DOI:
10.1039/D2RA01208A
(Paper)
RSC Adv., 2022,
12, 15098-15104
Monoammonium salts of multiprotic acids as dopants for proton-conductive hydrogel membranes: the effects of anions
Received
23rd February 2022
, Accepted 11th May 2022
First published on 18th May 2022
Abstract
Monoammonium salts of diprotic acid (NH4HSO4) and triprotic acid (NH4H2PO4), whose cations and anions are both potential proton carriers, were employed as dopants for proton-conductive hydrogel membranes to explore the effects of anions on thermal, mechanical, and electrochemical performance. Robust hydrogel membranes were obtained by radical copolymerization of acrylamide and ethylene glycol dimethacrylate dissolved in aqueous solutions of NH4HSO4 and NH4H2PO4. By virtue of the protonated ammonium cation, the ionic conductivities of the hydrogels doped with NH4HSO4 and NH4H2PO4 were superior to those doped with the corresponding inorganic acids (H2SO4 and H3PO4). The hydrogel doped with NH4HSO4 exhibited a higher ionic conductivity but lower mechanical strength and thermostability than that with NH4H2PO4. Ionic conduction in the doped hydrogels was dominated by the vehicle mechanism and NH4HSO4 resulted in lower activation energy for the conduction than NH4H2PO4. In addition, the fuel cell performances of the hydrogel membranes at room temperature were evaluated.
1. Introduction
Hydrogels, which are a three-dimensional network of hydrophilic polymers absorbing large quantities of aqueous medium, have been used as biosensors, drug delivery vectors and separating materials by virtue of the biocompatibility and water-dependent viscoelastic behavior.1,2 By tuning the polymeric networks or the aqueous medium, ion-conductive hydrogels can be constructed for electrochemical applications such as energy storage and conversion devices.3–6 Introduction of acid moieties to the polymeric networks rendered hydrogels with substantial proton conductivities. For example, Suzuki et al. prepared acid hydrogel films based on partially phosphorylated poly(vinyl alcohol)s crosslinked by glutaraldehyde and achieved a proton conductivity of 40 mS cm−1 at 40 °C.7 Zygadło-Monikowska et al. obtained hydrogels by copolymerization of 2-acrylamido-2-methyl-propanesulfonic acid (AMPSA) and N,N′-methylene-bis-acrylamide (MBA) dissolved in water, which exhibited high conductivities but weak mechanical performance.8 Because the acid-modified polymers were inherently rigid, few acid polymer networks satisfied the requirements of forming flexible hydrogels.9,10
Adding inorganic acid dopants such as H2SO4 and H3PO4 to aqueous medium was an alternative strategy to obtain proton-conductive hydrogels. Radical polymerization of acrylamide (AM) as monomer and MBA as cross-linking agent in aqueous H2SO4 or H3PO4 solution gave acid hydrogels whose proton conductivities reached up to 10 mS cm−1.11,12 We prepared H2SO4-doped polyacrylamide (PAM) hydrogels using divinylbenzene (DVB) as cross-linking agent and promoted the conductivity to 187 mS cm−1 at 30 °C.13 Modestino et al. fabricated ultra-thin proton-conductive hydrogel films (thickness, <100 nm) for electrocatalyst interfaces by submerging thermally crosslinked poly(acrylic acid)–poly(vinyl alcohol) into H2SO4 solution.14 Despite the simplicity, dopants other than inorganic acids have hardly been employed to prepare proton-conductive hydrogels. Ammonium slats, which were regarded as good proton donors, have ever been utilized to dope solid poly(vinyl alcohol) and poly(ethylene oxide) for proton conduction.15–17 Owing to the exchangeable protons available in both the cation and anions, monoammonium salts of multiprotic acids are expected to be efficient dopants for promoting conductivity of hydrogels.
The aim of present study is to evaluate the potential use of monoammonium salts of multiprotic acids as dopants to construct proton-conductive hydrogel membranes for fuel cell applications, considering the effects of acid anions. Aqueous solutions of ammonium hydrogen sulfate (NH4HSO4) and dihydrogen phosphate (NH4H2PO4) were converted into PAM hydrogel membranes by radical polymerization. Ethylene glycol dimethacrylate (EGDMA) instead of MBA or DVB was employed as cross-linking agent because it had stronger gelation ability in the salt solutions and favored the structural uniformity of PAM hydrogels.18 Impact of the acid anions on mechanical performance of the obtained hydrogels were determined by rheological, tensile, and compressive tests. Morphology of the PAM networks were observed using a scanning electron microscope (SEM) and ionic conductivities of the doped hydrogels were characterized by electrochemical impendence spectroscopy (EIS) measurements. Moreover, fuel cells were fabricated from the hydrogel membranes doped with NH4HSO4 and NH4H2PO4 to evaluate the electrochemical performance under practical working conditions.
2. Experimental
2.1. Materials
AM, NH4HSO4 and NH4H2PO4 were supplied from Greagent. EGDMA and 1-hydroxycyclohexylphenylketone (HCHPK) were obtained from Acros. Gas diffusion electrodes loaded with catalyst 0.5 mg Pt cm−2 were purchased from Suzhou YiLongSheng (thickness, 285 μm; pressure drop, >2.5 mm H2O). Deionized water was used in all experiments.
2.2. Preparation of the hydrogel membranes
Hydrogel composition in this work has been optimized to balance ionic conductivities and mechanical strengths. AM (4.2 mol L−1), EGDMA (0.05 mol L−1), HCHPK (0.005 mol L−1), ammonium salt (1.1 mol L−1) and water were mixed thoroughly in a sealed glass bottle by ultrasonic vibration at room temperature for 1 h to obtain a precursor. The precursor was then poured into a mold and exposed to a 365 nm UV irradiation at an intensity of 6 mW cm−2. After 30 min, a transparent hydrogel membrane was formed and demolded for succeeding characterizations. HG-AHSO4 and HG-AH2PO4 denoted the hydrogels doped with NH4HSO4 and NH4H2PO4, respectively. The monoammonium salts were the only dopants loaded in the hydrogels. The synthetic route and photographs of the resultant hydrogel membranes are shown in Fig. 1.
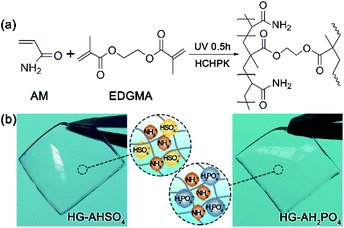 |
| Fig. 1 (a) Synthetic route of the polymer network and (b) photographs of HG-AHSO4 and HG-AH2PO4 membranes. | |
3. Characterization
3.1. Hydrogel structures
Fourier transform infrared (FT-IR) spectra were measured by a PerkinElmer Spectrum Two Li10014 spectrometer in the range of 400–4000 cm−1. HG-AHSO4 and HG-AH2PO4 were desalted in deionized water and then freeze-dried in Sientz 10 W vacuum freeze dryer to obtain XG-AHSO4 and XG-AH2PO4 xerogels. SEM observations of the xerogels were performed under a FEI Quanta 250 SEM running under 5 kV.
3.2. Thermal and mechanical parameters
Thermogravimetric (TG) analyses were conducted on a METTLER TOLEDO thermo gravimetric analyzer at a heating rate of 10 °C min−1 under an air atmosphere. Dynamic rheological analyses were performed on an Anton Paar MCR302 rheometer at 25 °C. The frequency sweep curves of cylindrical specimens (ø25 × 2 mm) were measured in a linear viscoelastic region (frequency range, 0.01–10 Hz; strain, 1%). Tensile and compressive measurements were carried out at 25 °C using an Instron 5967 electronic universal tester. The tensile tests were conducted using the dumbbell-shaped specimens (cross section: 4 × 5 mm) at a displacement rate of 20 mm min−1. The compressive tests were performed using cylindrical specimens (ø14 × 17 mm) at a displacement rate of 2 mm min−1.
3.3. Electrochemical parameters
Acidities (pH values) were measured by a York PHS-3C acidometer with a E-201-9 glass electrode. EIS measurements were conducted on an EG&G Princeton Application Research Versa STAT 3 workstation connecting with the PC running the electrochemical impedance software (frequency range: 1 MHz to 100 Hz; AC amplitude: 10 mV). Samples were sandwiched between two pieces of indium tin oxide (ITO) glass slides to collect impedance responses. The sample temperature was controlled by a hot-stage (WT-3000). The bulk resistance Rb (Ω) was obtained by fitting the impedance response with ZSimpWin software. Ionic conductivity σ (S cm−1) was then calculated based on the space between the electrodes (d, cm) and the contact area between sample and the electrodes (A, cm2). |
 | (1) |
3.4. Fuel cell assembly and performance
Owing to self-adhesiveness of the hydrogels, a membrane electrode assembly (MEA) was effortlessly constructed by sticking the Pt-loaded diffusion electrodes to both surfaces of a hydrogel membrane as shown in Fig. 2. The MEA was then clamped between a pair of graphite plates engraved with serpentine gas flow channels to obtain a single fuel cell with an active area of 1 cm2. Humidified H2 as fuel and O2 as oxidant were continuously fed to the anode and cathode at rates of 160 and 80 mL min−1, respectively. The unreacted fuel and oxidant gases were discharged without back pressure. Polarization curves of the fuel cells were collected at room temperature using a programmable DC electronic load (IT8512, ITECH).
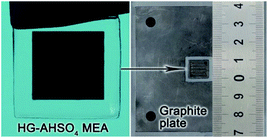 |
| Fig. 2 Photographs of HG-AHSO4 MEA and graphite plate of fuel cell. | |
4. Results and discussion
4.1. Architectures of the doped hydrogels
FT-IR spectra suggested that copolymerization of AM and EGDMA was successfully. As shown in Fig. 3, the bands at 1613 and 988 cm−1 in the spectra of HG-AHSO4 and HG-AH2PO4 precursors were ascribed to the stretching and bending vibrations of the C
C bond, respectively. The bands disappeared in the spectra of HG-AHSO4 and HG-AH2PO4 owing to the polymerization. The band at 1204 cm−1 in the spectrum of NH4HSO4 solution arose from the stretching vibration of the S
O bond, shifting to 1183 cm−1 in the spectrum of HG-AHSO4 (Fig. 3a). The fact indicated that the S
O bond of the HSO4− anion as acceptor formed hydrogen bond with the N–H bond of amide.19 Hydrogen bond between the H2PO4− anion and amide was also evidenced by the band shift of the stretching vibration of the P
O bond from 1157 cm−1 in the spectrum of NH4H2PO4 solution to 1116 cm−1 in that of HG-AH2PO4 (Fig. 3b).20
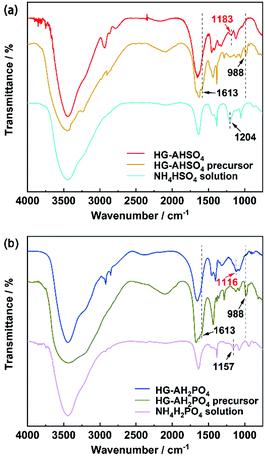 |
| Fig. 3 FT-IR spectra of (a) HG-AHSO4, HG-AHSO4 precursor, NH4HSO4 solution and (b) HG-AH2PO4, HG-AH2PO4 precursor, NH4H2PO4 solution. | |
SEM images of XG-AHSO4 and XG-AH2PO4 revealed morphologies of PAM networks in the hydrogels doped with the ammonium salts. As shown in Fig. 4, both XG-AHSO4 and XG-AH2PO4 exhibited a porous architecture, suggesting that EGDMA participated in the cross-linking efficiently. However, the pore size and wall thickness of XG-AH2PO4 were much larger than those of XG-AHSO4. The difference in the morphology was supposed to arise from the different solubility of AM in the precursors. At 25 °C, the maximum dissolved amounts of AM in HG-AHSO4 and HG-AH2PO4 precursors were measured to be 1845 and 933 g L−1, respectively. During the polymerization, AM in HG-AH2PO4 precursor tended to precipitate so that denser walls formed as observed in PAM hydrogels prepared at low temperatures.21 The morphology of PAM network had impacts on mechanical and electrochemical performance of the doped hydrogels as discussed later.
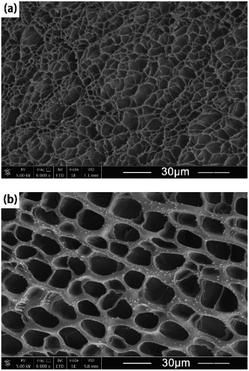 |
| Fig. 4 Cross sectional SEM images of (a) XG-AHSO4 and (b) XG-AH2PO4. | |
4.2. Effect of anion on the mechanical strengths of the hydrogels
Viscoelastic behavior of HG-AHSO4 and HG-AH2PO4 was characterized with dynamic mechanical analysis and the resultant frequency dependent storage (G′) and loss (G′′) moduli are shown in Fig. 5. HG-AHSO4 and HG-AH2PO4 exhibited essentially identical viscoelastic behavior over the measured frequency range. G′ was always higher than G′′ because the elastic deformation of the polymer network overwhelmed the plastic one. G′ of HG-AHSO4 was almost identical to that of HG-AH2PO4 while G′′ of HG-AHSO4 was slightly higher than that of HG-AH2PO4. The enhanced G′′ of HG-AHSO4 originated likely from the smaller pore size of the polymer network, which had greater hindrance to the shear deformation.22 The moduli of HG-AHSO4 and HG-AH2PO4 showed a weak frequency dependence and the both loss factors (tan
δ) kept approximately at 0.1 over the measured frequency range. The elasticity-dominated behavior of the hydrogels doped with the ammonium salts favored the application in fuel cells.
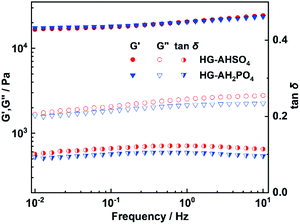 |
| Fig. 5 Changes of G′, G′′, and tan δ as a function of frequency for HG-AHSO4 and HG-AH2PO4 at 25 °C. | |
Mechanical strengths of HG-AHSO4 and HG-AH2PO4 were determined by tensile and compressive measurements. As shown in Fig. 6a, HG-AH2PO4 broke at a tensile strain of 405% with a stress of 87 kPa while HG-AHSO4 broke at a lower tensile strain (333%) with a reduced stress (52 kPa). The thicker walls of the network endowed HG-AH2PO4 with a higher strength and the larger pores of the network allowed HG-AH2PO4 to deform at a higher magnitude. For the same reason, the compressive stress of HG-AH2PO4 was higher at the same strain (Fig. 6b). At a compressive strain of 90%, the compressive stresses of HG-AHSO4 and HG-AH2PO4 reached 1.95 and 2.38 MPa, respectively. The robust mechanical strengths of the hydrogels doped with the ammonium salts assured stable geometric shape for the fuel cell application.
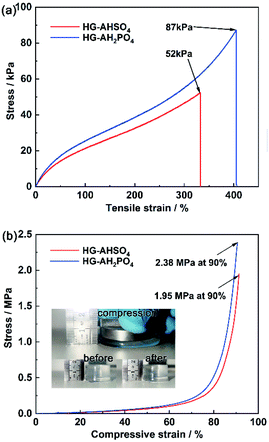 |
| Fig. 6 (a) Tensile and (b) compressive stress–strain curves of HG-AHSO4 and HG-AH2PO4 (insets are photographs of HG-AH2PO4 before and after compression). | |
4.3. Effect of anion on the thermal stability of the hydrogels
TG curves of HG-AHSO4 and HG-AH2PO4 are shown in Fig. 7. The initial weight loss at temperatures below 200 °C was attributed to the dehydration. At higher temperatures, a two-step decomposition process was observed for the both hydrogels. For HG-AHSO4, the first step decomposition began at 235 °C, arising from the breakdown of NH4HSO4.23 In the case of HG-AH2PO4, the first step decomposition at 273 °C was ascribed to the breakdown of NH4H2PO4.24 The second step decompositions of HG-AHSO4 and HG-AH2PO4 began at 364 °C and 429 °C, respectively. The weight loss in the second step was attributed to the degradation of the polymer network. Like PAM hydrogels derived from H2SO4 solution with different concentrations, PAM of HG-AHSO4 exhibited a lower degradation temperature than that of HG-AH2PO4 because of lower pH level.13 After the two-step decomposition, the residues of the hydrogels remained approximately 10 wt%.
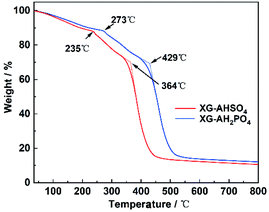 |
| Fig. 7 TG curves of HG-AHSO4 and HG-AH2PO4 obtained under an air atmosphere. | |
4.4. Effect of anion on the ionic conductivities
Conductivities of HG-AHSO4, HG-AH2PO4 and the aqueous solutions of NH4HSO4 (1.1 mol L−1) and NH4H2PO4 (1.1 mol L−1) were obtained from EIS measurements. As shown in Fig. 8a, a typical impedance of the ammonium salt electrolytes consisted of an inclined line. An equivalent circuit containing two constant phase elements (CPE1 and CPE2) and the bulk resistance Rb was fit well to the impedance (inset of Fig. 8a). The ionic conductivities based on the derived Rb as functions of temperature are shown in Fig. 8b. The aqueous solution of NH4HSO4 exhibited a higher conductivity than that of NH4H2PO4 at the same temperature owing to the higher H+ concentration. Because the dissociation constant of the HSO4− anion (1.2 × 10−2) was much higher than that of the H2PO4− anion (6.2 × 10−8),25 NH4HSO4 led to a lower pH level (0.75) than NH4H2PO4 (3.96) after dissolved in water. At 30 °C, the aqueous solutions of NH4HSO4 and NH4H2PO4 exhibited ionic conductivities of 360 and 104 mS cm−1, respectively. Since the molar concentration of the two ammonium salts was identical, the higher ionic conductivity of the NH4HSO4 solution should be ascribed to more hydronium ions resulted from the anion dissociation.
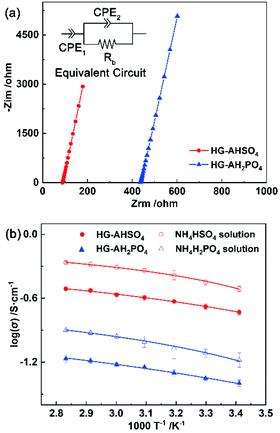 |
| Fig. 8 (a) EIS spectra of HG-AHSO4 and HG-AH2PO4 at 30 °C and (b) temperature dependence of conductivities for HG-AHSO4, HG-AH2PO4 and the aqueous solutions of NH4HSO4 and NH4H2PO4 (solid lines are fitting curves). | |
Ionic conductivity of HG-AHSO4 was also always higher than that of NH4H2PO4 over the measured temperature range. Because partial acid anions formed hydrogen bonds with the amide group of PAM, pH values of HG-AHSO4 and HG-AH2PO4 increased to 1.35 and 4.41, respectively. At 30 °C, ionic conductivity of HG-AHSO4 reached 225 mS cm−1, which was higher than that of the 3.5 mol L−1 H2SO4-doped PAM hydrogel (184 mS cm−1).13 Meanwhile, ionic conductivity of HG-AH2PO4 (46 mS cm−1) was also superior to the 14.3 mol L−1 (85 wt%) H3PO4-doped PAM hydrogel (10 mS cm−1).11 The higher conductivities of the hydrogels doped with the monoammonium salts were attributed to the protonated ammonium cation, which can enhance proton conduction via both forming hydrogen bond and acting as proton carrier.26 At 80 °C, ionic conductivities of HG-AHSO4 and HG-AH2PO4 climbed to 309 and 65 mS cm−1, respectively.
4.5. Activation energies for the ionic conduction
The temperature-dependent conductivities of the doped hydrogels were fit well to the Vogel–Tamman–Fulcher (VTF) equation.27 |
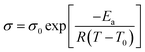 | (2) |
Where σ0, Ea, R, and T0 are the pre-factor (S cm−1), activation energy (kJ mol−1), ideal gas constant (8.314 J mol−1 K−1) and the Vogel temperature (K), respectively. According to the VTF behavior, it was speculated that the vehicle mechanism the ionic conduction in the hydrogels doped with the ammonium salts.27 In this case, hydronium ions, the ammonium cation, and the acidic anions migrated to account for the observed conductivities.28 The derived Ea values for the ionic conduction of HG-AHSO4 and HG-AH2PO4 were 0.440 and 0.748 kJ mol−1, respectively. In comparison with corresponding ammonium salt solution, Ea of the hydrogel approximately doubled owing probably to the hindrance of the polymer network to the conduction. The higher Ea of HG-AH2PO4 may be ascribed to larger volume of the H2PO4− anion, which was harder to diffuse in the hydrogels. The parameters resulted from the VTF fitting are listed in Table 1.
Table 1 Parameters resulted from the fitting to the VTF equation
Electrolytes |
Ea/kJ mol−1 |
σ0/mS cm−1 |
R2 |
NH4HSO4 solution |
0.216 |
360 |
0.998 |
NH4H2PO4 solution |
0.315 |
104 |
0.997 |
HG-AHSO4 |
0.440 |
225 |
0.996 |
HG-AH2PO4 |
0.748 |
46 |
0.995 |
4.6. Fuel cell performance
HG-AHSO4 and HG-AH2PO4 membranes with a thickness of 200 μm were assembled into fuel cells to evaluate the electrochemical performance under practical working conditions. The resultant polarization curves at 25 °C are shown in Fig. 9. The open-circuit voltages (OCV) of the fuel cells fabricated from HG-AHSO4 and HG-AH2PO4 were 1.02 and 0.96 V, respectively. The higher OCV resulted from HG-AHSO4 indicated that the smaller pores of the polymer network prevented hydrogen and oxygen from penetrating the membrane more efficiently. The short-circuit current (ISC) of the fuel cell fabricated from HG-AHSO4 was much higher than that from HG-AH2PO4, in accordance with the results of the EIS characterization. A peak power density (Pmax) of 106 mW cm−2 was achieved from HG-AHSO4, which overtook Pmax obtained from the H2SO4-doped hydrogel with the same membrane thickness (74 mW cm−2).13 The result confirmed the advantage of ammonium salts over corresponding inorganic acids for proton conduction. A lower Pmax (64 mW cm−2) was obtained from HG-AH2PO4 due to the declined proton conductivity. The measured VOC, ISC and Pmax for the fuel cells fabricated from HG-AHSO4 and HG-AH2PO4 are summarized in Table 2.
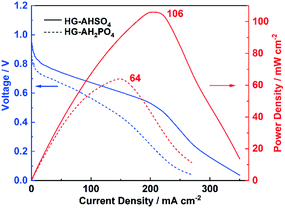 |
| Fig. 9 Polarization curves of the H2/O2 fuel cells fabricated from HG-AHSO4 and HG-AH2PO4 at 25 °C. | |
Table 2 Performance of the fuel cells fabricated from HG-AHSO4 and HG-AH2PO4 at 25 °C
Electrolytes |
OCV/V |
ISC/mA cm−2 |
Peak power density/mW cm−2 |
HG-AHSO4 |
1.027 ± 0.011 |
350 ± 10 |
106 ± 3 |
HG-AH2PO4 |
0.960 ± 0.009 |
159 ± 8 |
64 ± 2 |
Performance stability of the HG-AHSO4 and HG-AH2PO4 fuel cells was evaluated by recording OCV at 25 °C with a certain interval (24 h).29 As shown in Fig. 10, OCV of the fuel cell fabricated from HG-AHSO4 kept relatively stable and maintained above 90% of its initial value for 1272 h. For HG-AH2PO4, OCV of the fuel cell decreased to 90% of its initial value in 744 h. The faster OCV attenuation of the fuel cell fabricated from HG-AH2PO4 was assumed to be related to the larger pores of the polymer network, which allowed easier penetration of fuel gas. The stability measurements suggested that the hydrogels doped with the ammonium salts were durable for long-term operation of the fuel cells.
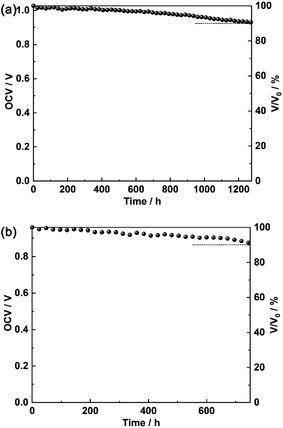 |
| Fig. 10 Time-dependent OCVs of the fuels cells fabricated from (a) HG-AHSO4 and (b) HG-AH2PO4. | |
5. Conclusions
The potential application of monoammonium salts of multiprotic acids as dopants to construct proton-conductive hydrogel membranes for fuel cell was demonstrated. Also, the effects of acid anions on thermal, mechanical, and electrochemical performance of the hydrogel membranes were evaluated. Robust hydrogel membranes were obtained by copolymerization of AM and EGDMA in aqueous solutions of NH4HSO4 and NH4H2PO4. Conductivity of the hydrogel doped with NH4HSO4 was superior to that with NH4H2PO4. Owing to the contribution of the protonated ammonium cation, the hydrogels doped with the ammonium salts exhibited higher conductivities than those doped with the corresponding inorganic acids. Performance tests of the fuel cells fabricated from the hydrogel membranes doped with NH4HSO4 and NH4H2PO4 showed that the monoammonium salts of multiprotic acids can be used as suitable dopants to enhance proton conductivity of flexible electrolytes for electrochemical devices.
Conflicts of interest
The authors declare no competing financial interest.
Acknowledgements
This research did not receive any specific grant from funding agencies in the public, commercial, or not-for-profit sectors.
Notes and references
- D. Y. Zhai, B. Liu, Y. Shi, L. J. Pan, Y. Q. Wang, W. B. Li, R. Zhang and G. H. Yu, ACS Nano, 2013, 7, 3540–3546 CrossRef CAS PubMed.
- Q. Y. Chai, Y. Jiao and X. J. Yu, Gels, 2017, 3, 6 CrossRef PubMed.
- Y. Zhao, B. Liu, L. J. Pan and G. H. Yu, Energy Environ. Sci., 2013, 6, 2856 RSC.
- Z. F. Wang, H. F. Li, Z. J. Tang, Z. X. Liu, Z. H. Ruan, L. T. Ma, Q. Yang, D. H. Wang and C. Y. Zhi, Adv. Funct. Mater., 2018, 28(48), 1804560 CrossRef.
- M. K. Yazdi, V. Vatanpour, A. Taghizadeha, M. Taghizadeh, M. R. Ganjali, M. T. Munir, S. Habibzadeh, M. R. Saeb and M. Ghaedi, Mater. Sci. Eng., C, 2020, 114, 111023 CrossRef CAS PubMed.
- C. Y. Chan, Z. Wang, H. Jia, P. F. Ng, L. Chow and B. Fei, J. Mater. Chem. A, 2021, 9, 2043–2069 RSC.
- M. Suzuki, T. Yoshida, S. Kobayashi, T. Koyama, M. Kimura, K. Hanabusa and H. Shirai, Phys. Chem. Chem. Phys., 1999, 1, 2749–2753 RSC.
- E. Zygadło-Monikowska, Z. Florjańczyk, E. Wielgus-Barry and E. Hildebrand, J. Power Sources, 2006, 159, 392–398 CrossRef.
- Z. Gao, L. Kong, R. Jin, X. Liu, W. Hu and G. Gao, J. Mater. Chem. C, 2020, 8, 11119 RSC.
- N. Wen, B. Jiang, X. Wang, Z. Shang, D. Jiang, L. Zhang, C. Sun, Z. Wu, H. Yan, C. Liu and Z. Guo, Chem. Rec., 2020, 20, 773–792 CrossRef CAS PubMed.
- W. Wieczorek, Z. Fldrjanczyk and J. R. Stevens, Electrochim. Acta, 1995, 40, 2327–2330 CrossRef CAS.
- J. Przyluski, Z. Poitarzewski and W. Wieczorek, Polymer, 1997, 39, 4343–4347 CrossRef.
- X. Wang, J. You and Y. Wu, RSC Adv., 2021, 11, 22461–22466 RSC.
- A. Katzenberg, C. Muñoz Davila, B. Chen, T. Siboonruang and M. A. Modestino, ACS Appl. Polym. Mater., 2020, 2, 2046–2054 CrossRef CAS.
- K. P. Radha, S. Selvasekarapandian, S. Karthikeyan, M. Hema and C. Sanjeeviraja, Ionics, 2013, 19, 1437–1447 CrossRef CAS.
- M. Kumar and S. S. Sekhon, Eur. Polym. J., 2002, 38, 1297–1304 CrossRef CAS.
- H. Pu and P. Huang, Mater. Lett., 2006, 60, 1724–1727 CrossRef CAS.
- S. Abdurrahmanoglu and O. Okay, J. Macromol. Sci., Part A: Pure Appl.Chem., 2008, 45, 769–775 CrossRef CAS.
- Y. Zhang, Y. Duan and T. Wang, Phys. Chem. Chem. Phys., 2014, 16, 26261 RSC.
- F. B. Brahim and A. Bulou, Mater. Chem. Phys., 2011, 130, 24–32 CrossRef.
- F. G. C. Tessarolli, S. T. S. Souza, A. S. Gomes and C. R. E. Mansur, J. Appl. Polym. Sci., 2019, 136, 47556 CrossRef.
- G. Yu, C. Yang, N. Dan, W. Dan and Y. Chen, Des. Monomers Polym., 2021, 24, 293–304 CrossRef CAS PubMed.
- L. Mao, A. T-Raissi, C. Huang and N. Z. Muradov, Int. J. Hydrogen Energy, 2011, 36, 5822–5827 CrossRef CAS.
- R. H. Chena, C. C. Yen and C. S. Shern, J. Appl. Phys., 2005, 98, 044104 CrossRef.
- S. S. Zumdahl and S. A. Zumdahl, Chemistry-Experimental Chemistry, Houghton Mifflin Harcourt, 9th edn, 2014 Search PubMed.
- S. Miyazawa, R. Hosono, R. Osuga, J. Nomura Kondo and S. Uchida, Acta Crystallogr., Sect. C: Struct. Chem., 2018, 74, 1289–1294 CrossRef CAS PubMed.
- M. B. Karimi, F. Mohammadi and K. Hooshyari, Phys. Chem. Chem. Phys., 2020, 22, 2917–2929 RSC.
- C. Ajith, A. P. Deshpande and S. Varughese, J. Polym. Sci., Part B: Polym. Phys., 2016, 54, 1087–1101 CrossRef CAS.
- S. Y. Lee, A. Ogawa, M. Kanno, H. Nakamoto, T. Yasuda and M. Watanabe, J. Am. Chem. Soc., 2010, 132, 9764–9773 CrossRef CAS PubMed.
|
This journal is © The Royal Society of Chemistry 2022 |