan, Z. Y. Wang, M. Chen, W. J. Li, Y. Shao, W. M. Li and C. L. Yang, Quantum yield enhancement of Mn-doped CsPbCl3 perovskite nanocrystals as luminescent down-shifting layer for CIGS solar cells, Sol. Energy, 2020, 206, 473–478 CrossRef CAS.
- F. unlu, M. Deo, S. Mathur, T. Kirchartz and A. Kulkarni, Bismuth-based halide perovskite and perovskite-inspired light absorbing materials for photovoltaics, J. Phys. D: Appl. Phys., 2022, 55, 13002 CrossRef.
- X. W. Zhou, Y. Zhao, W. Z. Huang, Y. Y. Wu, Z. E. Wu and G. F. He, Enhanced performance of inverted CsPbBr3 nanocrystal LEDs via Zn (II) doping, Org. Electron., 2021, 96, 106253 CrossRef CAS.
- J. F. Cao, Z. D. Yin, Q. Pang, Y. X. Lu, X. Q. Nong and J. Z. Zhang, Modulating optical properties and interfacial electron transfer of CsPbBr3 perovskite nanocrystals via indium ion and chlorine ion co-doping, J. Chem. Phys., 2021, 155, 234701 CrossRef CAS PubMed.
- Y. J. Guo, J. Su, L. Wang, Z. H. Lin, Y. Hao and J. J. Chang, Improved Doping and Optoelectronic Properties of Zn-Doped CsPbBr3 Perovskite through Mn Codoping Approach, J. Phys. Chem. Lett., 2021, 12, 3393–3400 CrossRef CAS PubMed.
- S. N. Liu, G. Z. Shao, L. Ding, J. M. Liu, W. D. Xiang and X. J. Liang, Sn-doped CsPbBr3 QDs glasses with excellent stability and optical properties for WLED, Chem. Eng. J., 2019, 361, 937–944 CrossRef CAS.
- K. W. Ma, Y. H. Sheng, G. Z. Wang, X. W. Zhang, Y. S. Di, C. H. Liu, L. Y. Yu, L. F. Dong and Z. X. Gan, Stable and multicolor solid-state luminescence of Mn doped CsPb(Cl/Br)3 perovskite quantum dots and its application in light-emitting diodes, J. Lumin., 2022, 243, 118622 CrossRef CAS.
- Y. X. Zhu, B. B. Yang, Q. Lu, Y. Li, M. M. Shi and J. Zou, A Cyan Emitting CsPbBr3 Perovskite Quantum Dot Glass with Bi Doping, ECS J. Solid State Sci. Technol., 2020, 9, 126003 CrossRef CAS.
- H. Kim, S. R. Bae, T. H. Lee, H. Lee, H. Kang, S. Park, H. W. Jang and S. Y. Kim, Enhanced Optical Properties and Stability of CsPbBr3 Nanocrystals Through Nickel Doping, Adv. Funct. Mater., 2021, 31, 2102770 CrossRef CAS.
- X. W. Zhou, Y. Zhao, W. Z. Huang, Y. Y. Wu, Z. E. Wu and G. F. He, Enhanced performance of inverted CsPbBr3 nanocrystal LEDs via Zn (II) doping, Org. Electron., 2021, 96, 106253 CrossRef CAS.
- Y. Ouyang, X. X. Jiang, F. Jiang, L. H. Li, H. P. Zhao, C. Zhang, M. Zheng, W. H. Zheng, Y. Jiang, X. L. Zhu, Y. X. Feng and X. J. Zhuang, Light-Soaking Induced Optical Tuning in Rare Earth-Doped All-Inorganic Perovskite, Adv. Funct. Mater., 2021, 2107086 Search PubMed.
- M. J. Patel, D. Raval, S. K. Gupta and P. N. Gajjar, First-Principles Study of Mn-Doped and Nb-Doped CsPbCl3 Monolayers as an Absorber Layer in Solar Cells, J. Phys. Chem. Lett., 2021, 12, 7319–7327 CrossRef CAS PubMed.
- M. Zeng, F. Locardi, D. Mara, Z. Hens, R. Van Deun and F. Artizzu, Switching on near-infrared light in lanthanide-doped CsPbCl3 perovskite nanocrystals, Nanoscale, 2021, 13, 8118–8125 RSC.
- Y. Zheng, X. Yuan, J. Yang, Q. Li, X. Yang, Y. Fan, H. Li, H. Liu and J. Zhao, Cu doping-enhanced emission efficiency of Mn2+ in cesium lead halide perovskite nanocrystals for efficient white light-emitting diodes, J. Lumin., 2020, 227, 117586 CrossRef CAS.
- D. Q. Chen, S. Zhou, F. F. Tian, H. T. Ke, N. Z. Jiang, S. J. Wang, Y. Z. Peng and Y. Liu, Halogen-Hot-Injection Synthesis of Mn-Doped CsPb(Cl/Br)3 Nanocrystals with Blue/Orange Dual-Color Luminescence and High Photoluminescence Quantum Yield, Adv. Opt. Mater., 2019, 7, 1901082 CrossRef CAS.
- A. Forde, S. A. Thomas, R. J. Petersen, S. L. Brown, D. S. Kilin and E. K. Hobbie, Size-Dependent Doping Synergy and Dual-Color Emission in CsPb1-xMnxCl3 Nanocrystals, J. Phys. Chem. C, 2021, 125, 18849–18856 CrossRef CAS.
- J. Ghosh, M. Hossain and P. K. Giri, Origin and tunability of dual color emission in highly stable Mn doped CsPbCl3 nanocrystals grown by a solid-state process, J. Colloid Interface
Sci., 2020, 564, 357–370 CrossRef CAS PubMed.
- S. K. Mehetor, H. Ghosh, B. Hudait, N. S. Karan, A. Paul, S. Baitalik and N. Pradhan, Reversible Color Switching in Dual-Emitting Mn(II)-Doped CsPbBr3 Perovskite Nanorods: Dilution versus Evaporation, ACS Energy Lett., 2019, 4, 2353–2359 CrossRef CAS.
- W. Z. Wang, J. K. Li, G. B. Duan, H. Zhou, Y. Z. Lu, T. Yan, B. Q. Cao and Z. M. Liu, Study on the Mn-doped CsPbCl3 perovskite nanocrystals with controllable dual-color emission via energy transfer, J. Alloys Compd., 2020, 821, 153568 CrossRef CAS.
- L. Bao, W. Liu, Y. Chen, Y. Zhang and Y. Zhang, Enhanced blue emission from CsPb(Br/Cl)3 perovskite nanocrystals by localized surface plasmon resonance of Au nanoparticles, J. Mater. Chem. C, 2021, 9, 5182–5189 RSC.
- L. M. Dong, Z. Chen, L. Ye, Y. Yu, J. B. Zhang, H. Liu and J. F. Zang, Gram-scale synthesis of all-inorganic perovskite quantum dots with high Mn substitution ratio and enhanced dual-color emission, Nano Res., 2019, 12, 1733–1738 CrossRef CAS.
- M. F. Abdelbar, M. El-Kemary and N. Fukata, Downshifting of highly energetic photons and energy transfer by Mn-doped perovskite CsPbCl3 nanocrystals in hybrid organic/silicon nanostructured solar cells, Nano Energy, 2020, 77, 105163 CrossRef CAS.
- W. He, Q. Zhang, Y. Qi, J. Xiong, P. Ray, N. R. Pradhan, T. V. Shahbazyan, F. Han and Q. Dai, Luminescence properties of CsPbBr3:Mn nanocrystals, J. Nanopart. Res., 2021, 23, 332–337 Search PubMed.
- Z. Tan, J. Pang, G. Niu, J.-H. Yuan, K.-H. Xue, X. Miao, W. Tao, H. Zhu, Z. Li, H. Zhao, X. Du and J. Tang, Tailoring the electron and hole dimensionality to achieve efficient and stable metal halide perovskite scintillators, Nanophotonics, 2021, 10, 2249–2256 CrossRef CAS.
- Y. Lou, Y. Niu, D. Yang, Q. Xu, Y. Hu, Y. Shen, J. Ming, J. Chen, L. Zhang and Y. Zhao, Rod-shaped thiocyanate-induced abnormal band gap broadening in SCN−doped CsPbBr3 perovskite nanocrystals, Nano Res., 2018, 11, 2715–2723 CrossRef CAS.
- D. Parobek, B. J. Roman, Y. Dong, H. Jin, E. Lee, M. Sheldon and D. H. Son, Exciton-to-Dopant Energy Transfer in Mn-Doped Cesium Lead Halide Perovskite Nanocrystals, Nano Lett., 2016, 16, 7376–7380 CrossRef CAS PubMed.
- S. Ye, M. Yu, M. Zhao, J. Song and J. Qu, Low temperature synthesis of high-quality all-inorganic cesium lead halide perovskite nanocrystals in open air and their upconversion luminescence, J. Alloys Compd., 2018, 730, 62–70 CrossRef CAS.
- L. Protesescu, S. Yakunin, M. I. Bodnarchuk, F. Krieg, R. Caputo, C. H. Hendon, R. X. Yang, A. Walsh and M. V. Kovalenko, Nanocrystals of Cesium Lead Halide Perovskites (CsPbX3, X = Cl, Br, and I): Novel Optoelectronic Materials Showing Bright Emission with Wide Color Gamut, Nano Lett., 2015, 15, 3692–3696 CrossRef CAS PubMed.
- G. Pan, X. Bai, W. Xu, X. Chen, Y. Zhai, J. Zhu, H. Shao, N. Ding, L. Xu, B. Dong, Y. Mao and H. Song, Bright Blue Light Emission of Ni2+ Ion-Doped CsPbClxBr3-x Perovskite Quantum Dots Enabling Efficient Light-Emitting Devices, ACS Appl. Mater. Interfaces, 2020, 12, 14195–14202 CrossRef CAS PubMed.
|
This journal is © The Royal Society of Chemistry 2022 |