DOI:
10.1039/D2RA01591A
(Paper)
RSC Adv., 2022,
12, 13401-13405
Magnetic coupling induced by the interaction between endohedral metal borofullerenes†
Received
11th March 2022
, Accepted 15th April 2022
First published on 4th May 2022
Abstract
Superatom-assembled materials have highly tunable magnetic and electronic properties and parameters of clusters. Here, eight superatom dimers composed of two U@B40 motifs have been studied by the density functional theory. Calculation results show that U@B40 dimers exhibit spin antiferromagnetic coupling, spin ferromagnetic coupling and nonmagnetic, that is, the magnetic coupling is induced by the interaction between the U@B40 superatoms. In addition, the monomers in U@B40 dimers still retain the superatomic orbitals, and some of the super atomic orbitals disappear due to the interaction between monomers. The assembly based on U@B40 induced a decrease in the energy gap. This study provides a basis for a deep understanding of controlling the cluster-assembled materials for tailoring their functionalities.
Introduction
Cluster-assembled materials (CAMs) have attracted wide interest in recent years1–4 and have been applied in organic pollutant sensing and biomedical applications,5 gas sensors,6,7 hydrogen storage,8 etc. Thus, numerous CAMs have been studied, such as CdO and ZnO clusters,9,10 TM@Si12 (TM = 3d transition metal),11–13 M12N12 (M = Al, Ga) fullerene-like clusters14 and noble metal nanostructures.5,15–17 CAM building blocks are clusters, meaning, more atoms and arrangements are available to select and assemble to adjust the properties of the materials.1 Stable cluster building blocks for self-assembly are of crucial importance, as many clusters at these small sizes have lifetimes too short for controlled assembly into materials.1 In addition, clusters with closed electronic shells and large HOMO–LUMO (highest occupied molecular orbital-lowest unoccupied molecular orbital) gaps have enhanced stability and reduced reactivity.18 Thus, superatoms, such as Al13−,19–21 As7,22,23 B40,24 C60,25–27 An@C28,28 can be used as building blocks in creating self-assembled cluster materials.
Previous studies on endohedral-metallofullerene (EMF) containing actinides have promoted the application of actinide compounds in functional nanomaterials and nanomedicine.29–31 In 2014, a combination of experimental and theoretical research discovered the B40 hollow cage.32 Subsequently, studies have shown that B40 clusters exhibit superatomic properties, but their electronic configuration is not fully filled.33 Moreover, the clusters of embedded atoms or small molecules can form stable core@shell systems.34,35 The studies on U@B40 prove that it is indeed a stable closed-shell electronic structure.35 Therefore, the U@B40 as building blocks for assembly are capable of tailoring more stable nanostructures or nanocrystals. Moreover, the interaction between EMFs of U@C28 can lead to different chemical and physical adsorption structures, which are derived from different magnetic coupling.36 Whether the interaction between U@B40 superatoms also causes magnetic coupling, will be helpful for the study of functional CAMs.
Currently, cluster-assembled materials are explored and tailored to control their functions.1 In this work, we constructed eight U@B40 dimers based on the first principles. Unlike the U@B40 monomer,35 the assembly based on U@B40 affects the magnetic properties of the structures, and different conformations exhibit different magnetic coupling. The monomers in the U@B40 dimer also retain part of the superatomic orbitals, similar to the assembly of B40 oligomers.24 The energy gap of the U@B40 dimer is smaller than that of U@B40.35 Therefore, we propose a possible route to construct cluster-assembled materials using U@B40 superatoms.
Models and computational methods
The U@B40 has two hexagons (61 and 62) and four heptagons (71–74), as the lower right corner of Fig. 1. In this work, we enumerated various possible directions along the two U@B40 and obtained eight U@B40 dimers, and arranged them according to their total energies. Structure (a) is formed by the 73 heptagons of one U@B40 stacked onto the vertical 71 heptagons of another (denoted as 73⊥71); it has the lowest total energy. Structures (b) and (c) are formed by 73 heptagons of one cluster parallel and antiparallel to 71 heptagons of another (denoted as 73↗↗ 71 and 73↗↙71, respectively). Structures (d), (e), (g) and (h) are formed by the 73 heptagons or 61 hexagons of one cluster stacked onto the 61 or 62 hexagons of another. The structure (f) is formed by the B atoms of one cluster connecting to the B atoms of another cluster (represented as vertex–vertex). The relative energies between other structures and the lowest energy structure (73⊥71) are placed in brackets in Fig. 1. The relative energies of the U@B40 dimers are listed in Table S1† in the ESI. Since the energy of the conformation 73⊥71 is the lowest, the following analysis and discussion are mainly performed on it.
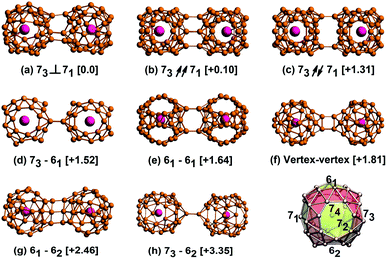 |
| Fig. 1 Structure diagrams for all the isomers formed by the interaction between two U@B40 superatoms. (a) Stacking the 73 heptagons of one cluster vertical to the 71 heptagons of another. (b and c) Stacking the 73 heptagons of one cluster parallel or antiparallel to the 71 heptagons of another. (d and e) Stacking the 73 heptagons or 61 hexagons of one cluster onto the 61 hexagons of another. (f) Connecting the B atoms of one cluster with the B atoms of another cluster. (g and h) Stacking the 61 hexagons or 73 heptagons of one cluster onto the 62 hexagons of another. The values in brackets are the relative energies between each isomer and the lowest energy structure, given in eV. The structure of the U@B40 is shown in the lower right corner. A similar drawing method refers to ref. 24 and 37. | |
The Amsterdam density functional package (ADF, 2012.01)38 was employed to perform calculations. To ensure the reliability of the obtained structures, geometry optimizations were performed without imposing any symmetry constraints. All calculations presented in this work were performed using Perdew–Burke–Ernzerhof (PBE)39 generalized gradient approximation exchange–correlation functionals. Scalar relativistic effects were accounted for using the zero-order regular approximation (ZORA) method. TZ2P Slater basis sets (relativistic valence triple-ζ) with two polarization functions were employed,40 and the frozen-core approximation was used for 1s–4f electrons of the uranium atom. The empirical dispersion-corrected density functional theory (DFT-D3)41 was used to fully obtain the intermolecular interaction of the U@B40 structures.
Results and discussion
The spin polarization of U@B40 dimers was analyzed and the results were different from those of U@B40 without spin polarization.35 As shown in Fig. 2, the spin orientation of one U@B40 monomer in the 73⊥71, 73↗↗71 and 61–62 conformations is spin-up, and that of another monomer is spin-down. The spin orientations of the two monomers are opposite, thus exhibiting the spin antiferromagnetic coupling phenomenon, which is consistent with (U@C28)2.42 For 73↗↙71, vertex–vertex and 73–62 conformations, the spin orientations of the two U@B40 monomers are spin-up, and thus exhibiting spin ferromagnetic coupling. Distinct from 73–62, although the ground state of 73–61 conformation is also a triplet state, the two unpaired electrons are occupied on one monomer. Thus, one U@B40 monomer exhibits spin polarization, while another monomer has no spin polarization (shown in Fig. 2(d)). In addition, the ground state of the 61–61 structure is a closed-shell singlet state without spin polarization, similar to U@B40.35 The results show that different U@B40 dimers exhibit different spin polarization phenomena, thus U@B40 as the motif for assembly can affect the magnetic properties.
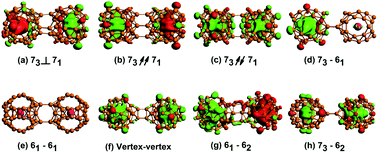 |
| Fig. 2 Spin density diagram of U@B40 dimers for eight conformations. The green region represents spin up and the red region represents spin down. The isosurface value is 0.01 a.u. | |
To further explore the origin of the spin-polarized phenomenon, the MOs of U@B40 dimers were analyzed. There are 232 α and 232 β MOs of the conformation 73⊥71, and the frontier MOs diagram is shown in Fig. 3. The HOMO for the α electron (denoted as HOMOα) is strongly localized on one U@B40 monomer, while the HOMO for β electron (HOMOβ) mainly occupies another monomer. The other occupied MOs were delocalized on the U@B40 dimer. Therefore, the spin density of 73⊥71 conformations is mainly contributed by HOMOα and HOMOβ. The spin density for spin-up comes from HOMOα, while that for spin-down derives from HOMOβ, thus exhibiting spin antiferromagnetic coupling. Furthermore, the MOs of other U@B40 dimers were also analyzed, and the results show that the spin density comes from the frontier MOs of structures (as shown in Fig. S1–S7†).
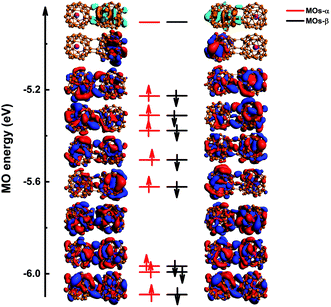 |
| Fig. 3 The frontier molecular orbital (MOs) diagram of the ground state of 73⊥71 conformations. The red and black lines represent α-MOs and β-MOs, respectively. The MOs indicated by blue and red are occupied MOs, while those indicated by orange and cyan are unoccupied MOs. The isosurface value is 0.01 a.u. | |
Previous studies on B40 oligomers have shown that B40 monomers still retain superatomic properties.24 Similarly, the superatomic MOs of 73⊥71 conformations were also analyzed in detail. In the MOs with lower energy levels, we found that the monomeric superatomic orbitals are composed of B40 cages. While superatomic orbitals are contributed by the 2s orbital of B atoms, similar to the superatomic orbital 1S, 1P, 1D and 1F of B40 (ref. 33) (as shown in Fig. 4). The superatomic MOs of two monomers cooperate in in-phase and out-of-phase cooperation, denoted as 1S–1S, 1P–1P, 1D–1D, 1F–1F. In the MOs with higher energy levels, the U@B40 monomer still retains superatomic MOs, and the superatomic orbitals are a hybridization of the 7s, 7p, 6d, and 5f orbitals of the U atom with the B40 cage orbitals.35 The superatomic MOs of two U@B40 monomers cooperate in in-phase and out-of-phase, denoted as 2S–2S, 2P–2P, 2D–2D, 2F–2F, as shown in Fig. 5. However, U@B40 exhibits a 32-electron closed-shell singlet configuration.35 There should be two S–S, six P–P, ten D–D and fourteen F–F superatomic MOs in accordance with the in-phase and out-of-phase cooperation between each other. Here, we found that some monomeric superatomic orbitals disappeared due to the stronger orbital interaction. The disappearance of 1D–1D MOs is caused by the distortion of the B40 cages and the disappearance of 2D–2D and 2F–2F MOs result from the deviation of the U atom from the cage center. Fig. 4 and 5 are the superatomic MOs diagrams of α electrons, and the superatomic MOs diagrams of β electrons are shown in Fig. S8 and S9† in ESI. In addition, we also analyzed the MOs of 61–61 conformation, as this conformation is less twisted and the U atom is not severely deviated from the B40 cage center. The results show that only two 2F–2F MOs disappeared, as shown in Fig. S10† in the ESI.
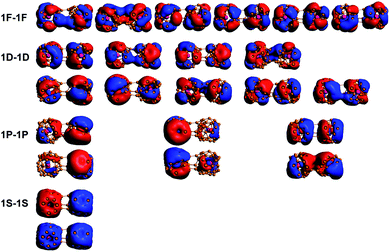 |
| Fig. 4 The MOs diagram for α electrons of 73⊥71 confirmations for the U@B40 superatomic orbital components. Superatomic orbitals 1S, 1P, 1D, and 1F are composed of the B40 cages. The blue and red areas indicate the positive and negative signs of the wave functions, respectively. Isovalue = 0.02 a.u. | |
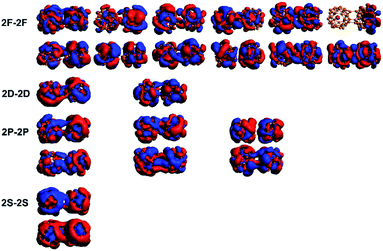 |
| Fig. 5 MOs-α of the 73⊥71 conformations for the U@B40 superatomic orbital components. 2S, 2P, 2D, and 2F refer to the U@B40 monomer superatomic orbitals. | |
To further explore the orbital composition, the density of states of 73⊥71 conformations was analyzed. The total density of states (TDOS) of the U@B40 dimer for 73⊥71 conformations, the local density of states (LDOS) and the partial density of states (PDOS) of U atoms are displayed in Fig. 6. The results demonstrate that U atoms in 73⊥71 conformations mainly contribute to frontier and unoccupied MOs, and the occupied MOs are mainly contributed by the 5f orbitals of the U atoms. Therefore, 1S–1S, 1P–1P, 1D–1D and 1F–1F MOs are composed of B40 cages, and 2S–2S, 2P–2P, 2D–2D, 2F–2F MOs are composed of B40 cages and U atoms. Further, the DOS of other U@B40 dimers, 73↗↙71, 73–61, 61–61 and 61–62, were also analyzed (as shown in Fig. S11–S14†). Furthermore, the HOMO–LUMO gap of the U@B40 dimers was analyzed, as listed in Table 1. The results showed that the HOMO–LUMO gap of the eight conformations of U@B40 dimers are 0.32 eV, 0.32 eV, 0.27 eV, 0.16, 0.45 eV, 0.30 eV, 0.30 eV and 0.12 eV, respectively. While the HOMO–LUMO gap of U@B40 was 0.76 eV.35 Therefore, U@B40 assembly causes a reduction in the energy gap, which is similar to the result of previous studies on B40 superatom-assembly.24
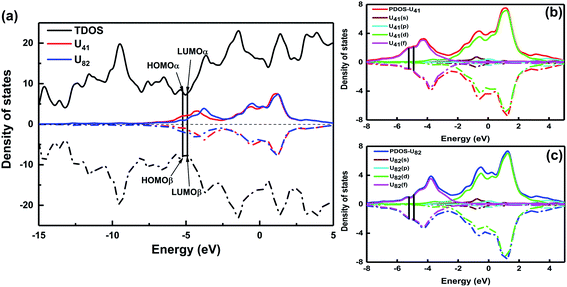 |
| Fig. 6 Density of states of structure 73⊥71. (a) The total density of states (TDOS) of 73⊥71 and the local density of states (LDOS) of U atoms. (b) and (c) The partial density of states (PDOS) of s, p, d, and f atomic orbital of two U atoms. | |
Table 1 The HOMO–LUMO gap of U@B40 dimers
The HOMO–LUMO gap (eV) |
73⊥71 |
73↗↗71 |
73↗↙71 |
73–61 |
61–61 |
Vertex–vertex |
61–62 |
73–62 |
0.32 |
0.32 |
0.27 |
0.16 |
0.45 |
0.30 |
0.30 |
0.12 |
Conclusions
In this study, U@B40 superatom dimers were investigated using the first principles. The results show that different U@B40 dimers exhibit different spin polarization phenomena, they have spin antiferromagnetic coupling, spin ferromagnetic coupling and nonmagnetic. As such, the magnetic coupling can be tuned according to different types of the U@B40 assembly. Moreover, the spin density mainly comes from the frontier MOs. Further analysis of the MOs revealed that the monomer of the U@B40 dimers still retained some superatomic properties. One kind of superatomic orbital is composed of B40 cages and another kind of superatomic orbital is composed of B40 and U atoms. In addition, the U@B40 superatomic assembly causes the energy gap to decrease. This work mainly studied the interaction between embedded metal fullerenes, which provides a new idea for cluster assembly materials.
Author contributions
Jia Wang calculated and analyzed the results. All the authors contributed to the general discussion.
Conflicts of interest
There are no conflicts to declare.
Acknowledgements
This work was financially supported by the National Natural Science Foundation of China (grant number 11947039), the Natural Science Foundation Project of Jilin Province (grant number YDZJ202101ZYTS075).
Notes and references
- S. A. Claridge, A. W. Castleman Jr, S. N. Khanna, C. B. Murray, A. Sen and P. S. Weiss, ACS Nano, 2009, 3, 244–255 CrossRef CAS PubMed.
- S. Mandal, A. C. Reber, M. Qian, P. S. Weiss, S. N. Khanna and A. Sen, Acc. Chem. Res., 2013, 46, 2385–2395 CrossRef CAS PubMed.
- J. Schmelzer Jr, S. A. Brown, A. Wurl, M. Hyslop and R. J. Blaikie, Phys. Rev. Lett., 2002, 88, 226802 CrossRef CAS PubMed.
- Q. D. Liu and X. Wang, InfoMat, 2021, 3, 854–868 CrossRef CAS.
- A. Nag, P. Chakraborty, A. Thacharon, G. Paramasivam, B. Mondal, M. Bodiuzzaman and T. Pradeep, J. Phys. Chem. C, 2020, 124, 22298–22303 CrossRef CAS.
- Y. Yong, X. Su, Q. Zhou, Y. Kuang and X. Li, Sci. Rep., 2017, 7, 17505 CrossRef PubMed.
- Y. Yong, H. Jiang, X. Li, S. Lv and J. Cao, Phys. Chem. Chem. Phys., 2016, 18, 21431–21441 RSC.
- C. S. Liu, H. An, L. J. Guo, Z. Zeng and X. Ju, J. Chem. Phys., 2011, 134, 024522 CrossRef PubMed.
- R. Łazarski, M. Sierka, J. Heinzelmann, A. Koop, R. Sedlak, S. Proch and G. F. Ganteför, J. Phys. Chem. C, 2015, 119, 6886–6895 CrossRef.
- Y. L. Yong, B. Song and P. M. He, J. Phys. Chem. C, 2011, 115, 6455–6461 CrossRef CAS.
- Z. Liu, X. Wang, J. Cai and H. Zhu, J. Phys. Chem. C, 2015, 119, 1517–1523 CrossRef CAS.
- Z. Nie, P. Guo, J. Zheng, P. Zhao, Y. Wan and Z. Jiang, Comput. Mater. Sci., 2018, 146, 134–142 CrossRef CAS.
- K. Dhaka and D. Bandyopadhyay, Dalton Trans., 2016, 45, 12432–12443 RSC.
- Y. Yong, B. Song and P. He, Phys. Chem. Chem. Phys., 2011, 13, 16182–16189 RSC.
- Y. Song, F. Fu, J. Zhang, J. Chai, X. Kang, P. Li, S. Li, H. Zhou and M. Zhu, Angew. Chem., Int. Ed., 2015, 54, 8430–8434 CrossRef CAS PubMed.
- J. Y. Wang, R. W. Huang, Z. Wei, X. J. Xi, X. Y. Dong and S. Q. Zang, Chem.–Eur. J., 2019, 25, 3376–3381 CrossRef CAS PubMed.
- M. Cao, R. Pang, Q. Y. Wang, Z. Han, Z. Y. Wang, X. Y. Dong, S. F. Li, S. Q. Zang and T. C. W. Mak, J. Am. Chem. Soc., 2019, 141, 14505–14509 CrossRef CAS PubMed.
- A. C. Reber and S. N. Khanna, Acc. Chem. Res., 2017, 50, 255–263 CrossRef CAS PubMed.
- A. C. Reber, S. N. Khanna and A. W. Castleman Jr, J. Am. Chem. Soc., 2007, 129, 10189–10194 CrossRef CAS PubMed.
- W. J. Zheng, O. C. Thomas, T. P. Lippa, S. J. Xu and K. H. Bowen Jr, J. Chem. Phys., 2006, 124, 144304 CrossRef PubMed.
- F. Liu, M. Mostoller, T. Kaplan, S. N. Khanna and P. Jena, Chem. Phys. Lett., 1996, 248, 213–217 CrossRef CAS.
- A. W. Castleman and S. N. Khanna, J. Phys. Chem. C, 2009, 113, 2664–2675 CrossRef CAS.
- A. W. Castleman Jr, S. N. Khanna, A. Sen, A. C. Reber, M. Qian, K. M. Davis, S. J. Peppernick, A. Ugrinov and M. D. Merritt, Nano Lett., 2007, 7, 2734–2741 CrossRef PubMed.
- J. Wang, W. R. Jiang, W. Y. Xie, J. P. Wang and Z. G. Wang, Sci. China Mater., 2019, 62, 416–422 CrossRef CAS.
- C. A. Mirkin and W. B. Caldwell, Tetrahedron, 1996, 52, 5113–5130 CrossRef CAS.
- F. Diederich and C. Thilgen, Science, 1996, 271, 317–323 CrossRef CAS.
- X. Roy, C. H. Lee, A. C. Crowther, C. L. Schenck, T. Besara, R. A. Lalancette, T. Siegrist, P. W. Stephens, L. E. Brus and P. Kim, Science, 2013, 341, 157 CrossRef CAS PubMed.
- P. Pyykkö, C. Clavaguéra and J.-P. Dognon, Comput. Methods Lanthanide Actinide Chem., 2015, 401–424 Search PubMed.
- T. Guo, M. D. Diener, Y. Chai, M. J. Alford, R. E. Haufler, S. M. McClure, T. Ohno, J. H. Weaver, G. E. Scuseria and R. E. Smalley, Science, 1992, 257, 1661–1664 CrossRef CAS PubMed.
- P. W. Dunk, N. K. Kaiser, M. Mulet-Gas, A. Rodríguez-Fortea, J. M. Poblet, H. Shinohara, C. L. Hendrickson, A. G. Marshall and H. W. Kroto, J. Am. Chem. Soc., 2012, 134, 9380–9389 CrossRef CAS PubMed.
- D. Wang, W. F. van Gunsteren and Z. Chai, Chem. Soc. Rev., 2012, 41, 5836–5865 RSC.
- H.-J. Zhai, Y.-F. Zhao, W.-L. Li, Q. Chen, H. Bai, H.-S. Hu, Z. A. Piazza, W.-J. Tian, H.-G. Lu, Y.-B. Wu, Y.-W. Mu, G.-F. Wei, Z.-P. Liu, J. Li, S.-D. Li and L.-S. Wang, Nat. Chem., 2014, 6, 727–731 CrossRef CAS PubMed.
- J. Wang, T. Yu, Y. Gao and Z. Wang, Sci. China Mater., 2017, 60, 1264–1268 CrossRef CAS.
- X. Dai, Y. Gao, W. Jiang, Y. Lei and Z. Wang, Phys. Chem. Chem. Phys., 2015, 17, 23308–23311 RSC.
- T. Yu, Y. Gao, D. Xu and Z. Wang, Nano Res., 2018, 11, 354–359 CrossRef CAS.
- W. Xie, W. Jiang, Y. Gao, J. Wang and Z. Wang, Chem. Commun., 2018, 54, 13383–13386 RSC.
- Y. Yang, Z. Zhang, E. S. Penev and B. I. Yakobson, Nanoscale, 2017, 9, 1805–1810 RSC.
- G. te Velde, F. M. Bickelhaupt, E. J. Baerends, C. Fonseca Guerra, S. J. A. van Gisbergen, J. G. Snijders and T. Ziegler, J. Comput. Chem., 2001, 22, 931–967 CrossRef CAS.
- J. P. Perdew, K. Burke and M. Ernzerhof, Phys. Rev. Lett., 1996, 77, 3865–3868 CrossRef CAS PubMed.
- E. Van Lenthe and E. J. Baerends, J. Comput. Chem., 2003, 24, 1142–1156 CrossRef CAS PubMed.
- S. Grimme, J. Antony, S. Ehrlich and H. Krieg, J. Chem. Phys., 2010, 132, 154104 CrossRef PubMed.
- W. Y. Xie, Y. Zhu, J. P. Wang, A. H. Cheng and Z. G. Wang, Chin. Phys. Lett., 2019, 36, 116401 CrossRef CAS.
Footnote |
† Electronic supplementary information (ESI) available: The relative energies, molecular orbitals diagram and density of states. See https://doi.org/10.1039/d2ra01591a |
|
This journal is © The Royal Society of Chemistry 2022 |
Click here to see how this site uses Cookies. View our privacy policy here.