DOI:
10.1039/D2RA01571D
(Review Article)
RSC Adv., 2022,
12, 15385-15406
Pyridine: the scaffolds with significant clinical diversity
Received
10th March 2022
, Accepted 27th April 2022
First published on 20th May 2022
Abstract
The nitrogen-bearing heterocycle pyridine in its several analogous forms occupies an important position as a precious source of clinically useful agents in the field of medicinal chemistry research. This privileged scaffold has been consistently incorporated in a diverse range of drug candidates approved by the FDA (Food and Drug Administration). This moiety has attracted increasing attention from several disease states owing to its ease of parallelization and testing potential pertaining to the chemical space. In the next few years, a larger share of novel pyridine-based drug candidates is expected. This review unifies the current advances in novel pyridine-based molecular frameworks and their unique clinical relevance as reported over the last two decades. It highlights an inclination to the use of pyridine-based molecules in drug crafting and the subsequent emergence of several potent and eligible candidates against a range of diversified diseases.
1. Introduction
Pyridine (C5H5N), an isostere of benzene, is used as a precursor for synthesizing target pharmaceuticals and agrochemicals. Besides, it is a key solvent and reagent. The term ‘pyridine’ is a derivative from a Greek word, and is the grouping of two words “pyr” denotes fire and “idine” is applicable for aromatic bases. The initial pyridine scaffold was isolated from picoline by Anderson in 1846. Later, Wilhelm Korner (1869) and James Dewar (1871) discovered the structure of pyridine. William Ramsay had prepared a pyridine-based compound by mixing acetylene and hydrogen cyanide under red-hot conditions. In the formulation of pharmaceuticals, pyridine is one of the nuclear components of more than 7000 existing drug molecules of medicinal importance. Pyridine scaffolds are found in nature, mainly from plant resources, such as alkaloids and one of the most effective cholinergic drugs like atropine (Atropa belladonna), which holds a saturated pyridine ring.1,2 In many enzymatic reactions, prosthetic pyridine nucleotide is engaged in numerous oxidation–reduction processes. Many vitamins, such as pyridoxine, niacin and nicotine, have high potency and selectivity in many biological systems.
Topical isolation of the structurally-modified natural products diploclinide and nakinadine contain pyridine moiety. Atazanavir (Rey-ataz) and imatinib mesylate (Gleevec) are medicinally-active pyridine derivatives. These drugs are useful against human immune deficiency virus (HIV) and chronic myelogenous leukemia. The chief constituent of coenzyme nicotinamide adenine dinucleotide (NADH) and nicotinamide adenine dinucleotide phosphate (NADPH) are prepared either from the 1-tryptophan kynurenine pathway in animals or the glyceraldehyde-3-phosphate and aspartic acid pathway in different plants. Anabasine, ricinine and arecoline are derived from nicotinic acid (Fig. 1), while in industrial scale-up processes they are prepared by using a mixture of crotonaldehyde, formaldehyde and ammonia in a gas phase.
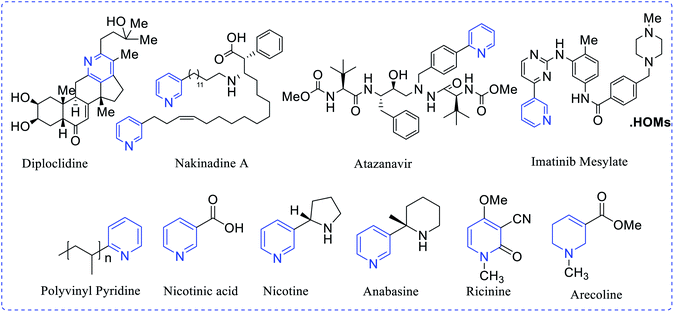 |
| Fig. 1 Examples of naturally occurring pyridine derivatives. | |
Ammonia has been used as the nitrogen source in countless protocols, such as [5 + 1] condensation with 1, 5 dicarbonyls (Scheme 1). Autoxidation is an important factor for aromatization in different condensation processes. In the [2 + 2 + 1 + 1] Hantzsch pyridine synthesis, ammonia is regularly used (Scheme 2). Further, the preparation of pyridine depends on alkyl or vinyl amine and is a type of condensation reaction between 1, 3 dicarbonyl derivatives and vinylogous amide (Scheme 3).
 |
| Scheme 1 Synthesis of substituted pyridines by a condensation method. | |
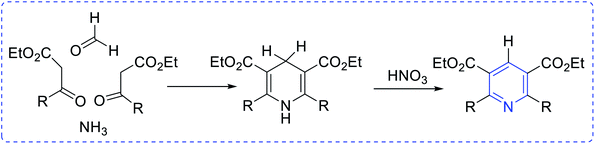 |
| Scheme 2 [2 + 2 + 1 + 1] multicomponent synthesis of pyridine derivatives. | |
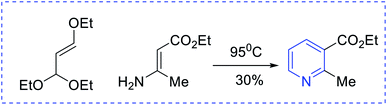 |
| Scheme 3 A one-pot synthesis of substituted pyridines from 1,3-dicarbonyl and vinylogous amide. | |
Boger et al. have synthesized [4 + 2] pyridine by introducing enamines and triazine via an inverse electron demand aza-Diels–Alder reaction (Scheme 4). The key approaches are 6π-electrocyclization (Scheme 5) using a [2 + 2 + 2] cobalt-mediated synthesis of substituted pyridines (Scheme 6).3
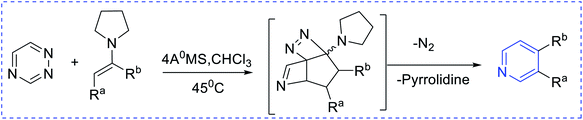 |
| Scheme 4 Synthesis of pyridine derivatives via the aza-Diels–Alder method. | |
 |
| Scheme 5 The [4 + 2] rhodium-mediated synthesis of pyridines. | |
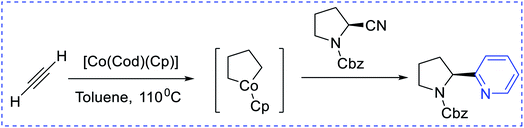 |
| Scheme 6 [2 + 2 + 2] cobalt-mediated synthesis of substituted pyridines. Cbz = carbobenzyloxy, Cod = cyclooctadiene. | |
2. Pharmacology
2.1 Antibacterial activity
Ivachtchenko et al. synthesized 2-imino-5-hydroxymethyl-8-methyl-2H-pyrano[2,3-c] pyridine-3-(N-aryl) carboxamides (1) that exhibited potency and selectivity (12.5–25 lg mL−1) toward fungal and bacterial straining and was also highly active in comparison with marketed drugs.4 Suksrichavalit et al. prepared the pyridine-based copper complexes (2–5) and evaluated them for superoxide scavenging and antimicrobial properties. These complexes showed superoxide dismutase activity in the range of 49.07–130.23 mM. One of copper complex based nicotinic acid with 2-hydroxypyridine showed inhibitory concentration of 49.07 mM.5 Bhatia et al., reported a set of pyridine scaffolds (6a–6h) obtained by reacting pyridyl amine with the aromatic aldehyde. All the synthesized compounds were screened for their antibacterial and antitubercular activities in the range of 3.12–6.25 μg mL−1.6 Leal et al. have evaluated the biological and theoretical studies of 4-(arylamino)-1-phenyl-1H-pyrazolo [3,4-b]pyridine-5-carboxylic acids (7), which indicated that compound 7 exhibited potent antibacterial efficacy against a drug-resistant S. epidermidis. The minimum inhibitory concentration of the prepared compound showed similar pharmacological activity in comparison with oxacillin and better potency with chloramphenicol.7 The thienopyridine and other pyridines (8–16), as developed by Zavyalova, were found to be effective against both Gram-positive bacteria, S. aureus and Gram-positive bacteria as E. coli, P. aeruginesa and P. vulgaris8 (Fig. 2). Sharma et al. reported a substituted pyridine analog (17) and evaluated its pharmacological properties such as antibacterial activity.9
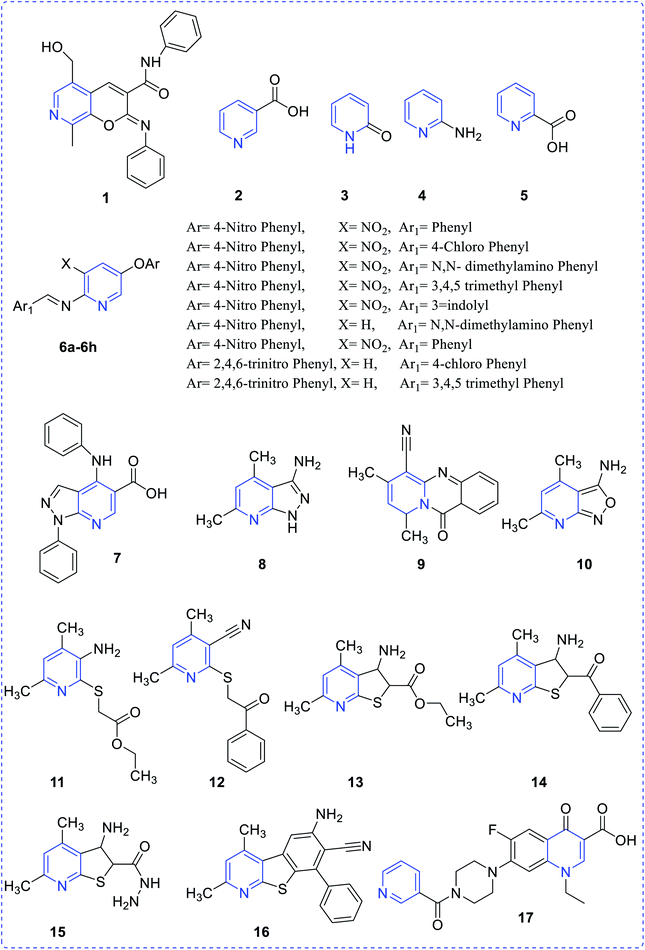 |
| Fig. 2 Some examples of most anti-bacterial agent of substituted pyridines. | |
2.2 Antiviral activity
Bernardino et al. synthesized a series of 4-(phenylamino)thieno[2,3-b]pyridine derivatives (18) and evaluated their inhibitory property against Herpes simplex virus type 1 (HSV-1). All the synthesized derivatives were chemically diverse in nature but showed moderate potency. To enhance the efficiency, similar type of derivatives with a new series of 4-(phenylamino)-1H-pyridazino [3,4-b]pyridine derivatives (19) were prepared and these analogues exhibited better anti-HIV activity, as compared to those in the earlier series.10
Attia et al. reported the preparation of a few pyridine ribosides (20) and subsequently inferred their potentiality against HIV infection. 1-(β-D-ribofuranosyl)-pyridine-2-thione with the substituent (phenyl, 4-chlorophenyl, methylphenyl, 4-furyl) was found to be the most active one.11 Chezal et al. have synthesized a series of imidazo pyridine scaffolds (21) and evaluated the antimicrobial efficacy towards classical swine fever virus and the border disease virus which belongs to the genus Pestivirus.12 A few thiazolo pyridine-based oxime exhibit better potency towards influenza B-Mass virus. In continuation, the above compounds also showed better selectivity and activity against HIV. Many naphthyridine compounds have been evaluated for their antibacterial activity and oximes-pyridine scaffolds as antidotes against organophosphorus poisoning (22).13 Hydrazone of 3 and 4-acetyl pyridine, which has anti-tumor activity, is capable of inhibiting the replication of RNA (+) and RNA (−) strains of hepatitis C-virus (HCV) (23). Bi-pyridinyl derivatives complex with ruthenium displayed antiviral property against HCV (24).14 Chen et al. synthesized chalcone scaffolds, which contained malonate and pyridine moieties (25a–25v). The compound 25i and 25n exhibited notable curative activities15 along with potent antiviral activity towards cucumber mosaic virus (CMV) at 500 lg mL−1 with EC50 values of 186.2 and 211.5 lg mL−1, respectively. The results are comparatively better than that of ningnanmycin (330.5 lg mL−1) (Scheme 7).
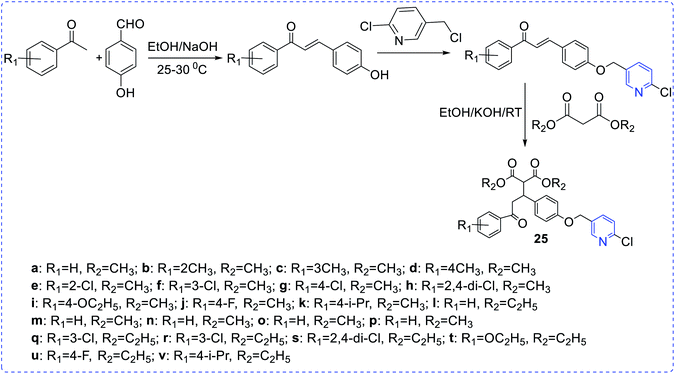 |
| Scheme 7 Synthesis of most active antiviral agent chalcone conjugate with malonate and pyridine. | |
Hartwich et al. synthesized phosphonylated triazolo[4,5-b]pyridine(1-deaza-8-azapurine) (26a), imidazo[4,5-b]pyridine (1-deazapurine) (26b) and imidazo[4,5-b]pyridin-2(3H)-one(1-deazapurin-8-one) (26c) were evaluated for antimicrobial properties. Compound 26c exhibited minimal activity (EC50 = 61.70 μM) when exposed to the varicella-zoster virus Oka strain in HEL cells16 while the other two compounds exhibited marginal activity (Fig. 3).
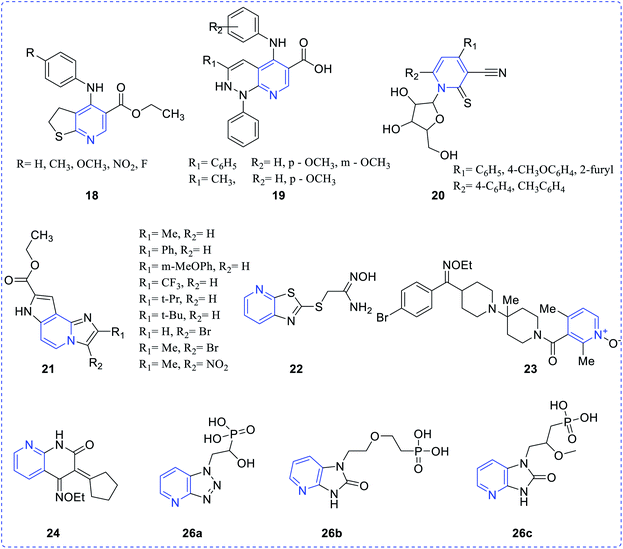 |
| Fig. 3 Representative examples of antiviral activity of compounds containing pyridine as the basic unit. | |
2.3 Anticancer activity
Nicolaou et al. proposed pyridine epothilones and evaluated their cytotoxicity against a number of human cancer cell lines. Compounds (27) and (28) exhibited the highest activity, which may be ascribed to the ring nitrogen and the pyridyl methyl group at 5th and 6th positions, respectively.17 Here, it is worth mentioning that the presence of pyridine nitrogen has traditionally been assumed to be protonated in enzyme active sites, with the protonated pyridine ring providing resonance stabilization of carbanionic intermediates. Therefore, the ring nitrogen in the pyridyl system plays a crucial role in controlling the enzyme activity and hence impacts the potent biological attributes of analogues.
A series of a few 2,6-diaryl-substituted pyridines (29) was synthesized by Jong-Keun Son et al. and reported their cytotoxic effect against several human cancer cell lines. Compounds with their substituents revealed promising cytotoxic topoisomerase I inhibitory activity.18 Hayakawa et al. prepared a series of imidazo [1,2-a] pyridines scaffolds (30) and tested their p110a (emerging target for cancer therapy) inhibitory property. Only, thiazolyl imidazo [1,2-a] pyridine compound showed inhibition of tumor cell growth.19
A series of 2-amino-3-(3′,4′,5′-trimethoxybenzoyl)-6-substituted-4,5,6,7-tetrahydrothieno [2,3-c] pyridine derivatives (31) was evaluated for anticancer properties against several cancer cell lines, as reported by Romagnoli et al. These substituted compounds exhibited promising anti-proliferative activity. The mechanistic study suggested that the inhibition of tubulin polymerization may affect the G2 cell cycle.20 Liou et al. established a new indoline-sulfonamide (32) derivative and evaluated its anticancer properties for various human cancer cell lines via disruption of microtubule.21 The cobalt(III) pyridine complexes (33) and (34) as reported by Nagababu et al.22 efficiently interact with DNA. These complexes showed their photocleavage ability on plasmid DNA pBR322 upon irradiation at 365 nm (Fig. 4).
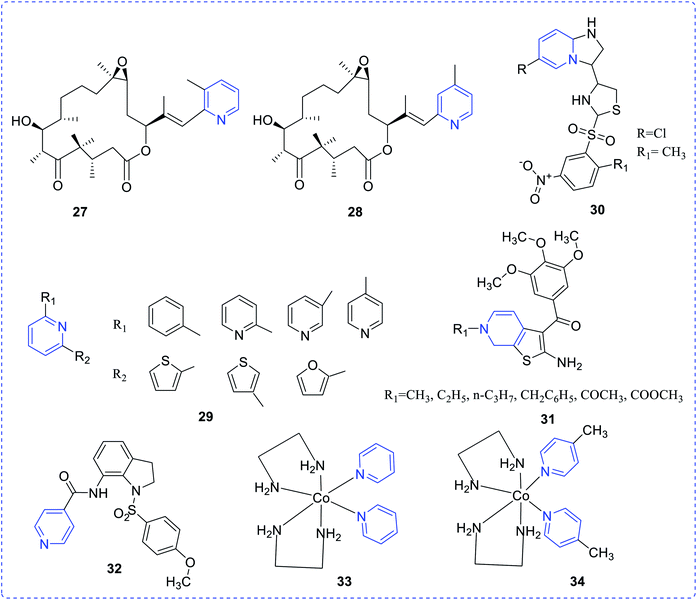 |
| Fig. 4 Representative examples of the most active anticancer agents. | |
El Naggar et al. synthesized pyridine-ureas (35a–n) and evaluated the in vitro anti-proliferative activity against MCF-7. The observed IC50 values are micromolar region, which is beneficial for getting good pharmacological activity (Scheme 8).23
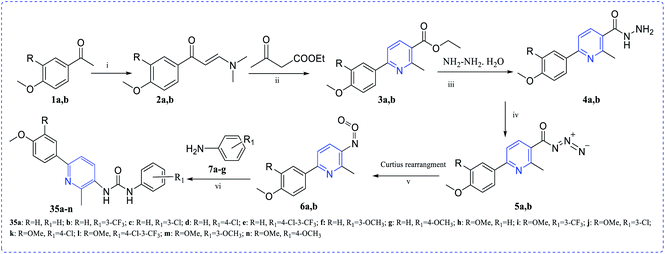 |
| Scheme 8 Reagents and conditions for synthesizing of most active anticancer agent pyridine-ureas 35a–n; (i) DMF-DMA, xylene, reflux 7 h; (ii) NH4OAc, AcOH, reflux 4 h; (iii) methanol, NH2NH2·H2O, reflux 2 h; (iv) NaNO2, AcOH, stirring 2 h; (v) xylene, reflux 1 h; (vi) xylene, reflux 3 h. | |
Ramya et al. synthesized a series of curcumin-conjugated imidazo[1,2-a]pyridine (36a–t) as a tubulin polymerization inhibitor. Among these, compounds 36e, 36r and 36t demonstrated potent growth inhibition and 36t demonstrated low IC50 values 1.7–2.97 μM with different cancer cell lines. Moreover, 36t showed a good cytotoxicity profile on PC-3, HGC-27 and HeLa cell lines with IC50 values of 2.11 ± 0.27 μM, 2.21 ± 0.25 μM and 2.53 ± 0.01 μM, respectively (Scheme 9 and Fig. 5).24
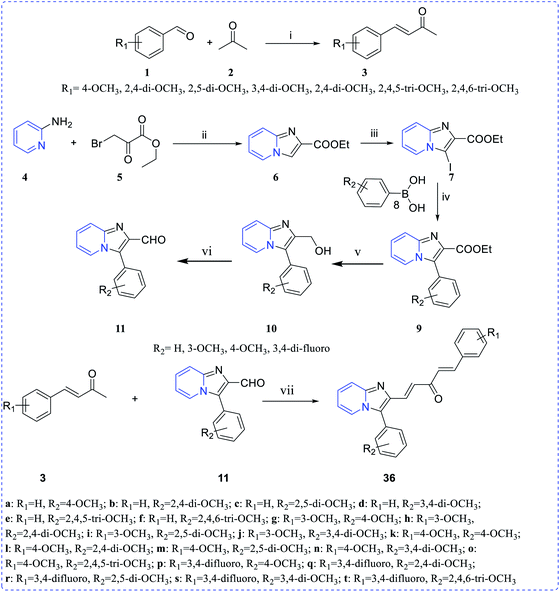 |
| Scheme 9 Synthesis of curcumin-inspired imidazo[1,2-a]pyridine analogues 36a–t; reagents and conditions: (i) 15% NaOH, ethanol, 0 °C – rt, 2–3 h, 71–85%. (ii) Ethanol, reflux, 1 h, 74.3%; (iii) NIS, rt, 1 h, 65.1%; (iv) Pd(PPh3)4, Na2CO3, dioxane : H2O = 8 : 2, 75 °C, 2–6 h, 65–73%; (v) LiAlH4, THF, 0 °C – rt, 1 h, 67–71%; (vi) DMP, DCM, 0 °C, 1 h, 65–74%; (vii) CH3ONa, ethanol, rt, 15 min, 63–82%. | |
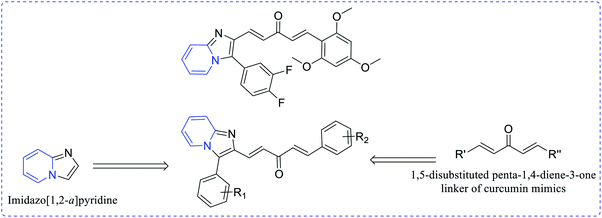 |
| Fig. 5 Most active anticancer agent curcumin conjugated imidazo[1,2-a]pyridine derivatives. | |
Khalaf et al. synthesized a number of dipyridyl (37–39) or pyridinyl sugar hydrazones linked to thienyl or the methylfuryl rings in addition to pyridine thioglycosides combining naphthyl and furyl systems via a multistep process. The prepared compounds showed higher cytotoxicity profiles toward various cancer cell lines. Anticancer activity of the products was tested against human prostatic adenocarcinoma (PC3), adenocarcinomic human alveolar basal epithelial (A549) and human colorectal carcinoma (HCT116) cell lines in addition to their effect on human normal retinal pigmented epithelial cell line (RPE1) using the MTT assay (Schemes 10–12).25
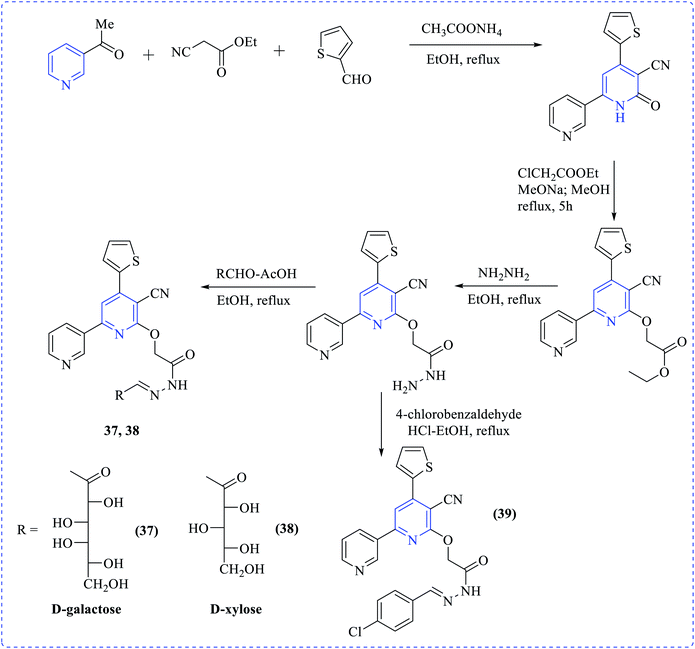 |
| Scheme 10 Synthesis of most active anticancer agent dipyridinyl-thiazole sugar and acrylidine derivatives. | |
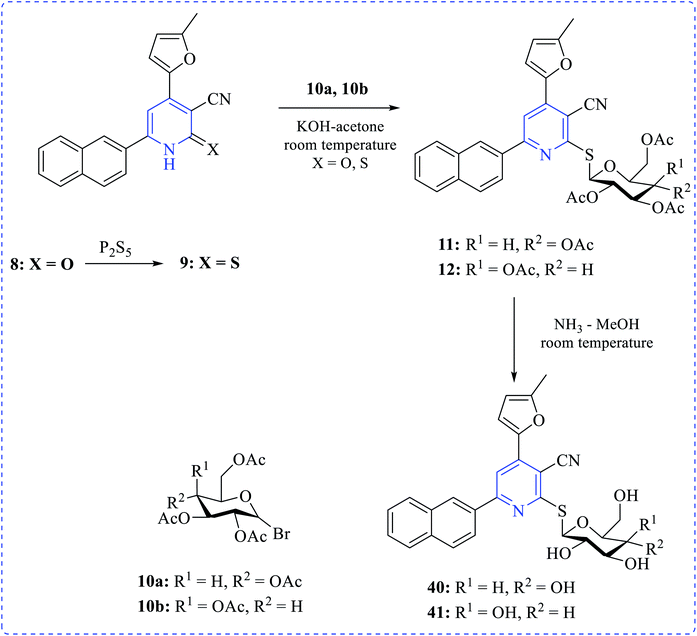 |
| Scheme 11 Synthesis of most active anticancer agent heteroaryl-substituted pyridine glycosides. | |
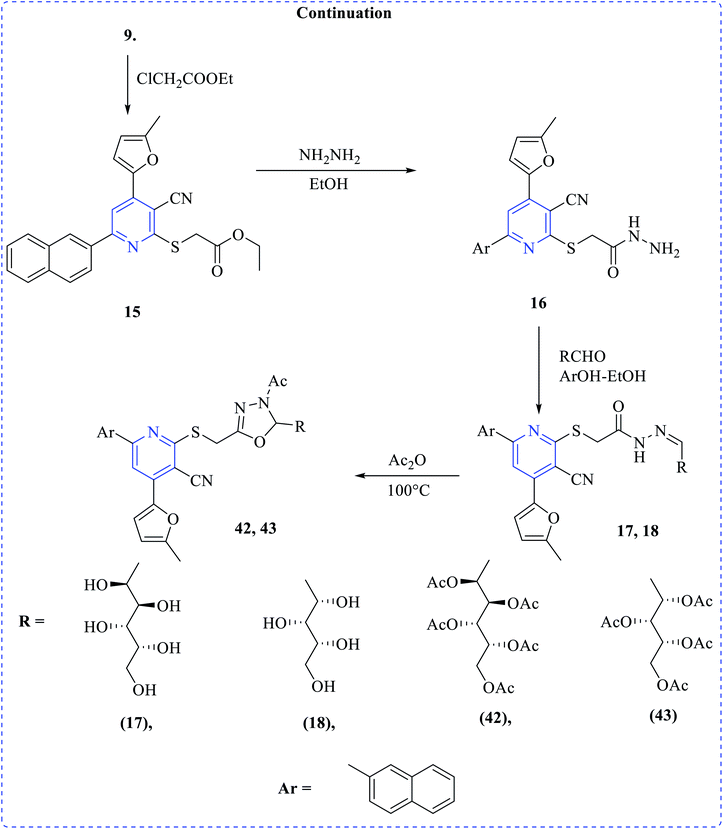 |
| Scheme 12 Synthesis of most active anticancer agent pyridinyl furan sugar derivatives. | |
Thongaram et al. developed a tandem reaction between 3-hydroxy-2-methylpyridine 1-oxide and substituted 2-fluorobenzaldehydes (44), which involved Knoevenagel condensation and nucleophilic aromatic substitution (Fig. 6). This type of reaction provides easy contact with pyridine 1-oxide derivatives with good yields. Among these, a few are highly potent and selective toward HCT-116 cell lines with IC50 values 24.95–45.80 μM.26
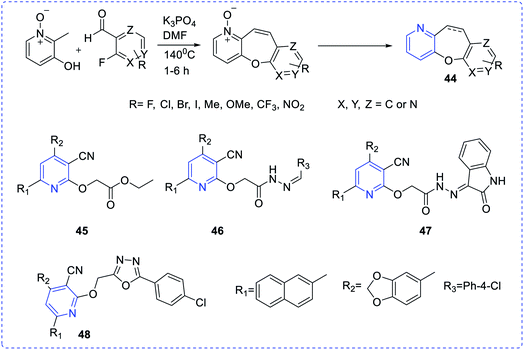 |
| Fig. 6 Synthesis of most active anticancer agent pyridine derivatives. | |
Mansour et al. investigated the cytotoxicity against MCF-7 and MDA-MB-231 cell lines for some new chemical entities at different concentrations (0–100 μg mL−1) via the MTT assay. Compounds (45–48) were found with the lowest half-maximal inhibitory concentrations (IC50) against those cell lines by cytotoxicity assays.27 Rani et al. have synthesized a new set of amide derivative imidazopyridine (49a–49j) compounds exhibiting anticancer activities when exposed to the breast (MCF-7 and MDA-MB-231) cancer, lung (A549) cancer, and prostate (DU-145) cancer cell lines via MTT assay (etoposide as the standard reference drug). Among those compounds, 49j showed the highest anticancer activities towards MDA-MB-231, MCF-7, A549 and DU-145 cell lines with the IC50 values of 0.95 ± 0.039 μM, 0.021 ± 0.0012 μM, 0.091 ± 0.0053 μM, and 0.24 ± 0.032 μM, respectively28 (Scheme 13).
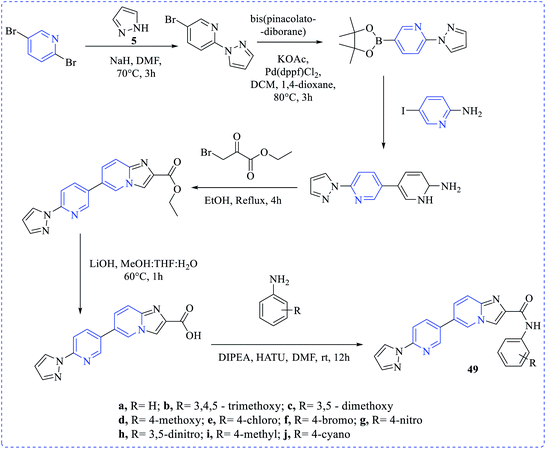 |
| Scheme 13 Synthesis of most active anticancer agent amide derivatives of imidazopyridine. | |
Barresi et al. reported vinyl-substituted pyridine (50, 51) compounds showing the antitumor activities (in vitro) of the synthesized compounds.29 The compounds 50a–50d and 51a, 51c, 51e showed in vitro antitumor antiproliferative activity, particularly evident in the MCF7 mammary adenocarcinoma cells. Son et al. synthesized and reported diaryl-based pyridine (52) compound having antitumor properties.30 Cocco et al. reported the synthesis of antitumoral complex dicyanopyridine (53) and (in vitro).31 Ester and amide of 2-arylamino-6-trifluoromethyl-3-pyridinecarboxylic acids (54,55) possess antitumor properties, as reported by Onnis et al.32 (Fig. 7).
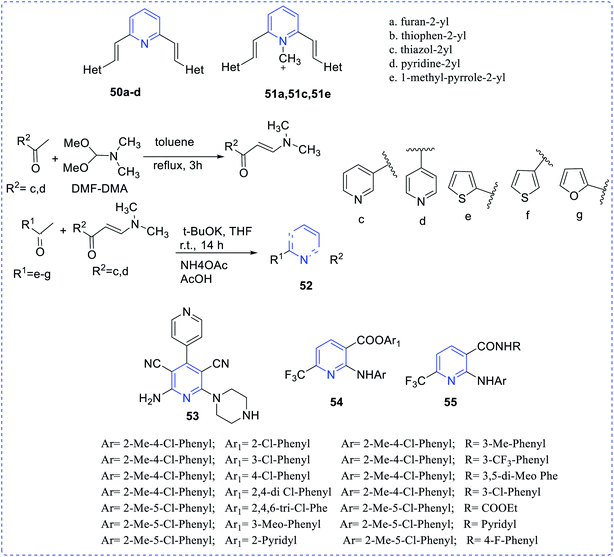 |
| Fig. 7 Most active anticancer agent 6-di-[2-(heteroaryl)vinyl]pyridines. | |
2.4 Antidiabetic activity
Type 1 diabetes mellitus (T1DM) can be produced with Streptozotocin (STZ) by the pancreatic islet β-cell destruction. Type 2 diabetes models are also going to be developed using STZ. Several animal species e.g. the mouse, rat, and monkey, are more sensitive to the pancreatic β-cell cytotoxic effects of STZ compared to a rabbit. Animals such as rats and mice are being used for assessing the pathological consequences and potential therapies/treatments in diabetes patients.33 Bahekar et al. reported two sets of pyrrolo-based pyridine derivatives and thieno-based pyridines, respectively (56–58). All the compounds exhibit in vitro glucose-dependent insulinotropic activity towards RIN5F cell lines.34 Kim et al. reported the synthesis of a few thiazolidinedione-based pyridines compounds (59) and showed the hypoglycemic activity and hypolipidemic activity of the synthesized compounds.35 Suresh et al. developed isoxazolo-based pyridine (60–64) heterocycles by an environmentally benign method. The synthesized compound (63) showed the highest inhibition of α-amylase activity (IC50 = 56.043 μg mL−1).36 Adi et al. reported a new set of antimicrobial complexes (Scheme 14) 65a–p, which showed in vitro yeast α-glucosidase inhibitory activity with IC50 values ranging from 101.0 ± 2.0 to 227.3 ± 1.4 μM, which was higher than that of the standard drug acarbose (IC50 = 750.0 ± 1.5 μM) (Fig. 8 and 9).37
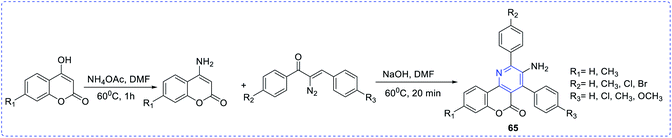 |
| Scheme 14 Synthesis of 3-amino-5H-chromeno[4,3-b]pyridine-5-ones derivatives. | |
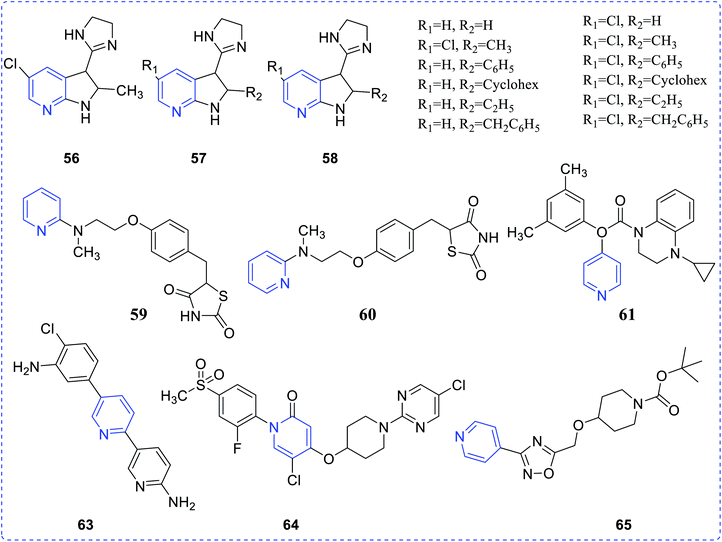 |
| Fig. 8 Most active anticancer agentImidazol pyridine and bipyridine derivatives. | |
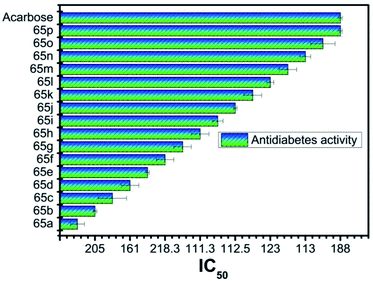 |
| Fig. 9 In vitro yeast α-glucosidase inhibition activity with IC50 values. | |
Ma et al. synthesized a new class of complexes of thieno-pyridine derivatives, which act as hepatic gluconeogenesis inhibitors that were found to be effective in type 2 diabetes mellitus (Fig. 10). The structure–activity relationship (SARs) study proved that thienopyridine core can increase the antimicrobial potency when replaced with –CF3, which led to the synthesis of a couple of complexes 66e (IC50 = 16.8 μM) and 67d (IC50 = 12.3 μM) that can inhibit the production of hepatic glucose. Added to this, complex 61e also showed similar activity and a decrease in the expression of the mRNA transcription level of gluconeogenic genes, including hepatic phosphoenolpyruvate carboxykinase (PEPCK) and glucose-6-phosphatase (G6Pase).38
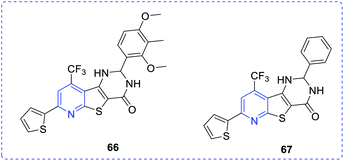 |
| Fig. 10 Most active anticancer agent thieno[2,3-b]pyridine derivatives. | |
2.5 Antifungal activity
Ozdemir et al. reported a group of eight novel pyridine derivatives showing antifungal activity when exposed to a panel of ten human pathogenic Candida species (Fig. 11). Among them, compound (68) exhibited strong inhibition (MIC 0.016 mg mL−1) against the screened Candida species.39 The synthesis and antifungal activities of a few 3-aryl-5-(pyridin-3-yl)-4,5-dihydropyrazole-1-carbothioamide (69) were reported by Shekarchia et al.40
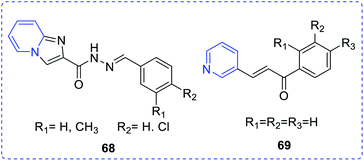 |
| Fig. 11 Most active antifungal agent pyridine derivatives. | |
Pyridine quaternization was performed using the betulin triterpenoids as the lipophilic substrates and Tempo + Br3 − cation. The application of Tempo + Br3 − cation provides in situ substrate bromination, which avoids the stage of additional halogenations. This process significantly reduces the number of stages, and it increases the final yield of the reaction product (Fig. 12). The obtained quaternized pyridine derivatives of betulin triterpenes showed good antibacterial and antifungal activities compared to the initial compounds.41–44
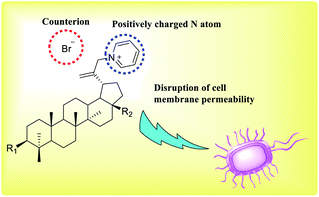 |
| Fig. 12 Quaternized pyridine derivatives of betulin triterpenes demonstrate antibacterial and antifungal activities. | |
2.6 Anti-inflammatory and anti-mycobacterial studies
A few diversified amides upon condensing with 2/4-acetyl pyridine connected to imidazo[1,2-a]pyridines (70) were evaluated for their anti-inflammatory property. Both the acetylated compounds exhibited an excellent anti-inflammatory activity.45–47 Pavlova et al. reported nicotinic acid amides derivatives to have both anti-inflammatory and antimicrobial activities.48 Compound 71 exerted the highest activity. Mamolo et al. synthesized and evaluated antimycobacterial activities of a series of pyridine derivatives (72–74).49,50 Navarrete-Vazquez et al. synthesized and investigated the antimycobacterial activity of pyridines (75) compounds substituted with oxadiazol (Fig. 13).51
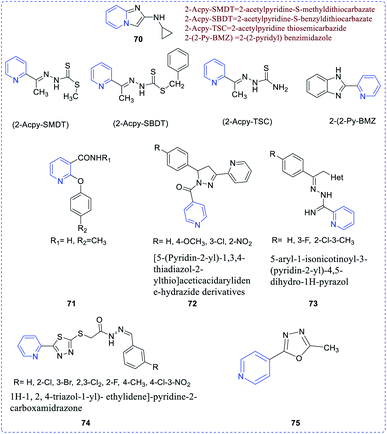 |
| Fig. 13 Structures of 2/4-acetyl pyridine connected to imidazo[1,2-a]pyridines. | |
2.7 Antimicrobial activity
Goda et al. reported and demonstrated the antimicrobial property of a set of novel pyrazolo fused pyridine complexes (76).52 A series of hydroxymethyl-pyrano-based carboxamides complexes (77) was synthesized by Zhuravel et al. The antimicrobial study revealed that the compound with the following substituents was highly active than the rest.53 Gaonkar et al. reported the synthesis of phenyl-based oxadiazoles complex (78) and established the highest antimicrobial activity for the compound.54 Yar et al. synthesized and reported phenyl substituted pyridylmethanone complex (79), which exhibited both antimicrobial and anti-HIV activities.55 Gaonkar et al. reported pyridine-based isooxazoline complexes (80) and demonstrated their antimicrobial activities56 (Fig. 14).
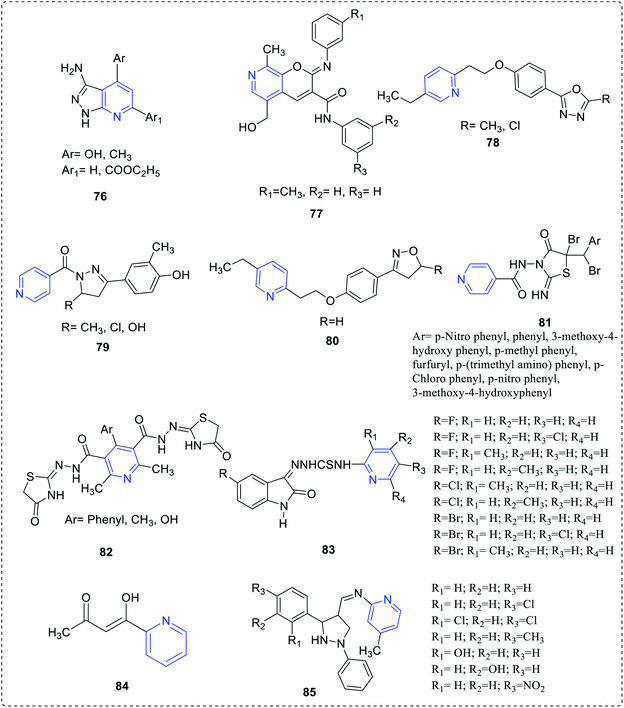 |
| Fig. 14 Structure of pyridines Moiety for antimicrobial. | |
Mishra et al. reported a novel carboxamido pyridyl-based thiazolidinediones complex (81) showing antimicrobial activity.57 Mehta et al. reported that some of the thiazolidinone contains pyridine (82), which is a new class of antimicrobial agents.58 Aanandhi et al. reported a group of pyridine based thiosemicarbazide complexes (83) exhibiting antimicrobial activity.59 The antimicrobial activity of compounds indicated that few derivatives showed the highest activity against B. subtilis NCIM2063, S. aureus NCIM2079, E. coli NCIM2065, P. aeruginosa NCIM2200, C. albicans NCIM3102 and A. niger. Riahi et al. prepared and demonstrated antimicrobial activity for the pyridyl-based carbonyl complex.60 Vora et al. reported and examined the antimicrobial activity of Schiff base methyl pyridine amine (85).61
2.8 Anti-tubercular activity
Sriram et al. synthesized phenyl-based thiourea (86) and studied its in vitro antitubercular activity.62 Compound 86 showed the highest antitubercular activity. Herzigova et al. studied 4-benzylsulfanylpyridine-2-carbohydrazides (87) and established their potential anti-tubercular property63 (Fig. 15).
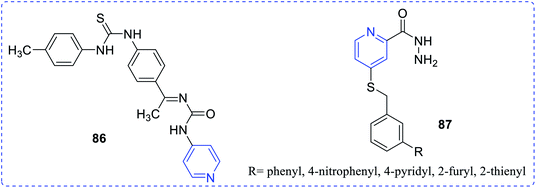 |
| Fig. 15 Structure of 4-benzylsulfanylpyridine-2-carbohydrazides. | |
2.9 Antichagasic, anti-oxidant, anti-malarial, anti-amoebic agents, iron overload disease and enzyme inhibition
Endemic of Chagas' disease can keep a hundred million people at risk and about twenty million people are infected chronically. Trypanosoma cruzi parasite is responsible for this disease. To prevent this, Dias et al. synthesized antichagasic 1H-pyrazolo [3,4-b] pyridine series compounds (88) (Fig. 16).64
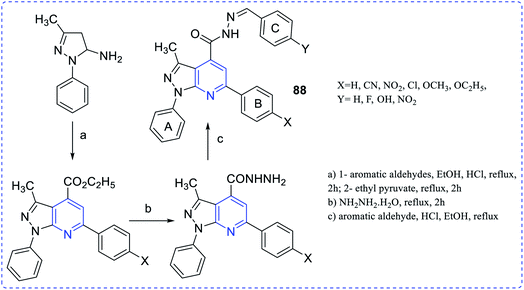 |
| Fig. 16 Synthesis of 1H-pyrazolo [3,4-b]pyridine analogues. | |
The synthesis and free radical scavenging activity of thiazolo [3,2-a] pyridines (89) were reported by Feng Shi et al. (Fig. 17).65
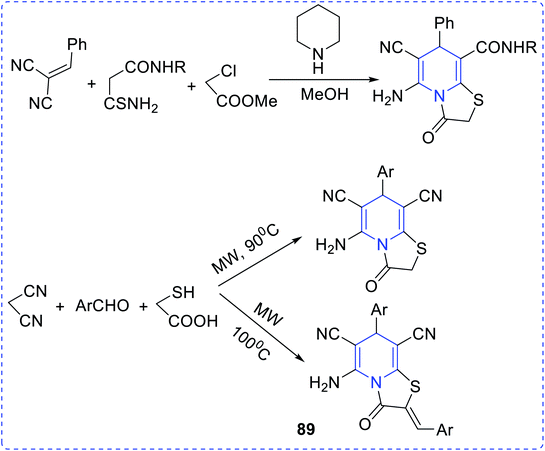 |
| Fig. 17 Synthesis of thiazolo pyridine. | |
The substituted thiazolopyridines showed significant superoxide dismutase (SOD) property. Studies show that molecular compounds with the lowest atomic polarizability have the highest DPPH activity.66 Timperley et al. have synthesized and evaluated the activity of mono- and bis-quaternary pyridine salts (90) in organophosphorus poisoning treatment (Fig. 18).67 An effective antimalarial (a chloroquine-susceptible strain of Plasmodium falciparum), a hybrid concept of quinoline-linked pyridine (91,92) was developed.68 The biological activity may be attributed to haem polymerization inhibition (HPI). It has been observed that ligands derived from acetyl pyridine possess anti-amoebic activity. However, it was observed that the anti-amoebic activity drastically increases when these ligands are coupled with ruthenium(II) to form a complex structure.69 The antiamoebic activity observed in complex (93) was much higher than that of the commercially available standard drug, metronidazole. The vanadium complex with 2-acetylpyridine exhibited higher amoebicidal activity (IC50 value = 1.68–0.40) than the commercially available metronidazole (IC50 value = 1.81), whereas only the ligand cannot act as the antiamoebic agent. The pyridine derivative, which was synthesized by the reaction between pyridine-2-carboxaldehyde isonicotinoyl hydrazone (PCIH) with iron, produces a significantly stable complex. The synthesized compound acts as a chelating agent. Zeglis et al. reported a series of imidazo[1,2-a]pyridine derivatives synthesized to establish their cholesterol acyltransferase inhibition properties. Compounds (94–98) showed greater inhibitory properties compared to the rest of the synthesized compounds.70–72
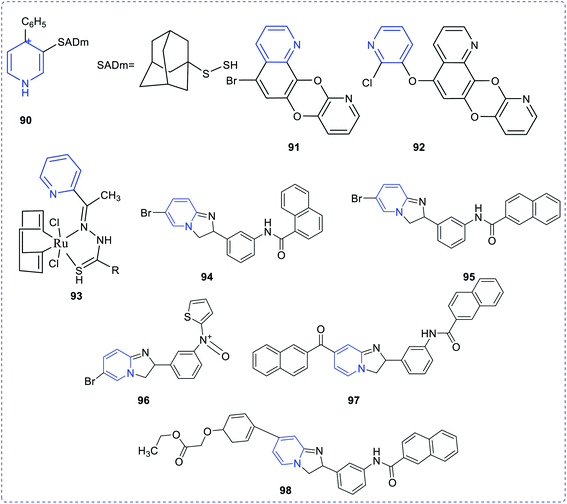 |
| Fig. 18 Structures of pyridine compounds for various pharmacological evaluation. | |
Some medicinally active pyridine-based analogues against various biological properties are summarized in the table given below (Table 1).
Table 1 Medicinally potent pyridine-based compounds against various biological attributes.73–80
Compound |
Activity |
Potency |
Mechanism of Action |
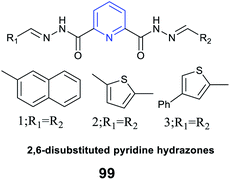 |
Cytotoxic activity against HT-29 cell line |
IC50 = 6.78, 8.88 and 8.26 μM respectively |
Morphological changes of HT-29 and ISH cells and caspase-3 activation |
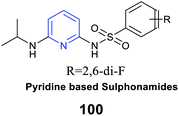 |
Antidiabetic action |
IC50 of alpha amylase inhibition is 54.18 ± 0.150 μg mL−1 |
Inhibition of α-amylase |
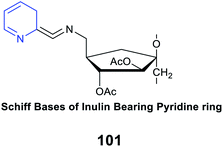 |
Antifungal activity |
An inhibitory index of 77.0% against botrytis cinerea at 2.0 mg mL−1 |
Interaction with anionic components of the cell membranes, i.e., glucan, mannan, proteins, and lipids, to destroy the cell membranes or to form an impervious layer preventing the transport of essential nutrients from entering the cell |
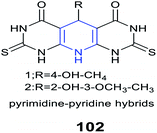 |
Anti-inflammatory activity |
COX-2 inhibitory activity in a range (IC50 = 0.25–0.89 mM) |
Competitive selective COX-2 inhibitors |
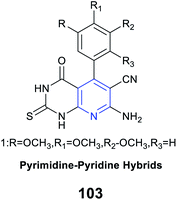 |
Ulcerogenic liability |
High COX-2 selectivity with COX-2 S.I. = 17.08 |
Competitive selective COX-2 inhibitors |
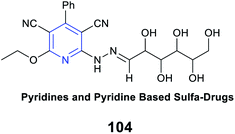 |
Antimicrobial activity |
ZOI value 34 mm (more than the standard drug cefotaxime) against E. coli |
Cell wall disruption |
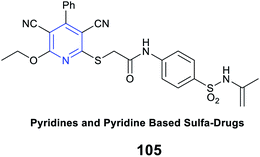 |
Antimicrobial activity |
ZOI value 34 mm (more than the standard drug cefotaxime) against Pseudomonas aeruginosa |
High cell permeability efficacy |
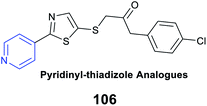 |
Antitubercular activity |
Inhibitory activity of 0.06 μM against the MDR H37RV strain |
Inhibition of RNA synthesis |
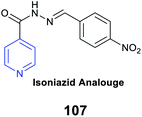 |
Antitubercular activity |
Antimycobacterial activity of 1.2 μg mL−1 against the MTB H37RV strain |
Strong binding to the DNA-dependent RNA polymerase |
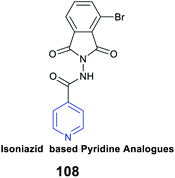 |
Antimycobacterial activity |
IC50 of 3.2 μM against the H37Rv strain |
Inhibition of RNA synthesis |
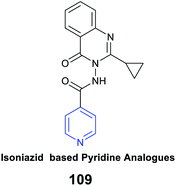 |
Antimycobacterial activity |
IC50 of 1.5 μM against the H37Rv strain |
Inhibition of RNA synthesis |
3. Pyridine-based scaffolds in marketed available drugs recently patented potent molecules and in bioactive natural products
Pyridine-based heterocycles are one of the most widely engaged pharmacophores in the field of drug development, mainly due to their intense pharmacological properties against various maladies, which resulted in the discovery of several therapeutic agents. Their incredible therapeutic applications have persuaded researchers to decorate a large number of biologically effective composites with pyridine to cure a variety of ailments. Several studies revealed that the pyridine moiety present in a drug molecule increases its biochemical potency and metabolic stability, enhances cellular permeability and fixes protein-binding issues. Hence, the pharmacokinetic and pharmacodynamic attributes of molecules are also enhanced by many folds. Amrinone and milrinone are the two marketed commercially available vasodilators containing pyridine in their pharmacophore. As per US-FDA, 95 approved pharmaceutical drugs originated from pyridine only.81 There is an overabundance of commercially available drugs in the market, which contain pyridine rings, such as delavirdine for HIV/AIDS, isoniazid and ethionamide for tuberculosis, abiraterone acetate and crizotinib for cancer, ciclopirox for anthelmintic condition, tacrine for Alzheimer's, nifedipine for Raynaud's syndrome, piroxicam for arthritis, nilvadipine for hypertension, roflumilast for COPD,82 pyridostigmine for myasthenia gravis, enpiroline for malaria, nicotinamide for pellagra, nikethamide for respiratory stimulation, tropicamide for antimuscarinic effects, doxylamine for allergy conditions, omeprazole for ulcers, and enisamium iodide for influenza.83
The drug resistance scenario and a better chemical nucleus to improvise the healthcare system to fight against disease conditions encouraged medicinal chemists to design a new molecule, which leads to a library of effective compounds. Recently, several patents for pyridine-based scaffolds to treat various maladies have been filed by many researchers, such as WO2020039097A1 (pyridine, dibenzylpyridine and dicarboxamide derivatives) for treating cancer as PI3K inhibitors (February 2020), US20200062754A1 (Fused amino pyridine) as hsp90 inhibitors(2020), WO2020039060A1 (4-substituted pyrrolo-pyridine) for treating cancer as erbb modulators (2020), WO2020033288A1 as PRMT5 inhibitors (2020), WO2020030925A1 for cancer (2020), US20200040002A1 (tricyclic fused thiophene derivatives) as JAK inhibitors (2020), US20200022983A1 for cancer as topoisomerase inhibitors (2020), CN110698491A (acetamide-pyridine analogues) for cancer (2020), WO2020014465A1 for polymorphic compounds (2020), US20200010458A1 (pyridine derivatives with tetrahydropyranylmethyl groups) abnormal cell (2020), US20200010420A1 (pyridyl inhibitors) for hedgehog signalling (2020), (US20200002310A1 9sulfonyl amide derivatives) for the treatment of cancer and US20190367456A1 (biaryl compositions) for modulating a kinase cascade (2020).84,85 Many bioactive natural products also bear pyridine-based rings, including the coenzymes (i.e., NAD and NADP), vitamins (i.e., niacin and vitamin B6), antibiotics (i.e.,nikkomycin and collismycin), alkaloids (i.e., trigonelline, oxirene, anabasine, huperzine A, paecilomide, and cystine)86 and many other medicinally potent compounds. The figure shown below depicts some naturally occurring and commercially available pyridine-based drug molecules (Fig. 19).
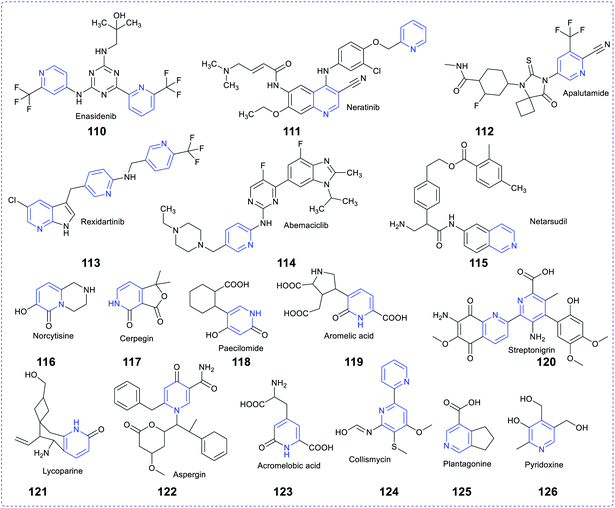 |
| Fig. 19 Commercially available and naturally occurring pyridine-based drug molecules. | |
4. Future direction
The simplest pyridine is a key unit in most of the drugs because of its characteristic chemical properties, such as basicity, solubility, hydrogen bond-forming ability, and able to act as the bioisosteres of amines, amides, heterocyclic rings containing nitrogen atoms. Due to these characteristic properties, pyridine units are incorporated in many drugs and pesticides. The replacement of a portion of drugs with a pyridine unit can improve its characteristic properties and this can be used in prodrug design strategies.
5. Conclusion
The biological activity of a drug depends largely on its physicochemical properties, which can be improved by structural diversity. The scaffold pyridine has been largely used in making clinical candidates owing to its substituent bearing capacity at multiple centers, which has not only been useful in sharpening the potency but also helps in improving pharmacokinetics. From an overall perusal of the literature, it is observed that numerous pyridine analogues were useful against several microbes, but the fatal side effects and drug resistance are the two biggest challenges to the researchers. The overwhelming clinical responses of pyridine derivatives often encourage putting endeavors towards better research. In this review, several forms of pyridine were shown along with their biological properties such as antiviral, anticancer, antimicrobial, anti-diabetic, anti-tubercular, antileishmanial, antichagasic, antioxidant, anticoagulant and antithrombin. In some areas, synthetic strategies were also described. The in-detail explanation of different architectures of pyridine delivered a better understanding of both, the pattern and position of substituents on this skeleton and was deemed to be accountable for its effectiveness.
Conflicts of interest
The authors declare that they have no competing financial interests or personal relationships that could have appeared to influence the work reported in this study.
Acknowledgements
The authors are thankful to the Department of Pharmaceutical Technology, School of Medical Sciences, Adamas University, Vellore Institute of Technology Management, Vellore and Gupta College of Technological Sciences Management, Asansol, for providing resources to write this review.
References
- X. Y. Lou and Y. W. Yang, J. Am. Chem. Soc., 2021, 28, 11976–11981 CrossRef PubMed.
- G. D. Henry, Tetrahedron, 2004, 29, 6043–6061 CrossRef.
- E. D. Anderson and D. L. Boger, J. Am. Chem. Soc., 2011, 133, 12285–12292 CrossRef CAS PubMed.
- I. O. Zhuravel, S. M. Kovalenko, A. V Ivachtchenko, K. V Balakin and V. V Kazmirchuk, Bioorg. Med. Chem. Lett., 2005, 15, 5483–5487 CrossRef CAS PubMed.
- T. Suksrichavalit, S. Prachayasittikul, C. Nantasenamat, C. Isarankura-Na-Ayudhya and V. Prachayasittikul, Eur. J. Med. Chem., 2009, 44, 3259–3265 CrossRef CAS PubMed.
- S. L. Harer and M. S. Bhatia, Chem. Sci. Trans., 2015, 4, 1–16 Search PubMed.
- B. Leal, I. F. Afonso, C. R. Rodrigues, P. A. Abreu, R. Garrett, L. C. S. Pinheiro, A. R. Azevedo, J. C. Borges, P. F. Vegi and C. C. C. Santos, Bioorg. Med. Chem., 2008, 16, 8196–8204 CrossRef CAS PubMed.
- V. K. Zavyalova, A. A. Zubarev and A. M. Shestopalov, Russ. Chem. Bull., 2009, 58, 1939–1944 CrossRef CAS.
- P. C. Sharma and S. Jain, Acta Pol. Pharm., 2008, 65, 551–556 CAS.
- A. M. R. Bernardino, L. C. da Silva Pinheiro, C. R. Rodrigues, N. I. Loureiro, H. C. Castro, A. Lanfredi-Rangel, J. Sabatini-Lopes, J. C. Borges, J. M. Carvalho and G. A. Romeiro, Bioorg. Med. Chem., 2006, 14, 5765–5770 CrossRef CAS PubMed.
- A. M. Attla, H. A. Mansour, A. A. Almehdi and M. M. Abbasi, Nucleosides Nucleotides, 1999, 18, 2301–2306 CrossRef PubMed.
- J.-M. Chezal, J. Paeshuyse, V. Gaumet, D. Canitrot, A. Maisonial, C. Lartigue, A. Gueiffier, E. Moreau, J.-C. Teulade and O. Chavignon, Eur. J. Med. Chem., 2010, 45, 2044–2047 CrossRef CAS PubMed.
- E. Abele, R. Abele and E. Lukevics, Chem. Heterocycl. Compd., 2003, 39, 825–865 CrossRef CAS.
- S. A. M. El-Hawash, A. E. Abdel Wahab and M. A. El-Demellawy, Arch. Pharm., 2006, 339, 14–23 CrossRef CAS PubMed.
- Z. Chen, P. Li, D. Hu, L. Dong, J. Pan, L. Luo, W. Zhang, W. Xue, L. Jin and B. Song, Arabian J. Chem., 2019, 12, 2685–2696 CrossRef CAS.
- A. Hartwich, N. Zdzienicka, D. Schols, G. Andrei, R. Snoeck and I. E. Głowacka, Nucleosides, Nucleotides Nucleic Acids, 2020, 39, 542–591 CrossRef CAS PubMed.
- K. C. Nicolaou, R. Scarpelli, B. Bollbuck, B. Werschkun, M. M. A. Pereira, M. Wartmann, K. H. Altmann, D. Zaharevitz, R. Gussio and P. Giannakakou, Chem. Biol., 2000, 7, 593–599 CrossRef CAS PubMed.
- J.-K. Son, L.-X. Zhao, A. Basnet, P. Thapa, R. Karki, Y. Na, Y. Jahng, T. C. Jeong, B.-S. Jeong and C.-S. Lee, Eur. J. Med. Chem., 2008, 43, 675–682 CrossRef CAS PubMed.
- M. Hayakawa, H. Kaizawa, K. Kawaguchi, N. Ishikawa, T. Koizumi, T. Ohishi, M. Yamano, M. Okada, M. Ohta and S. Tsukamoto, Bioorg. Med. Chem., 2007, 15, 403–412 CrossRef CAS PubMed.
- R. Romagnoli, P. G. Baraldi, M. D. Carrion, O. Cruz-Lopez, C. L. Cara, M. Tolomeo, S. Grimaudo, A. Di Cristina, M. R. Pipitone and J. Balzarini, Bioorg. Med. Chem. Lett., 2008, 18, 5041–5045 CrossRef CAS PubMed.
- J.-P. Liou, K.-S. Hsu, C.-C. Kuo, C.-Y. Chang and J.-Y. Chang, J. Pharmacol. Exp. Ther., 2007, 323, 398–405 CrossRef CAS PubMed.
- P. Nagababu, D. A. Kumar, K. L. Reddy, K. A. Kumar, M. B. Mustafa, M. Shilpa and S. Satyanarayana, Met.-Based Drugs, 2008, 2008, 1–8 CrossRef PubMed.
- M. El-Naggar, H. Almahli, H. S. Ibrahim, W. M. Eldehna and H. A. Abdel-Aziz, Molecules, 2018, 23, 1459 CrossRef PubMed.
- P. V. S. Ramya, L. Guntuku, S. Angapelly, C. S. Digwal, U. J. Lakshmi, D. K. Sigalapalli, B. N. Babu, V. G. M. Naidu and A. Kamal, Eur. J. Med. Chem., 2018, 143, 216–231 CrossRef CAS PubMed.
- H. S. Khalaf, H. E. M. Tolan, M. N. El-Bayaa, M. A. A. Radwan, M. El-Manawaty and W. A. El-Sayed, Russ. J. Gen. Chem., 2020, 90, 1706–1715 CrossRef CAS.
- P. Thongaram, S. Borwornpinyo, P. Kanjanasirirat, K. Jearawuttanakul, M. Kongsema, N. Chuanopparat and P. Ngernmeesri, Tetrahedron, 2020, 76, 131473 CrossRef CAS.
- E. Mansour, A. A. Abd-Rabou, I. F. Nassar and S. I. Elewa, Polycyclic Aromat. Compd., 2021, 1–22 Search PubMed.
- C. S. Rani, A. G. Reddy, E. Susithra, K.-K. Mak, M. R. Pichika, S. Reddymasu and M. V. B. Rao, Med. Chem. Res., 2021, 30, 74–83 CrossRef CAS.
- V. Barresi, D. F. Condorelli, C. G. Fortuna, G. Musumarra and S. Scirè, Bioorg. Med. Chem., 2002, 10, 2899–2904 CrossRef CAS PubMed.
- M. B. Gurdere, B. Yilmaz and Y. Budak, Synth. Commun., 2014, 44, 981–986 CrossRef CAS.
- M. T. Cocco, C. Congiu, V. Lilliu and V. Onnis, Bioorg. Med. Chem., 2007, 15, 1859–1867 CrossRef CAS PubMed.
- V. Onnis, M. T. Cocco, V. Lilliu and C. Congiu, Bioorg. Med. Chem., 2008, 16, 2367–2378 CrossRef CAS PubMed.
- B. L. Furman, Curr. Protoc., 2021, 1, e78 CAS.
- R. H. Bahekar, M. R. Jain, P. A. Jadav, V. M. Prajapati, D. N. Patel, A. A. Gupta, A. Sharma, R. Tom, D. Bandyopadhya and H. Modi, Bioorg. Med. Chem., 2007, 15, 6782–6795 CrossRef CAS PubMed.
- B. Y. Kim, J. B. Ahn, H. W. Lee, S. K. Kang, J. H. Lee, J. S. Shin, S. K. Ahn, C. Il Hong and S. S. Yoon, Eur. J. Med. Chem., 2004, 39, 433–447 CrossRef CAS PubMed.
- L. Suresh, P. S. V. Kumar, P. Onkar, L. Srinivas, Y. Pydisetty and G. V. P. Chandramouli, Res. Chem. Intermed., 2017, 43, 5433–5451 CrossRef CAS.
- M. Adib, F. Peytam, M. Rahmanian-Jazi, M. Mohammadi-Khanaposhtani, S. Mahernia, H. R. Bijanzadeh, M. Jahani, S. Imanparast, M. A. Faramarzi and M. Mahdavi, New J. Chem., 2018, 42, 17268–17278 RSC.
- F. Ma, J. Liu, T. Zhou, M. Lei, J. Chen, X. Wang, Y. Zhang, X. Shen and L. Hu, Eur. J. Med. Chem., 2018, 152, 307–317 CrossRef CAS PubMed.
- A. Ozdemir, G. Turan-Zitouni, Z. A. Kaplancıklı, G. Işcan, S. Khan and F. Demirci, Eur. J. Med. Chem., 2010, 45, 2080–2084 CrossRef PubMed.
- E. R. Shakurova, D. A. Pozdnyakova, E. V. Tretyakova and L. V. Parfenova, Lett. Drug Des. Discovery, 2020, 17, 79–84 CrossRef CAS.
- A. Altundas, S. Ayvaz and E. Logoglu, Med. Chem. Res., 2011, 20, 1–8 CrossRef CAS.
- S. M. Sondhi, M. Dinodia and A. Kumar, Bioorg. Med. Chem., 2006, 14, 4657–4663 CrossRef CAS PubMed.
- B. N. Acharya, D. Thavaselvam and M. P. Kaushik, Med. Chem. Res., 2008, 17, 487–494 CrossRef.
- M. Shekarchi, M. Pirali-Hamedani, L. Navidpour, N. Adib and A. Shafiee, J. Iran. Chem. Soc., 2008, 5, 150–158 CrossRef CAS.
- E. Kita and R. Lisiak, Transition Met. Chem., 2010, 35, 441–450 CrossRef CAS.
- N. Bharti, M. R. Maurya, F. Naqvi and A. Azam, Bioorg. Med. Chem. Lett., 2000, 10, 2243–2245 CrossRef CAS PubMed.
- M. R. Maurya, S. Agarwal, M. Abid, A. Azam, C. Bader, M. Ebel and D. Rehder, Dalton Trans., 2006, 937–947 RSC.
- M. V Pavlova, A. I. Mikhalev, M. E. Kon’shin, M. Y. Vasil’eva, L. G. Mardanova, T. F. Odegova and M. I. Vakhrin, Pharm. Chem. J., 2001, 35, 664–666 CrossRef.
- M. G. Mamolo, D. Zampieri, V. Falagiani, L. Vio and E. Banfi, Farm, 2001, 56, 593–599 CrossRef CAS.
- M. G. Mamolo, V. Falagiani, D. Zampieri, L. Vio and E. Banfi, Farm, 2001, 56, 587–592 CrossRef CAS.
- G. Navarrete-Vazquez, G. Marı, Z. V. Duarte-Fajardo, J. Vargas-Villarreal, S. Estrada-Soto, F. González-Salazar, E. Hernández-Núñez and S. Said-Fernández, Bioorg. Med. Chem., 2007, 15, 5502–5508 CrossRef CAS PubMed.
- F. E. Goda, A.-M. Alaa and O. A. Attef, Bioorg. Med. Chem., 2004, 12, 1845–1852 CrossRef CAS PubMed.
- I. O. Zhuravel, S. M. Kovalenko, A. V. Ivachtchenko, K. V. Balakin and V. V. Kazmirchuk, Bioorg. Med. Chem. Lett., 2005, 15, 5483–5487 CrossRef CAS PubMed.
- S. L. Gaonkar, K. M. L. Rai and B. Prabhuswamy, Eur. J. Med. Chem., 2006, 41, 841–846 CrossRef CAS PubMed.
- M. S. Yar, M. A. ALIa, D. Sriram and P. Yogeeswari, Acta Pol. Pharm., 2015, 63, 491–496 Search PubMed.
- S. L. Gaonkar, K. M. L. Rai and B. Prabhuswamy, Med. Chem. Res., 2007, 15, 407–417 CrossRef CAS.
- P. Mishra, T. Lukose and S. K. Kashaw, Indian J. Pharm. Sci., 2007, 69, 665 CrossRef CAS.
- S. Mehta, N. Swarnkar, R. Vyas, J. Vardia, P. B. Punjabi and S. C. Ameta, Phosphorus, Sulfur Silicon Relat. Elem., 2007, 183, 105–114 CrossRef.
- V. Aanandhi, S. George and V. Vaidhyalingam, Arkivoc, 2008, 11, 187–194 Search PubMed.
- A. Riahi, M. Wurster, M. Lalk, U. Lindequist and P. Langer, Bioorg. Med. Chem., 2009, 17, 4323–4326 CrossRef CAS PubMed.
- J. J. Vora, S. B. Vasava, K. C. Parmar, S. K. Chauhan and S. S. Sharma, E-J. Chem., 2009, 6, 1205–1210 CrossRef CAS.
- D. Sriram, P. Yogeeswari and K. Madhu, Bioorg. Med. Chem. Lett., 2006, 16, 876–878 CrossRef CAS PubMed.
- P. Herzigova, V. Klimesova, K. Palat, J. Kaustova, H. Dahse and U. Mollmann, Arch. Pharm., 2009, 342, 394–404 CrossRef CAS PubMed.
- L. R. S. Dias, M. B. Santos, S. de Albuquerque, H. C. Castro, A. M. T. de Souza, A. C. C. Freitas, M. A. V DiVaio, L. M. Cabral and C. R. Rodrigues, Bioorg. Med. Chem., 2007, 15, 211–219 CrossRef CAS PubMed.
- F. Shi, C. Li, M. Xia, K. Miao, Y. Zhao, S. Tu, W. Zheng, G. Zhang and N. Ma, Bioorg. Med. Chem. Lett., 2009, 19, 5565–5568 CrossRef CAS PubMed.
- A. Worachartcheewan, S. Prachayasittikul, R. Pingaew, C. Nantasenamat, T. Tantimongcolwat, S. Ruchirawat and V. Prachayasittikul, Med. Chem. Res., 2012, 21, 3514–3522 CrossRef CAS.
- C. M. Timperley, M. Bird, S. C. Heard, S. Notman, R. W. Read, J. E. H. Tattersall and S. R. Turner, J. Fluorine Chem., 2005, 126, 1160–1165 CrossRef CAS.
- N. Bharti, M. R. Maurya, F. Naqvi and A. Azam, Bioorg. Med. Chem. Lett., 2000, 10, 2243–2245 CrossRef CAS PubMed.
- J. T. E. Lim, G. A. Piazza, E. K. H. Han, T. M. Delohery, H. Li, T. S. Finn, R. Buttyan, H. Yamamoto, G. J. Sperl and K. Brendel, Biochem. Pharmacol., 1999, 58, 1097–1107 CrossRef CAS PubMed.
- B. M. Zeglis, V. Divilov and J. S. Lewis, J. Med. Chem., 2011, 54, 2391–2398 CrossRef CAS PubMed.
- T. B. Chaston and D. R. Richardson, JBIC, J. Biol. Inorg. Chem., 2003, 8, 427–438 CrossRef CAS PubMed.
- A. Deep, R. K. Bhatia, R. Kaur, S. Kumar, U. J. Kumar, H. Singh, S. Batra, D. Kaushik and P. D. Kishore, Curr. Top. Med. Chem., 2017, 17, 238–250 CrossRef CAS PubMed.
- S. Şenkardes, A. Ture, S. Ekrek, A. T. Durak, M. Abbak, O. Çevik, B. Kaskatepe, I. Kuçukguzel and S. G. Kuçukguzel, J. Mol. Struct., 2021, 1223, 128962 CrossRef.
- G. Sadawarte, S. Jagatap, M. Patil, V. Jagrut and J. D. Rajput, Eur. J. Chem., 2021, 12, 279–283 CrossRef CAS.
- L. Wei, W. Tan, J. Zhang, Y. Mi, F. Dong, Q. Li and Z. Guo, Polymers, 2019, 11, 371 CrossRef PubMed.
- M. A. Abdelgawad, R. B. Bakr and A. A. Azouz, Bioorg. Chem., 2018, 77, 339–348 CrossRef CAS PubMed.
- H. A. El-Sayed, A. H. Moustafa, A. E. El-Torky and A. El-Salam, Russ. J. Gen. Chem., 2017, 87, 2401–2408 CrossRef CAS.
- H. M. Patel, M. N. Noolvi, N. S. Sethi, A. K. Gadad and S. S. Cameotra, Arabian J. Chem., 2017, 10, S996–S1002 CrossRef CAS.
- K. S. Chaudhari, H. M. Patel and S. J. Surana, Indian J. Tuberc., 2017, 64, 119–128 CrossRef PubMed.
- H. Patel, K. Chaudhari, P. Jain and S. Surana, Bioorg. Chem., 2020, 102, 104099 CrossRef CAS PubMed.
- S. R. Alizadeh and M. A. Ebrahimzadeh, Mini-Rev. Med. Chem., 2021, 21, 2584–2611 CrossRef CAS PubMed.
- R. Sahu, R. Mishra R, R. Kumar, A. Mazumder and A. Kumar, Indian J. Pharm. Sci., 2021, 83, 162–185 CAS.
- H. Dai, M. Huang and J. Qian, et al., Eur. J. Med. Chem., 2019, 166, 470–479 CrossRef CAS PubMed.
- Z. Cournia, A. Efstratiadis, A. Kapella, E. Couladouros and S. Christoforidis, WO2020039097A1, 2020.
- A. Z. Abdelazem, M. M. Al-Sanea, M. H. Park and S. H. Lee, Bioorg. Med. Chem. Lett., 2016, 26, 1301–1304 CrossRef CAS PubMed.
- Y. Ling, Z. Y. Hao, D. Liang, C. L. Zhang, Y. F. Liu and Y. Wang, Drug Des., Dev. Ther., 2021, 15, 4289 CrossRef CAS PubMed.
|
This journal is © The Royal Society of Chemistry 2022 |