DOI:
10.1039/D2RA01798A
(Paper)
RSC Adv., 2022,
12, 11923-11932
The effect of Mn and Co dual-doping on the structural, optical, dielectric and magnetic properties of ZnO nanostructures
Received
19th March 2022
, Accepted 8th April 2022
First published on 19th April 2022
Abstract
This paper addresses the effect of Mn (2%, fixed) and Co (2, 4, and 6%, varied) substitution on the structural, optical, dielectric and magnetic responses of ZnO nanoparticles synthesized by the co-precipitation chemical route. The X-ray diffraction analysis confirms the hexagonal wurtzite structure of ZnO. The incorporation of co-doping in the ZnO host, indicated by peak shifting in the XRD patterns, enhanced the crystallite size of the Mn/Co dual-doped ZnO nanoparticles. The FTIR spectra show a characteristic peak around 875 cm−1 assigned to Zn–O stretching, this validates the formation of the wurtzite structure of ZnO. Raman spectroscopy reveals the characteristic band of the wurtzite structure of ZnO nanoparticles along with coupled vibration modes of Mn/Co with the donor defect states in the doped samples. Enhanced optical absorption in the visible region and a significant red-shift in the absorption band edge were found due to doping. The optical band gap is found to decrease from 3.45 eV to 3.15 eV when Co doping increases up to 6%. The dielectric properties, strongly frequency-dependent, decrease with increasing Co doping while the electrical conductivity increases. Ferromagnetism is observed in all the doped samples, and its origin is attributed to an increase in oxygen vacancies which form bound magnetic polarons. It can be inferred that the doping of Mn and Co can be an effective tool to tune the physical properties of ZnO nanoparticles for potential spintronics and high-frequency applications.
1. Introduction
Because of the extraordinary qualities and many applications, zinc oxide (ZnO) has piqued the curiosity of many scientists. It has a broad bandgap (3.37 eV) and a high exciton binding energy (60 meV) under ambient conditions,1 as well as a high piezoelectric constant,2 and chemical and mechanical stability.3 Because of its distinct responses, it has the potential to be used in a wide range of applications, including spintronics, photodetectors, solar cells, light-emitting diodes, gas sensors, and photocatalysts.4,5 Many synthesis techniques, such as co-precipitation, hydrothermal methods, sol–gel methods, wet chemical methods, and chemical vapour deposition, have been used to create ZnO nanostructures.5,6 Among them, the co-precipitation chemical method is a facile, economical, and valuable synthesis route. It was used in the current report to synthesize ZnO and (Mn, Co) doped ZnO nanoparticles.
Doping in ZnO with transition metal (TM) can effectively enhance the physical properties, particularly optical, dielectric and magnetic. Recently, there have been many reports on room temperature ferromagnetism (RTFM) in TM ions (Ni2+ Co2+, Mn2+, etc.) doped ZnO nanostructures.7 The magnetic ordering and magnetization are exceedingly feeble in these ZnO-based diluted magnetic semiconductors (DMSs). Additionally, the mechanism by which TM doped into ZnO exhibits ferromagnetism (FM) is still contested, and whether it is an inherent or extrinsic property remains unclear. Therefore, the root of RTFM has not been entirely resolved and opened a new path in spintronics research. Identifying the key parameters that govern DMS ferromagnetism remains a significant challenge. The system with intrinsic FM and high magnetic moment at room temperature is the only important factor in realizing the dream of a spintronics device.8 Therefore, enhancing their magnetic properties with suitable and tunable FM and coercivity is essential for potential application as a data storage device. Country to single TM doped ZnO, the simultaneous doping of two TM ions in ZnO is preferable to obtain the best magnetic and optical properties due to the occupancy and adjusted position of the Fermi level.9 Consequently, the researchers made immense efforts in the TM dual-doped ZnO and studied distinct combinations of TM ions in the ZnO system to accomplish RTFM.10–12 For instance, it has been reported by Vijayaprasath et al. that the RTFM in ZnO nanoparticles is enhanced by the co-doping Ni/Mn, and its origin is attributed to exchange interaction between localized spin-polarized electrons.13 Intrinsic defects and oxygen vacancies induced the FM at room temperature in Ni–Co co-doped ZnO thin films.14 The vacancy-associated defect may enhance RTFM response in nanocrystalline co-doped Co/Cu ZnO.15 Under the optical point of view, ZnO being wide band gap can only be stimulated in the presence of UV light, which covers only 5% of the sun's energy that reaches the earth. In addition, electron–hole pairs of the photo-excited recombine quickly on the ZnO surface, reducing its photocatalytic activity. To address these issues, doping with transition metals is used to improve ZnO optical characteristics and prevent the recombination of electron–hole pairs of light-generated charge carriers.16 The enhancement in ZnO visible light optical properties can be attributed to the metal ions incorporation in the lattices of ZnO, which slender its band gap and raise the delocalization of electrons in the ZnO nanostructure.17 Though Co and or Mn-doped ZnO system has been numerously studied, most of them focus on thin films or ZnO nanostructures with limited Co/Mn composition,18–20 and the detailed investigation of the physical properties of Mn/Co-doped ZnO nanopowders is still inadequate.
An experimental effort has been made to resolve the contradictory issues on the optical and magnetic properties. In the present work, we have simultaneously doped both Mn and Co ions into ZnO to alter the structures and crystalline properties of the host ZnO lattice to obtain the best optical and magnetic response for future application. The most important findings of the current work are narrowing the band gap of ZnO nanostructure from 3.45 eV to 3.15 by Mn/Co doping. Ferromagnetism is found in all doping concentrations, and it is ascribed to an increase in oxygen vacancies, which create bound magnetic polarons. The dielectric characteristics, which are substantially frequency-dependent, drop as Co substitution increases while electrical conductivity improves. It is concluded that Mn and Co doping can be a useful tool for tuning the properties of ZnO nanoparticles for possible spintronics and photocatalytic applications.
2. Experimental details
2.1 Materials
Sigma-Aldrich provided zinc nitrate hexahydrate [Zn (NO3)2·6H2O], cobalt nitrate hexahydrate [Co(NO3)2·6H2O] and manganese nitrate tetrahydrate [Mn(NO3)2·4H2O], with the purity of 99.99 percent. In addition, sodium hydroxide (NaOH), which was used as a precipitation agent, was also purchased from Sigma-Aldrich. All of the reagents in the experiment were used in the as-received form, with no further purification.
2.2 Sample preparation
The co-precipitation method was used to synthesize undoped and (Mn, Co) dual-doped ZnO nanoparticles. Zinc nitrate hexahydrate was dissolved in 100 ml of deionized water, and then 50 ml of the aqueous solution of NaOH (1 M) was applied drop by drop while constantly swirling until a pH of 10 was reached.21 After the reaction, white precipitates were obtained, washed with distilled water several times in a centrifuge for 10 minutes each to remove contaminants. The obtained precipitates were oven-dried at 80 °C for 10 hours. Lastly, the ZnO nanopowder was heated in a muffle furnace at 600 °C for 2 hours under environmental temperature. A 100 ml solution of zinc nitrate hexahydrate, manganese nitrate tetrahydrate and cobalt nitrate hexahydrate was prepared for Mn and Co dual-doped specimens. A 1 M aqueous solution of NaOH was introduced drop by drop while vigorously swirling until a pH of 10 was obtained. The precipitates were collected and cleaned by centrifugation before being dried in an oven at 80 °C and heated in a furnace at 600 °C for 2 hours. The synthesized samples were labeled as undoped ZnO (pure ZnO), 2% Mn and 2% Co doped ZnO (Mn2Co2), 2% Mn, and 4% Co-doped ZnO (Mn2Co4), and 2% Mn and 6% Co doped ZnO (Mn2Co6).
2.3 Characterization
Next, the manufactured samples were analyzed using XRD (PAN analytical) and Cu K radiation, and the lattice constants were obtained using the Rietveld program's GSAS suite. The morphology of the prepared sample was assessed using Scanning Electron Microscopy (SEM, Hitachi S4800, Japan). The chemical composition was measured using Energy Dispersive X-ray Spectrometer (EDAX) attached to the SEM. The functional groups were identified by Fourier Transfer Infrared Spectroscopy (FTIR) in the frequency region 500 to 4000 cm−1. The optical properties of the samples were investigated by UV-Vis spectrometer. The dielectric characteristics and conductivities of the sample were determined using a gold terminal pellet in the impedance analyzer in the frequency range of 40–106 Hz. The magnetic responses were measured through Superconducting Quantum Interface Devices (SQUID).
3 Results and discussion
3.1 Structural analysis
Fig. 1 demonstrates XRD patterns of the pristine and the dual doped ZnO nanostructures obtained by the co-precipitation route. From these patterns, it can be seen that all samples are polycrystalline. All the specimens have a pure single-phase and crystallize with the hexagonal structure (wurtzite-type P63mc space group). The Lattice parameters of a = 3.2481 Å, c = 5.1852 Å were obtained using Rietveld refinement, (weighted profile factor RWP = 9.51%, and the goodness-of-fit χ2 = 2.572) for the pure ZnO as shown in Fig. 1(a). The diffraction patterns were indexed well according to JCPDS card no. 36-1451, which validates the pure wurtzite phase of ZnO for all synthesized samples, with the diffraction peak corresponding to planes (100), (002), (101), (102), (110), (103) and (200) as shown in Fig. 1(b). Also, there are no extra peaks related to the impurity. It can be seen that there is a relative change in the position of characteristic peak (101) with dopant concentration for all the samples, as shown in Fig. 1(c). This change with dopant concentration confirms substituting dopants ions (Mn, Co) into the ZnO host lattice. The substitution of (Mn, Co) ion into ZnO host material induces lattice distortion, which is due to the difference in the radii of dopant Mn (0.80 Å), Co (0.72 Å), and host Zn (0.74 Å) ions.22,23
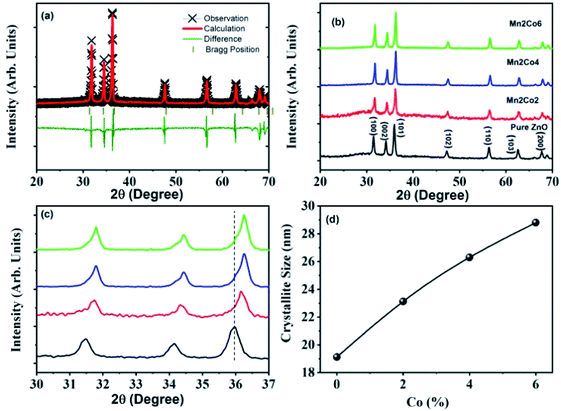 |
| Fig. 1 (a) Shows the solid lines display the Rietveld refinement of the XRD of ZnO nanoparticles, (b) the powder XRD patterns of different doping, (c) the peak shifting with different doping, and (d) the crystallite size of ZnO, and (Co, Mn) dual-doped ZnO. | |
The lattice parameters a and c and the volume of the unit cell (V) of all samples were calculated using the following equations:
|
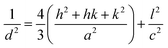 | (1) |
|
 | (2) |
where
d is the interplanar distance and (
h,
k,
l) are the Miller indices. The values of the structural parameters calculated from XRD are listed in
Table 1. The unit cell volume was
V = 47.36 Å
3 for pure ZnO and it increased to
V = 47.41 Å
3 (corresponding to
a = 3.2492 Å,
c = 5.1871) for lower Mn and Co doped ZnO specimen. In higher Mn and Co doped ZnO, the unit cell volume further increased to
V = 47.48 Å
3 (corresponding to
a = 3.2501 Å,
c = 5.1910 Å) and was higher when compared to pure ZnO. This was expected since the ionic radius of Mn
2+ (0.80 Å), and Co
2+ (0.72 Å) are different than Zn
2+ (0.74 Å). Also, it is detected from the XRD patterns that with increasing the concentration of Mn and Co co-dopant in ZnO, the peaks shifted towards higher angle, as shown in
Fig. 1(c). This indicates that samples are transferring to disordered form due to Mn and Co doping.
Table 1 Crystallite size, lattice parameters and unit cell volume for all for pure and Mn/Co-doped ZnO nanostructures assessed from XRD results
Sample |
(hkl) |
2θ (degree) |
FWHM (radians) |
Crystallite size (nm) |
Lattice constants |
Unit cell volume (Å3) |
a (Å) |
c (Å) |
ZnO |
(101) |
35.97 |
0.456 |
19.12 |
3.2481 |
5.1852 |
47.36 |
Mn2Co2 |
(101) |
36.16 |
0.377 |
23.12 |
3.2479 |
5.1864 |
47.38 |
Mn2Co4 |
(101) |
36.24 |
0.331 |
26.3 |
3.2492 |
5.1781 |
47.41 |
Mn2Co6 |
(101) |
36.26 |
0.303 |
28.8 |
3.2501 |
5.1910 |
47.48 |
The following Debye–Scherrer's formula was used to determine the average crystallite size D of the synthesized samples from XRD spectra:
|
 | (3) |
where
λ is the wavelength of incident X-ray,
β (in radians) is the angular peak width at half maximum, and
θ represents the Bragg's diffraction angle.
The crystallite size calculated from XRD data was found to be 19.01 nm (Fig. 1(d)); which increases to 28.82 nm with the increase of Co-doping concentration up to 6% and can be attributed to the intervention of dopant in the ZnO crystal growth.
3.2 SEM analysis
The surface morphology of the synthesized samples, which was studied by SEM, is depicted in Fig. 2(a) and (b). To clarify and understand the crystal growth, only two SEM micrographs of pure and heavily doped ZnO samples (2% Mn and 6% Co) are shown. For the pure ZnO sample, the SEM micrograph shows that particles of the prepared samples are spherical with a large aggregation of small individual nanoparticles and high porosity area as shown in Fig. 2(a). For Mn/Co doped ZnO nanoparticles, it is clearly evident from Fig. 2(b) that aggregation is nearly controlled, and particles show a consistent and uniform morphology with a slightly larger size distribution. The average crystal size of the nanoparticles for pure ZnO was around 70 nm, increasing to around 110 nm as the doping concentration is increased. The particle size rose as the Mn/Co concentration of ZnO increased, which is commensurate with the crystallite size reported from XRD data. The EDX spectrum validates all the selective elements of Zn, Mn, Co, and O (Fig. 2c and d). From the spectra of ZnO nanoparticles, it can be seen that the most abundant element is Zn followed by O. The doping of Mn/Co in ZnO can be reflected from the EDX spectrum. It is clear from the figure that the doped samples contain the expected Mn and Co elements indicating that the prepared samples were free of impurities. The EDX results further confirmed that the compositions of the synthesized samples were nearly in stoichiometric ratio.
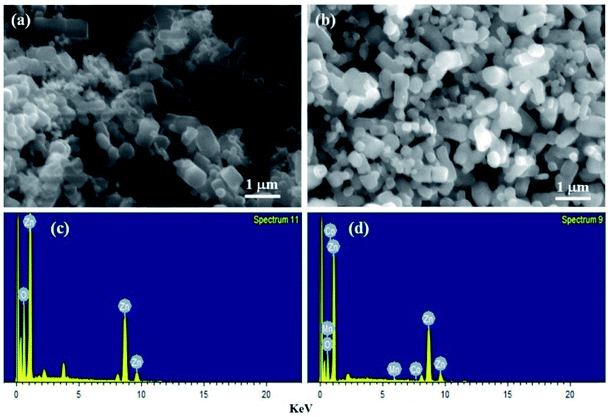 |
| Fig. 2 SEM micrographs of (a) ZnO (b) 2% Mn and 6% Co doped ZnO nanoparticles. EDX spectra of (c) ZnO (d) 2% Mn and 6% Co doped ZnO nanoparticles. | |
3.3 FTIR analysis
Fourier Transfer Infrared Spectroscopy (FTIR) was carried out for the identification of functional groups present in prepared samples in the region 500 to 4000 cm−1. Fig. 3(a) shows the FTIR spectrum for pure ZnO, Mn2Co2, Mn2Co4, and Mn2Co6 samples. Absorption peaks appeared around 625 cm−1, 875 cm−1, 1475 cm−1, a cluster of peaks between 1950 to 2350 cm−1 and 3476 to 3742 cm−1. The peak of absorption that appeared at 875 cm−1 may be due to stretching vibration Zn–O. The peak centred around 1400 cm−1 and 1600 cm−1 can be attributed to the asymmetric and symmetric stretching mode of C
O. A cluster of peaks appeared between 1950 cm−1 and 2350 cm−1 in all samples, corresponding to the presence of CO2 in air trapped on the surface of nanoparticles.24 The peaks observed in the range of 3350–3750 cm−1 corresponds to the O–H stretching vibration of water molecule indicates adsorption of moisture contents on the surface of the sample. A vibrational mode observed around 625 cm−1 for the doped samples is recognized as metal-oxide (Mn–O or Co–O) modes and it confirms the successful inclusion Co/Mn ions into the ZnO lattice sites.25,26 The obtained results from FTIR are in good agreement with XRD findings.
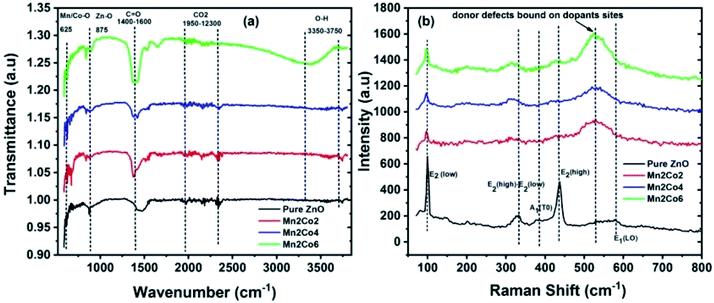 |
| Fig. 3 (a) The FTIR spectra for pure ZnO, Mn2Co2, Mn2Co4, and Mn2Co6 nanoparticles at 300 K. (b) Raman spectra of ZnO and co-doped ZnO specimens. | |
3.4 Raman spectroscopy
Raman spectroscopy has been employed to study the disorder-induced due to the inclusion of dopant in the host matrix and to detect the existence of defects in material. Fig. 3(b) shows a sharp and strong peak centred around 98 cm−1 and 432 cm−1 are due to the E2 (low) and E2 (high) phonon mode of ZnO respectively.27 These peaks become broadened and their intensity decreases or vanishes with doping elements. Peak appeared around 331 and 380 cm−1 is created due to multi phonon mode of E2 (high)–E2 (low) and A1(TO) respectively, which can be attributed to the single-crystalline nature of ZnO.28 The intensities of these peaks decrease with doping, indicating that the inclusion of Mn/Co in the lattice may induce disorder in the system. A weak absorption peak at 580 cm−1 corresponds to E1 (LO) mode of vibration which is created due to oxygen vacancies. For the doped samples, a prominent feature peak appeared around 532 cm−1 which can be ascribed to local vibration mode allied to Mn/Co that is coupled with donor defects like zinc interstitials and doubly ionized oxygen vacancies.29
3.5 Optical analysis
The optical properties of the samples have been investigated by UV-Vis spectrometer. Fig. 4(a) shows the UV-Vis absorption spectra at room temperature of pure ZnO and (Mn, Co) dual doped ZnO (Mn = 2% and Co = 2, 4 and 6%) nanoparticles. The absorbance spectra of (Mn, Co) dual doped ZnO shows a strong enhancement in the absorption of the visible region in comparison with the pure ZnO. This variation in the absorption can be related to size effects such as a shift in the average crystallite size, a disorder that occurs when the Mn and Co concentration increases, and the impurity levels induced by doping.30 The pure ZnO shows an absorption edge at ∼380 nm that shifts towards a higher wavelength (λ) (red-shift) for the doped samples. Along with the bandgap transition, other optical absorption features are observed for the Mn, Co doped ZnO samples in the λ range 510–610 nm, as displayed in the inset of Fig. 4(a). This kind of absorption features has been shown in the optical absorption analysis of Mn and Co doped ZnO in the wavelength range (500–650 nm).31 This kind of absorption behaviour is related to electronic transitions due to the existence of Mn2+ and Co2+ in a tetrahedral.32,33 Given that the wurtzite structure of ZnO (P63/mmc space group) is formed of tetrahedral-coordinated O2− and Zn2+ ions, the identification of this absorption signature in the visible region indicates the efficient replacement of Mn2+ and Co2+ ions for the Zn2+ positions in the ZnO host lattice.
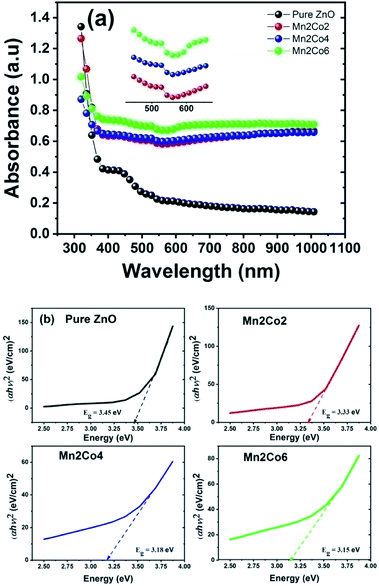 |
| Fig. 4 (a) The UV-Vis absorption spectra for the pure and Mn/Co doped ZnO nanoparticles (b) calculation of energy band gap using Tauc plot method for all samples. | |
The optical band gap (Eg) for the synthesized samples were calculated by Tauc relation given as34
where
α is the coefficient of optical absorption (calculated by relation
α = 2.303 ×
A/
t where
A is the absorbance of the material and
t is the thickness of the cuvette),
B is a constant and
hν is the energy of the incident photon. The value of
n is assumed to be 1/2 since ZnO is a semiconductor with a straight bandgap. The energy gap could be calculated by extrapolating the linear portion of (
αhν)
2 against the
hν plots to the
x-axis. The extrapolated curves of the synthesized pure and (Mn, Co) dual doped ZnO nanoparticles are shown in
Fig. 4(b). The bandgap of pure ZnO is calculated as 3.45 eV, which is larger than the bulk value of ZnO. The calculated value of bandgap progressively decreases from 3.45 eV to 3.15 eV with doping of Mn = 2% and Co = 2, 4 and 6% in ZnO (
Table 2). A decrease in energy the bandgap in various transition metal-doped ZnO was previously obtained by many researchers.
27,35 The enhancement in optical absorption and decrease in band gap of Mn/Co-doped ZnO is attributed to the increase in defects amount in the crystal structure compared to pure ZnO.
17 Mn
2+/Co
2+ incorporation in ZnO adds a tail state in the proximity of the valence band due to defect sites narrowing its energy gap. Thus, visible light's energy photon will be enough to excite electrons from the modified valence band to the conduction state.
36 Moreover, the observed reduction in bandgap with doping may originate from the exchange interaction between s–d and p–d band of localized d electron of Mn
2+/Co
2+ ions and ‘s’ and ‘p’ electrons of the host Zn atom. The negative corrections of the conduction band and the positive corrections valence band may arise from the s–d and p–d exchange interaction; it would also lead to narrowing of the bandgap.
37 The decrease in energy in the bandgap of the doped sample can also be attributed to strong quantum confinements and an increase in surface-to-volume ratio.
38
Table 2 Optical band gap values of ZnO and Mn/Co dual-doped ZnO nanostructures
S. no. |
Sample |
Band gap (eV) |
1 |
ZnO |
3.45 |
2 |
Mn2Co2 |
3.33 |
3 |
Mn2Co4 |
3.18 |
4 |
Mn2Co6 |
3.15 |
3.6 Dielectric properties
The measurement of dielectric constant versus frequency (f) at 300 K for the pure ZnO, Mn2Co2, Mn2Co4 and Mn2Co6 nanoparticles are depicted in Fig. 5(a). The dielectric properties were measured using an impedance analyzer (40 Hz ≤ f ≤ 106 Hz). The samples powder were pressed at 10 tons for 1 min into a circular shaped pellet of diameter 9 mm and thickness ∼3 mm. The pellets were heated at 400 °C for 2 h to remove the porosity in the material. Both sides of the pellets were silver-pasted that acted as electrodes having the sample as the dielectric medium. A thin copper wire joined the electrodes in turn to serve as ohmic contacts. From the measurement of capacitance (C), dielectric loss (ε′′) and the dimensions of the pellet (thickness and area), the dielectric constant (εr) and ac conductivity (σac) were determined using eqn (5) and (6), respectively:39 |
 | (5) |
where C denotes the capacitance, ε0 refer to the permittivity of free space (8.85 × 10−12 m−3 kg−1 s4 A2), d is the thickness of circular-shaped sample, and A represents the pellet area in m2 where ω = 2πf is the angular frequency.
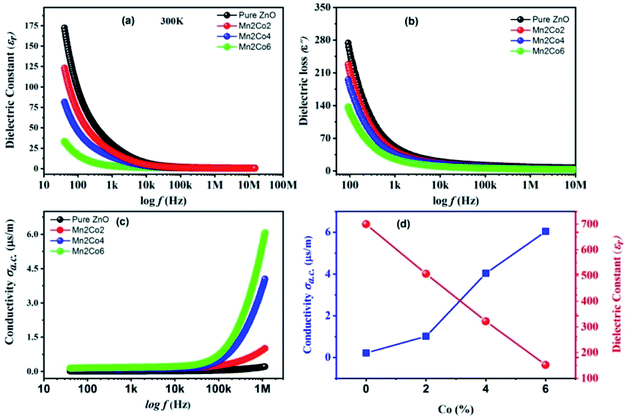 |
| Fig. 5 Room temperature frequency-dependent variation for the pure ZnO, Mn2Co2, Mn2Co4 and Mn2Co6 nanoparticles in (a) εr, (b) ε′′, (c) σac, (d) σac and εr versus Co concentration. | |
The εr versus f for ZnO and co-doped ZnO reveals that the εr decreases with the enhanced f for all the specimens. At low f, an exponentially decreasing trend is observed; however, it becomes slow at high f, attaining a f-independent trend. The reduction in εr with an increase in f is expected behaviour for oxide-based nanoparticles.24 All the f-dependent dielectric constant plots show a rapid decrease at lower fs, this grows steadily slower as f increases until an almost-independent behaviour appears at higher fs. The showed higher values of εr may be discussed by using Rotation dielectric polarization (RDP) and space charge polarization (SCP) mechanisms. The region at the interface restrains the considerable number of oxygen vacancies, vacancy clusters, immobilized radicals, etc. The single or double ionized vacancies are formed when these oxygen vacancies are ionized.40 The randomly oriented combination of negative and positive vacancies constitutes a large dipole moment that can only be polarized in the presence of an external applied field.41 These dipole moments will be field-aligned in the presence of an external field, resulting in RDP. Additionally, the moment of charges in the opposite direction of the electric field induces SCP and are trapped by a defect present at the interface of the nanostructure material. This SCP in the specimens produces large polarization at lower fs and enhanced dielectric constant due to electrically active grain boundaries.42 At higher f, the εr decreases with increasing f because any component granting to polarizability is observed to lag behind the applied field. Beyond a particular f, dipoles and the alternating field are strongly mismatched finally; the dielectric constant receive a constant value; hence a f-independent dielectric response becomes visible.43
3.7 Dielectric loss
Fig. 5(b) illustrates the dielectric loss measurement ε′′ (imaginary part of εr) versus f at 300 K for the pure and Mn 2%, Co 2, 4 and 6% doped ZnO nanoparticles. The figure shows that the ε′′ decreases sharply with increasing f up to 1 kHz followed by a steady slow decrease. All the samples show similar activity, i.e., low-f dispersion behaviour and f-independent nature of dielectric loss at high f. The migration of ions in the material is ascribed to the decrease in ε′′ in the low fs region.41 Likewise, the behaviour of ε′′ at low and intermediate fs can be attributed to ionic hopping, i.e. losses in conduction due to ion migration.6 On the other hand, ion vibrations might be the only way for ε′′ steady behaviour at high fs.40
3.8 Electrical conductivity
Fig. 5(c) shows the f-dependent σac for the pure and Mn, Co doped ZnO nanoparticles measured at room temperature. The σac enhances with rising fs, especially at high fs, which corresponds to the published results.44 The enhancement in σac is negligible at low fs, showing a gradual increase with the increase in fs, finally showing a rapid increase in the conductivity in the higher fs region. The variation of εr and σac as a function of Co doping is shown in Fig. 5(d). The hopping model can be used to explain the behaviour of conductivity.24 The conductivity is steady in the low fs region; the transport occurs on infinite paths. However, in the high fs region, the transport takes place via the hopping of charge carriers. The hopping of charges between the charge carriers ions is enhanced by increasing f of the applied field, which improves the mobility of carriers, thus leading to an increase in conductivity.45 The undoped and (Mn,Co) doped ZnO specimens follow the following equation, σac = ε0ε′′ω, where ω = 2πf. It is obvious from the equation that σac is ε′′ dependent only. Hence, as the ε′′ reduces with increase in f, it σac enhances. This result is well-consistent with literature, where it is revealed that the increase σac with the enhancement in f is ascribed to the series resistance effect.46 Actually, the increase in σac can be described by two possible mechanisms; one is the electric energy allied with the high f can effectively promote the hopping of charge carriers between the nano-sized particles, and the second is owing to the improved dielectric relaxation of the polarization of ZnO nanostructure in the high-f range.47
3.9 Magnetic properties
For practical spintronic application, the room temperature magnetic properties of DMS are important. Fig. 6(a) illustrates the magnetization hysteresis loops (M–H loops) for Mn2Co2, Mn2Co4 and Mn2Co6 nanoparticles measured at 300 K. The pure ZnO sample display a diamagnetic response at 300 K as expected (the data is not shown here). It is evident from the figure that the FM behaviour was progressively enhanced for Mn = 2% and Co = 2, 4% doped ZnO. However, the FM behaviour decremented for Mn = 2% and Co = 6% doped sample. The remanent magnetization (Mr) and coercive field (Hc) is also observed to enhance for Mn2Co2 and Mn2Co4; however, they decreased for Mn2Co6. A Hc of 27 and 56 Oe was found for Mn2Co2, and Mn2Co4 samples, respectively and Mr of 0.0014 and 0.0.027 emu/g was observed for Mn2Co2 and Mn2Co4, respectively (Table 3). The Hc and Mr subsequently decreased to 51 Oe and 0.0022 emu g−1 correspondingly for heavily doped samples (Mn2Co6). When ZnO is heavily doped (Mn = 2 and Co = 6%), the ZnO lattice's rapid expansion and the FM ordering may be destroyed due to significant structural disorder.40 The observed highest Mr for 4% Co (Mn2Co4) is higher than that reported in the literature.48
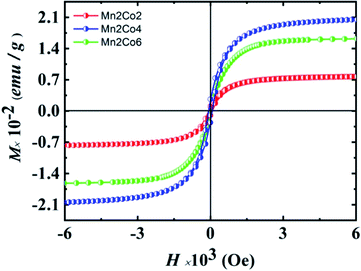 |
| Fig. 6 M–H curves for Mn 2% (fixed) and Co 2, 4, and 6% doped ZnO at room temperature. | |
Table 3 The magnetic properties parameters for all the doped samples
Sample |
Coercive field (Oe) |
Remanent magnetization (emu g−1) |
Saturation magnetization (emu g−1) |
Mn2Co2 |
27 |
0.0014 |
0.038 |
Mn2Co4 |
56 |
0.0027 |
0.101 |
Mn2Co6 |
51 |
0.0022 |
0.080 |
The origin of RTFM in DMS is an open-ended question that may originate from many potential sources. The most likely contamination of CoO and MnO may come at the time of preparation. The CoO phase is antiferromagnetic with a Néel temperature of 293 K and hence cannot be the basis of RTFM.49 MnO and its secondary phases MnO2 and Mn2O3 are antiferromagnetic at room temperature.50 Our XRD and Raman results show no phase related to MnO and CoO, signifying that ferromagnetism of (Mn, Co) dual doped ZnO is likewise inappropriate to the Mn and Co-associated phases. In oxides with a wide bandgap, the TM ions and the impurity bands hybridization are robustly linked to the TM 3d bands inside the bandgap affecting the magnetic behaviors.51 The impurity band (oxygen vacancy, VO) may fall into the split 3d major spin and minor spin states of (Mn, Co) dual doped ZnO. In the case of increasing VO content, the spin-up and spin-down state is unequal, causing unpaired electrons in the degenerated d-orbitals (eg) of TM ions that cause an imbalance of electron distribution.51,52 To keep the system charge-neutral, few oxygen ions leave from the lattice, leaving oxygen in the vicinity of Mn and Co doped ions. The electronic configuration for Mn2+ ion is 3d54s0; the three electrons of 3d level occupy the t2g state while the other two electrons reside in the eg state. 3d74s2 is the electronic configuration of Co2+ where its eg level is occupied with two electrons.52 Theoretically, it is reported by Jorgensen et al. that oxygen vacancies can cause significant changes in the band structures of the parent oxides and might make contributions considerably to ferromagnetism.53 Oxygen vacancies are best known as color centers (F centers) illustrate three different charge states, F2+ as unoccupied corresponding to V++0, F+ as singly occupied corresponding to V+0 and F0 as doubly occupied corresponding to
. V++0 and
do not contribute to the ferromagnetism in ZnO because it is in zero spin ground states.51 The V+0 carries a strongly localized trapped electron that induced bound magnetic polarons (BMP) and magnetic moment in DMSs.54 The origin of ferromagnetism in our observed experiment results can be attributed to V+0 and Mn2+/Co2+ ions; VO electrons lying at the bottom of the gap interact with the many surrounding ions Mn2+ and Co2+, directing all spins, forming a BMP, thereby inducing ferromagnetic ordering. The BMPs overlap when the concentration of TM ions goes over a critical threshold leading to long-range ferromagnetism ordering.52 Our investigation shows that in the Mn2Co4 sample, the simultaneous doping of Mn and Co into ZnO can increase the carrier concentration through oxygen vacancies, leading to enhanced RTFM.
4. Conclusions
Pure ZnO and Mn, Co doped ZnO nanoparticles with a fixed Mn content of 2% and varies Co substitution (2%, 4%, and 6%) were prepared by co-precipitation synthesis route. XRD characterization revealed that the nanoparticles crystallize in a hexagonal wurtzite structure with no extra phase present. The crystallite size was established to be (Mn, Co) doping depended and it increased in the doped samples compared to the pure ZnO. SEM analysis showed that the nanoparticles were spherical, and the required elements were present as confirmed by EDX analysis. The FTIR and Raman spectroscopy showed the wurtzite structure of ZnO nanoparticles and the vibration mode assigned to the bound of Mn/Co with the donor defects for the doped samples. The UV-Vis spectroscopy showed a red-shift for Mn/Co-doped ZnO. Furthermore, it revealed that dual doping in ZnO can be used to manipulate both optical absorption and bandgap of ZnO nanoparticles. Our study showed that dielectric constant and dielectric loss decremented with increasing doping and frequency whilst the electrical conductivity enhanced. Based on M–H measurements, we showed that room-temperature ferromagnetism could be induced in the (Mn, Co) doped samples, and its origin is attributed to bound magnetic polarons. Thus, our systematic study may contribute to advancing research on magneto-optical and spintronic materials based on Mn/Co dual doped ZnO nanostructures.
Funding
This work was financially supported by Taif University with a Project number (TURSP-2020/228), Taif University, Taif, Saudi Arabia.
Author contributions
Conceptualization, A. S., K. S., M. S. and R. K; data curation, A. S., M. S., Y. I. and N. A.; formal analysis, A. R. K., G. A., W. H. S., and K. A.; investigation, A. S., K. S., M. S., N. A. and R. K.; methodology, A. S., W. H. S., G. A. and K. A.; project administration, A. S., A. R. K., Y. I. and R. K.; resources, A. S., G. A., A. R. K., K. A.; software, A. S., N. A. and R. K.; supervision, A. S., Y. I., W. H. S., S. A. O. and R. K.; writing—original draft, A. S., A. R. K. and R. K.; writing – review and editing, A. S., K. S., W. H. S., G. A., K. A., S. A. O. and R. K.; all authors have read and agreed to the published version of the manuscript.
Conflicts of interest
There are no conflicts to declare.
Acknowledgements
The authors appreciated Taif University Researchers Supporting Project number TURSP-2020/228, Taif University, Taif, Saudi Arabia.
References
- M. Y. Ali, M. Khan, A. T. Karim, M. M. Rahman and M. Kamruzzaman, Heliyon, 2020, 6, e03588 CrossRef PubMed.
- R. Sangeetha, S. Muthukumaran and M. Ashokkumar, Spectrochim. Acta, Part A, 2015, 144, 1–7 CrossRef CAS PubMed.
- K. Karthika and K. Ravichandran, J. Mater. Sci. Technol., 2015, 31, 1111–1117 CrossRef CAS.
- K. C. Verma, in Magnetic Materials and Magnetic Levitation, IntechOpen, 2020 Search PubMed.
- A. B. Djurišić, X. Chen, Y. H. Leung and A. M. C. Ng, J. Mater. Chem., 2012, 22, 6526–6535 RSC.
- R. Khan, C. I. L. de Araujo, T. Khan, S. A. Khattak, E. Ahmed, A. Khan, B. Ullah, G. Khan, K. Safeen and A. Safeen, J. Mater. Sci.: Mater. Electron., 2019, 30, 3396–3404 CrossRef CAS.
- K. C. Verma, in Magnetic Materials and Magnetic Levitation, IntechOpen, 2020, p. 109 Search PubMed.
- S. Sinha, M. Singh and R. Singh, Int. J. Emerg. Res. Manag. Technol, 2015, 4, 16–20 Search PubMed.
- N. Lathiotakis, A. N. Andriotis and M. Menon, Phys. Rev. B: Condens. Matter Mater. Phys., 2008, 78, 193311 CrossRef.
- D. Sharma and R. Jha, Ceram. Int., 2017, 43, 8488–8496 CrossRef CAS.
- H. Cao, P. Lu, N. Cai, X. Zhang, Z. Yu, T. Gao and S. Wang, J. Magn. Magn. Mater., 2014, 352, 66–71 CrossRef CAS.
- J. Beltrán, C. Barrero and A. Punnoose, J. Phys. Chem. C, 2016, 120, 8969–8978 CrossRef.
- G. Vijayaprasath, R. Murugan, S. Asaithambi, P. Sakthivel, T. Mahalingam, Y. Hayakawa and G. Ravi, Ceram. Int., 2016, 42, 2836–2845 CrossRef CAS.
- A. Kocyigit and R. Topkaya, Mater. Res. Express, 2019, 6, 096116 CrossRef CAS.
- S. Das, A. Bandyopadhyay, P. Saha, S. Das and S. Sutradhar, J. Alloys Compd., 2018, 749, 1–9 CrossRef CAS.
- C. B. Ong, L. Y. Ng and A. W. Mohammad, Renewable Sustainable Energy Rev., 2018, 81, 536–551 CrossRef CAS.
- R. Ullah and J. Dutta, J. Hazard. Mater., 2008, 156, 194–200 CrossRef CAS PubMed.
- B. Yahmadi, O. Kamoun, B. Alhalaili, S. Alleg, R. Vidu and N. Kamoun Turki, Nanomaterials, 2020, 10, 1507 CrossRef CAS PubMed.
- W. Li, G. Wang, C. Chen, J. Liao and Z. Li, Nanomaterials, 2017, 7, 20 CrossRef PubMed.
- R. K. Yadav and P. Chauhan, Indian J. Pure Appl. Phys., 2020, 57, 881–890 Search PubMed.
- R. Khan and S. Fashu, J. Mater. Sci.: Mater. Electron., 2017, 28, 10122–10130 CrossRef CAS.
- R. T. Da Silva, A. Mesquita, A. O. De Zevallos, T. Chiaramonte, X. Gratens, V. A. Chitta, J. M. Morbec, G. Rahman, V. M. Garcia-Suarez and A. C. Doriguetto, Phys. Chem. Chem. Phys., 2018, 20, 20257–20269 RSC.
- P. Gu, X. Zhu and D. Yang, RSC Adv., 2019, 9, 8039–8047 RSC.
- M. Zubair, A. Khan, T. Hua, N. Ilyas, S. Fashu, A. M. Afzal, M. A. Safeen and R. Khan, J. Mater. Sci.: Mater. Electron., 2021, 32, 9463–9474 CrossRef.
- D. Kumbhar, S. Kumbhar, A. Dhodamani, S. Delekar, N. Harale, R. Nalawade and A. Nalawade, Inorg. Nano-Met. Chem., 2021, 51, 1258–1271 CAS.
- G. Vijayakumar, G. Boopathi and M. Elango, Mater. Technol., 2019, 34, 807–817 CrossRef CAS.
- C. Belkhaoui, N. Mzabi, H. Smaoui and P. Daniel, Results Phys., 2019, 12, 1686–1696 CrossRef.
- M. Almoussawi, A. Abdallah, K. Habanjar and R. Awad, Mater. Res. Express, 2020, 7, 105011 CrossRef CAS.
- A. Chanda, S. Gupta, M. Vasundhara, S. R. Joshi, G. R. Mutta and J. Singh, RSC Adv., 2017, 7, 50527–50536 RSC.
- W.-J. Qin, J. Sun, J. Yang and X.-W. Du, Mater. Chem. Phys., 2011, 130, 425–430 CrossRef CAS.
- K. J. Kim and Y. R. Park, J. Appl. Phys., 2003, 94, 867–869 CrossRef CAS.
- S. Deka and P. Joy, Solid State Commun., 2007, 142, 190–194 CrossRef CAS.
- M. Patra, K. Manzoor, M. Manoth, S. Vadera and N. Kumar, J. Phys. Chem. Solids, 2009, 70, 659–664 CrossRef CAS.
- K. Safeen, V. Micheli, R. Bartali, G. Gottardi, A. Safeen, H. Ullah and N. Laidani, Mod. Phys. Lett. B, 2019, 33, 1950313 CrossRef CAS.
- M. Ahmad, E. Ahmed, Y. Zhang, N. Khalid, J. Xu, M. Ullah and Z. Hong, Curr. Appl. Phys., 2013, 13, 697–704 CrossRef.
- E. Pragna, M. Ramanadha, A. Sudharani and K. S. Kumar, J. Supercond. Novel Magn., 2021, 1–10 Search PubMed.
- S. D. Birajdar, P. P. Khirade, V. Bhagwat, A. V. Humbe and K. Jadhav, J. Alloys Compd., 2016, 683, 513–526 CrossRef CAS.
- C. Mrabet, O. Kamoun, A. Boukhachem, M. Amlouk and T. Manoubi, J. Alloys Compd., 2015, 648, 826–837 CrossRef CAS.
- G. Asghar, S. Asri, S. N. Khusro, G. H. Tariq, M. Awan, M. Irshad, A. Safeen, Y. Iqbal, W. H. Shah and M. Anis-ur-Rehman, J. Electron. Mater., 2020, 49, 4318–4323 CrossRef CAS.
- K. Rajwali and F. Ming-Hu, Chin. Phys. B, 2015, 24, 127803 CrossRef.
- N. Grimes and R. W. Grimes, J. Phys.: Condens. Matter, 1998, 10, 3029 CrossRef CAS.
- R. Khan, S. Fashu and M.-U. Rahman, J. Mater. Sci.: Mater. Electron., 2016, 27, 7725–7730 CrossRef CAS.
- R. Khan, S. Fashu and Y. Zaman, J. Mater. Sci.: Mater. Electron., 2016, 27, 5960–5966 CrossRef CAS.
- J. V. Siva, S. K. Tripathy and R. A. Ramalingeswara, J. Electron. Mater., 2020, 49, 3540–3554 CrossRef.
- N. Mazumder, A. Bharati, S. Saha, D. Sen and K. Chattopadhyay, Curr. Appl. Phys., 2012, 12, 975–982 CrossRef.
- O. Pakma, N. Serin, T. Serin and Ş. Altındal, J. Phys. D: Appl. Phys., 2008, 41, 215103 CrossRef.
- C.-H. Ho, C.-D. Liu, C.-H. Hsieh, K.-H. Hsieh and S.-N. Lee, Synth. Met., 2008, 158, 630–637 CrossRef CAS.
- M. Shatnawi, A. Alsmadi, I. Bsoul, B. Salameh, G. Alna'washi, F. Al-Dweri and F. El Akkad, J. Alloys Compd., 2016, 655, 244–252 CrossRef CAS.
- A. Mandziak, G. D Soria, J. E. Prieto, P. Prieto, C. Granados-Miralles, A. Quesada, M. Foerster, L. Aballe and J. de la Figuera, Sci. Rep., 2019, 9, 1–8 CAS.
- T.-L. Phan and S. Yu, J. Phys. Chem. C, 2013, 117, 6443–6453 CrossRef CAS.
- J. Coey, M. Venkatesan and C. Fitzgerald, Nat. Mater., 2005, 4, 173–179 CrossRef CAS PubMed.
- Q. Gao, Y. Dai, B. Han, W. Zhu, X. Li and C. Li, Appl. Surf. Sci., 2019, 490, 178–187 CrossRef CAS.
- J. Jorgensen, B. Veal, W. Kwok, G. Crabtree, A. Umezawa, L. Nowicki and A. Paulikas, Phys. Rev. B: Condens. Matter Mater. Phys., 1987, 36, 5731 CrossRef CAS PubMed.
- H. Ji, C. Cai, S. Zhou and W. Liu, J. Mater. Sci.: Mater. Electron., 2018, 29, 12917–12926 CrossRef CAS.
|
This journal is © The Royal Society of Chemistry 2022 |