DOI:
10.1039/D2RA01102F
(Review Article)
RSC Adv., 2022,
12, 16927-16941
Applications of nanocomposites based on zeolitic imidazolate framework-8 in photodynamic and synergistic anti-tumor therapy
Received
19th February 2022
, Accepted 6th May 2022
First published on 9th June 2022
Abstract
Due to the limitations resulting from hypoxia and the self-aggregation of photosensitizers, photodynamic therapy (PDT) has not been applied clinically to treat most types of solid tumors. Zeolitic imidazolate framework-8 (ZIF-8) is a common metal–organic framework that has ultra-high porosity, an adjustable structure, good biocompatibility, and pH-induced biodegradability. In this review, we summarize the applications of ZIF-8 and its derivatives in PDT. This review is divided into two parts. In the first part, we summarize progress in the application of ZIF-8 to enhance PDT and realize theranostics. We discuss the use of ZIF-8 to avoid the self-aggregation of photosensitizers, alleviate hypoxia, increase the PDT penetration depth, and combine PDT with multi-modal imaging. In the second part, we summarize how ZIF-8 can achieve synergistic PDT with other anti-tumor therapies, including chemotherapy, photothermal therapy, chemodynamic therapy, starvation therapy, protein therapy, gene therapy, and immunotherapy. Finally, we highlight the challenges that must be overcome for ZIF-8 to be widely applied in PDT. To the best of our knowledge, this is the first review of ZIF-8-based nanoplatforms for PDT.
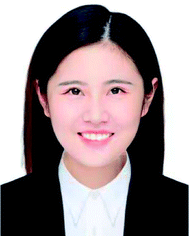 Wen Kang | Wen Kang, was born in Jiangsu, China, in 1997. She received her B. S. Med degree (2015) from Jiangsu University and is studying for a M. S. degree at Nanjing Medical University. Her current research focuses on the molecular imaging fields. |
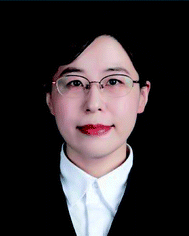 Ying Tian | Ying Tian received her B. S. degree from Dezhou University in 2006, obtained her M. S. degree in biochemistry and molecular biology from Nanjing Normal University in 2009, respectively. She got a PhD degree in clinical medicine from Nanjing University in 2020. Since 2010, she has been working as an associate researcher at Jinling hospital. From 2021, she changed her unit to affiliated hospital of Nanjing University of Chinese Medicine, and further continued her research in diagnosis and treatment of tumor. |
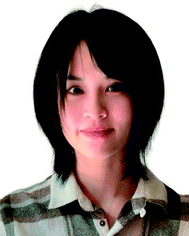 Ying Zhao | Ying Zhao received her B. S. degree and PhD degree in Clinical Medicine from Nanjing University in 2015 and 2018, respectively. After her PhD she joined the Department of Radiology, Nanjing First Hospital, and worked on molecular imaging. Her research focuses on the nanomedicine, multi-modality imaging and synergistic therapy of tumor. |
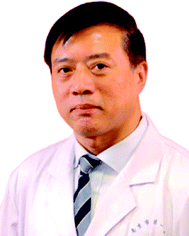 Xindao Yin | Xindao Yin, received his B. S. (1989) and M. S. degree (1996) from Suzhou University. He obtained his PhD in Medical Imaging and Nuclear Medicine from Fudan University. Since 2009 he has become a chief physician. He is an associate professor at Nanjing Medical University. His research interests include molecular imaging, functional imaging and diagnostic imaging. |
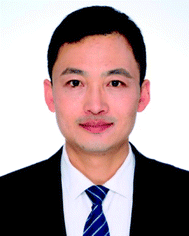 Zhaogang Teng | Zhaogang Teng received his B. S. (2004) and PhD (2009) from Jilin University. He conducted postdoctoral research at Fudan University from 2009 to 2011. Then he worked at Jinling Hospital from 2011 to 2019. He currently works at Department of Materials Science and Engineering of University of Posts and Telecommunications. He has published more than 100 peer reviewed papers. His research interests focus on the synthesis of porous nanoparticle, interfacial chemistry, and nanomedicine. |
Introduction
Cancer is an urgent problem that threatens human life and health,1 and how to cure cancer at the lowest possible cost is an important issue. Traditional cancer treatments such as surgery, chemotherapy, radiation therapy, and immunotherapy have several side effects.2–4 The application of nanomaterials in the biomedical field provides a new direction for cancer researchers. Nanomaterials can be applied to facilitate controlled drug transport, reduce side effects in normal tissues, and improve the curative effect of cancer treatment.5,6 At present, liposomes, polymeric micelles, and inorganic nanoparticles [NPs; e.g., gold NPs (AuNPs), magnetic NPs, upconversion NPs (UPNPs), and mesoporous silica NPs] are widely used in drug delivery systems.7,8 However, the hypersensitivity of liposomes, immunogenicity and complicated biological characteristics of polymeric micelles, and the toxicity of inorganic NPs restrict their clinical applications.9–12 In recent years, metal–organic frameworks (MOFs) have attracted considerable attention as a new type of nanomaterial. MOFs are a class of porous materials composed of metal ions or their clusters coordinated with organic ligands.13 Conventional MOFs have various advantages, including ultra-high porosity, high surface area, and adjustable structure,14–16 giving MOFs great potential in gas storage,17 chemical separation,18,19 catalysis,20,21 sensing,22 drug delivery,23 and other fields. Nanoscale MOFs (nMOFs) display high drug loading efficiency, high biodegradability, and low biological toxicity.24 Thus, nMOFs are regarded as ideal nanocarriers of biomacromolecules.25
Zeolitic imidazolate framework-8 (ZIF-8) is a common MOF constructed from zinc ions and 2-methylimidazole (H-MeIM). ZIF-8 was first synthesized by Park and colleagues in 2006 via a solvothermal method.26 In this solvothermal synthesis, zinc nitrate tetrahydrate and H-MeIM were mixed and dissolved in dimethylformamide (DMF). After heating (5 °C min−1) the solution to 140 °C and keeping this state for 24 h, the solution was cooled (0.4 °C min−1) to room temperature. Then the mother liquor was removed, and chloroform was added. Colorless polyhedral crystals were collected from the upper layer, washed with DMF, and dried in air to obtain ZIF-8. In comparison to other MOFs, ZIF-8 has better biocompatibility because it is composed of constituents of the physiological system.27,28 Under physiological conditions (pH 7.4) and alkaline conditions, ZIF-8 shows excellent chemical and thermal stability. However, acidic conditions (pH 5.0–6.0) destroy the coordination between H-MeIM and zinc ions, leading to the decomposition of the ZIF-8 structure.26 This feature allows the construction of pH-controlled drug delivery systems based on ZIF-8.29 Since the first solvothermal synthesis of ZIF-8, additional solvothermal methods along with microwave-assisted, sono-chemical, mechanochemical, dry-gel, microfluidic, and high-throughput methods have been reported to synthesize ZIF-8 (Fig. 1).26,30–36 ZIF-8 NPs with different average particle sizes can be obtained using different synthetic methods. Among reported methods, the solvothermal synthesis of ZIF-8 in DMF results in the largest particle size (approximately 150–200 μm). ZIF-8 with relatively uniform particle sizes can be synthetized via sono-chemical routes.
 |
| Fig. 1 SEM images and particle sizes of ZIF-8 samples prepared using different synthetic methods. [This figure has been reproduced from ref. 36 with permission from Elsevier Inc., copyright 2015]. | |
The delivery of functional molecules by ZIF-8 is typically based on pore encapsulation, the core–shell structure, surface attachment, ion doping, and in situ growth (Fig. 2).37–40 However, due to the small apertures (diameter of approximately 3.4 Å) of ZIF-8,41 some drugs can only be adsorbed on the surface of ZIF-8. To solve this problem, Tsung and colleagues developed a general one-pot synthetic route to encapsulate small molecules and improve the drug-loading capacity of ZIF-8; they incorporated fluorescein to imitate drugs that are larger than the ZIF-8 apertures. Zinc nitrate hexahydrate (150 mg) and H-MeIM (330 mg) were dissolved in 7.15 mL of methanol. After fluorescein-containing methanol solution was mixed with the zinc solution, H-MeIM solution was added into the zinc-based solution under magnetic stirring for 5 min. The change in color of the solution from bright green to milky white indicated the formation of ZIF-8 NPs. The obtained solution was centrifuged at 7000 rpm for 10 min and washed with methanol to obtain the fluorescein-loaded ZIF-8.28 This general process provides an effective method to embed different therapeutic molecules in ZIF-8 and expands the available ZIF-8-based strategies for tumor treatment.
 |
| Fig. 2 Schematic overview of common strategies to deliver functional molecules and the applications of ZIF-8 nanoplatforms in photodynamic therapy and synergistic therapy. | |
Generally speaking, photodynamic therapy (PDT) is a kind of phototherapy in which singlet oxygen (1O2) and reactive oxygen species (ROS) are produced to kill tumor cells at the areas of photosensitizer (PS) accumulation under light irradiation at a specific wavelength [generally ultraviolet, visible, or near-infrared (NIR) light] and dose.42–44 PDT kills tumor cells directly or indirectly by inducing cell apoptosis, necrosis, and/or autophagy, triggering inflammation and immune response, and damaging the vasculature system.45–47 For PDT to be effectively applied in cancer treatment, a combination of light, a PS, and O2 is required. However, the hypoxia of tumor sites and the nonselective distribution of PSs limit the therapeutic efficacy of conventional PDT.46 Because ZIF-8 can be used to load PSs,48 it has great prospects in PDT for cancer. This review summarizes PDT-related nanocomposites based on ZIF-8 and its derivatives (Table 1) and discusses problems related to these nanoplatforms.
Table 1 Summary of nanoplatforms based on ZIF-8 and its derivatives for monotherapy or synergistic PDT (* indicates a derivative-based nanoplatform)
Therapy modalities |
Nanoplatforms |
Payloads |
Ref. |
Monotherapy |
PS delivery |
ZnPc@ZIF-8 |
ZnPc |
53 |
ZnPc–COOH@ZIF-8 |
ZnPc–COOH |
54 |
ZIF-8@Ce6-HA |
Ce6 |
57 |
MPEG2000-ZIF/PC |
PC |
64 |
PS@ZIF-8-PMMA-S-S-mPEG |
D–A photosensitizers |
68 |
Alleviation of hypoxia |
CAT-PS-ZIF@Mem |
AlPcS4, CAT |
75 |
UCNPs/MB@ZIF-8@catalase |
UCNPs, MB, CAT |
78 |
Au@ZIF-8 |
AuNPs, Ce6 |
84 |
Increase the penetration depth |
UCNPs-g-C3N4–CDs@ZIF-8 |
g-C3N4, CDs, UCNPs |
89 |
Multi-modal imaging-guided PDT |
BSA–MnO2/Ce6@ZIF-8 |
Ce6, (BSA)-MnO2 |
94 |
ZIF-8–IR820–MnPc–HA |
IR820, MnPc |
99 |
![[thin space (1/6-em)]](https://www.rsc.org/images/entities/char_2009.gif) |
Synergistic PDT |
PDT and chemotherapy |
g-C3N4@ZIF-8 |
g-C3N4, DOX |
102 |
AuNCs@MOF-DOX |
AuNCs, DOX |
103 |
F127–MnO2-ZIF@DOX/C3N4 |
g-C3N4, MnO2, DOX |
105 |
ZDZP@PP |
DOX, PpIX |
106 |
BCP/Cit-Fe(III)@ZIF-8 |
DOX, BCP, Cit-Fe(III) |
108 |
FZIF-8/DOX-PD-FA - |
DOX, Ce6 |
112 |
PDT and PTT |
Fe3O4/ZIF-8-Au25 |
Au25(SR)18−, Fe3O4 |
48 |
PDAs-MB-CAT-ZIF-8 |
MB, CAT, PDAs |
118 |
ZCNs* |
|
119 |
PDT and CDT |
O2–Cu/ZIF-8@Ce6/ZIF-8@F127 |
O2, Cu2+, Ce6 |
71 |
PDT and ST |
UCNPs/TAPP@ZIF-8@Catalase/GOx |
UCNPs, TAPP, CAT, GOx |
73 |
PEG-COOH@Enzymes@TPP–DNB@ZIF-8 |
TPP–DNB, CAT, GOx |
130 |
PDT and protein therapy |
Ce6/Cyt c@ZIF-8/HA |
Cyt c, Ce6 |
134 |
PDT and gene therapy |
DNAzyme@ZIF-8 |
DNAzyme |
138 |
PDT, PTT, and chemotherapy |
FeNC@PAA* |
|
143 |
PDT, CDT, and ST |
Ce6/GOx@ZIF-8/PDA@MnO2 |
GOx, MnO2, Ce6 |
146 |
PDT, CDT, and chemotherapy |
MIL-88-ICG@ZIF-8-DOX |
ICG, DOX |
147 |
(DOX and ICG)@H-PMOF@mem* |
ICG, DOX |
150 |
PDT, PTT, chemotherapy, and immunotherapy |
CuZPMn@CpG |
PpIX, CuS, DOX, CpG, MnO2 |
151 |
Individual PDT nanoplatforms
PS delivery. PSs, which are one of the three crucial factors in PDT, largely determine the therapeutic efficiency of PDT. PSs are usually divided into different generations. First-generation PSs are complex natural mixtures and include hematoporphyrin derivatives.49 Photofrin®, the first Food and Drug Administration-approved PS for clinical treatment, is excited by a 630 nm laser, leading to poor tissue penetration.50,51 Second-generation PSs are pure synthetic compounds. Most second-generation PSs are based on porphyrin and chlorin structures that absorb in the region of 650–750 nm. Compared to first-generation PSs, second-generation PSs are irradiated by light with a longer wavelength (650–850 nm) and can penetrate more deeply into tissue.52 Second-generation PSs usually have poor tumor selectivity, and most are hydrophobic, leading to self-aggregation in aqueous solution. As a result, the fluorescence is rapidly quenched, leading to a local reduction in ROS generation.24 Moreover, the poor water solubility of second-generation PSs limits their application by intravenous administration. To increase the PS solubility and enhance PS specific accumulation in tumors, third-generation PSs have been developed by combining traditional second-generation PSs with drug delivery systems including liposomes, NPs, and monoclonal antibodies.49 Because the therapeutic efficacy of third-generation PSs depend on actual PSs, the limitations of traditional PSs need to be overcome. For example, zinc(II) phthalocyanine (ZnPc) was enclosed in the micropores of ZIF-8 to form ZnPc@ZIF-8 via a one-step coprecipitation route to prevent the self-aggregation of ZnPc and fluorescence quenching in aqueous solution.53 ZnPc@ZIF-8 shows good biodegradability because of its disassembly in the acidic tumor microenvironment (TME). Song et al. also developed a pH-responsive drug delivery system based on ZIF-8, termed ZnPc–COOH@ZIF-8.54 ZnPc–COOH@ZIF-8 realized passive tumor targeting via the release of ZnPc in the acidic TME. Similarly, hydrophobic Chlorin e6 (Ce6) was encapsulated into ZIF-8 to prevent its self-aggregation via a one-pot process.51,55–57 The surface of ZIF-8 was modified with hyaluronic acid (HA), a significant component of the extracellular matrix,58 via covalent bonds, resulting in an active targeting effect. CD44 and CD44-like receptors are relatively over-expressed in many solid tumors.59,60 CD44 can bind HA fragments via HA-binding domains in a region (amino acids 31–120 at the N-terminus) homologous to the B loop of cartilage link protein.61,62 Compared with ZIF-8@Ce6, ZIF-8@Ce6-HA has a longer blood circulation time and lower hepatotoxicity due to the presence of HA.ZIF-8 has also been employed to encapsulate phycocyanin, an unstable new PS, via co-precipitation.63,64 Together, the ZIF-8 skeleton and MPEG2000–COOH modified on the surface of ZIF-8 greatly improved the stability and cell uptake of phycocyanin. At the same time, papaverine, an inhibitor of mitochondrial complex I,65 combined with MPEG2000-ZIF/phycocyanin to overcome hypoxia by restricting mitochondrial respiration at tumor sites and increasing ROS production for efficient PDT.
Another way to overcome PS inefficiency is to introduce donor (D) and acceptor (A) molecules to develop D–A PSs.66,67 For example, Wang et al. utilized ZIF-8 to construct a nanocarrier (PS@ZIF-8-PMMA-S–S-mPEG) to handle the problem that water molecules reduce the efficiency of D–A PSs (Fig. 3).68 The carrier realized the self-assembly of PS-loaded ZIF-8 in vivo. In this system, poly(methyl methacrylate) (PMMA) and disulfide-linked methoxy polyethylene glycol (S–S-mPEG) were connected with ZIF-8. The reduction of the disulfide bond and cleavage of mPEG led to the self-assembly of PS@ZIF-8-PMMA by PMMA fusion between adjacent nanoplatforms in tumor cells. These self-assemblies, which had sizes exceeding 200 nm, helped reduce drug extravasation and extend the retention time of organic PSs in tumors.69 ZIF-8 can separate PSs from water and carry O2 for PDT, thereby enhancing ROS generation in cancer PDT.
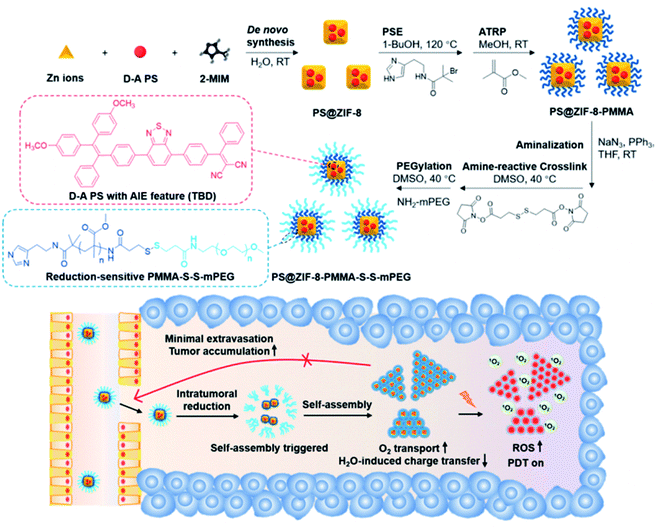 |
| Fig. 3 Schematic of the self-assembly of PS@ZIF-8-PMMA-S–S-mPEG. [This figure has been reproduced from ref. 68 with permission from American Chemical Society, copyright 2020]. | |
Alleviation of hypoxia. The TME is characterized by hypoxia, resulting in the low efficacy of PDT.70 Existing strategies employed to alleviate hypoxia include the use of O2 carriers, the adjustment of the TME, synergistic PDT, hypoxia-independent/dependent PDT and fractional PDT.11 Researchers have directly loaded O2, catalase (CAT), and CAT-like materials on nanoplatforms.71–74 Cheng et al. co-embedded CAT as an O2 generator and a second-generation photosensitizer [Al(III) phthalocyanine chloride tetrasulfonic acid (AlPcS4)] in ZIF-8 to alleviate hypoxia. In other studies, the surface of a CAT-PS-ZIF-8 nanocomposite was covered with cancer cell membrane (Mem) to form CAT-PS-ZIF@Mem,75 which delivered abundant Mem proteins and mimicked the antigenic diversity of the source cells to avoid an immune response and prolong blood circulation,76,77 thereby improving the specific delivery of the PS. Cai et al. modified the surface of ZIF-8 with CAT to construct a highly productive NIR/hydrogen peroxide (H2O2)-responsive core–shell nanocomposite (termed UCNPs/MB@ZIF-8@catalase).78 UCNPs (NaYF4: 60% Yb/2% Er) and methylene blue (MB) were incorporated with ZIF-8 via a one-pot synthetic method. UCNPs are highly photostable for NIR imaging and emit anti-Stokes luminescence at wavelengths shorter than the absorption wavelength.79–81 The combination of UCNPs and MB can guarantee fluorescence resonance energy transfer due to the spectral overlap between UCNP emission and MB absorption, which facilitates the production and release of 1O2 and promotes efficient PDT.82,83ZIF-8 was also used as a vehicle for CAT-like materials in an O2 self-sufficient nanoplatform (Au@ZIF-8) in which AuNPs were connected to the surface of ZIF-8.84 In addition to emitting fluorescence, AuNPs can simulate CAT-like properties.85 Au@ZIF-8 can catalyze endogenous H2O2 to produce O2 and relieve tumor hypoxia, thereby enhancing the curative effect of PDT. ZIF-8 plays an essential role in stabilizing the AuNPs so that they are not removed from blood circulation.
Increase the penetration depth. At present, the most common PDT therapeutic window is between 600 and 950 nm. However, the excitation wavelength of most traditional PSs is lower than 780 nm. Short-wavelength light has poor tissue penetration due to its strong attenuation by the UV-visible absorption of oxyhemoglobin and tissue scattering.86 As a result, the in vivo photodynamic effects are limited.87,88 To address this problem, Yang et al. employed dual-modal PDT mediated by high-transmittance NIR light (Fig. 4A).89 The authors co-loaded carbon dots (CDs) and graphitic carbon nitride (g-C3N4) onto ZIF-8 via stepwise water splitting and covered the surface of the nanoplatform with UCNPs through in situ growth. The addition of CDs to the g-C3N4 sheets enhanced the absorption intensity over the absorption range compared to g-C3N4 alone (Fig. 4B).90 In this system, the g-C3N4 and CDs are continuously activated by the UV light emitted from the UCNPs, which transfer deep-penetrating low-energy NIR light to high-energy UV light upon irradiation with a 980 nm laser (Fig. 4C and D). The visible light produced by the CDs can activate g-C3N4 to produce ROS, thereby maximizing light utilization. Many two photon-excited PSs have been developed to overcome the difficulty in activating long-wavelength NIR light (>750 nm) via one-photon excitation and improve tissue penetration.91,92 However, the combination of UCNPs with CDs and g-C3N4 can further increase the penetration depth and efficiency of the laser.
 |
| Fig. 4 (A) Schematic of the anti-tumor mechanism of UCNPs-g-C3N4–CDs@ZIF-8. (B) UV-visible absorbance spectra of g-C3N4, CDs, and g-C3N4–CDs. (C) The emission spectrum upon 360 nm excitation and the absorption spectrum of CDs. (D) The emission spectrum of UCNPs under 980 nm laser excitation and the absorption spectrum of the g-C3N4–CDs PS. [This figure has been reproduced from ref. 89 with permission from American Chemical Society, copyright 2017]. | |
Multi-modal imaging-guided PDT. Although fluorescence imaging, a noninvasive and real-time imaging modality, can be induced by PSs during PDT, this technique is not commonly used in clinical settings at present.93 Theranostic nanoprobes that can simultaneously realize traditional medical imaging and PDT have been reported. For example, Sun et al. reported a novel pH-responsive magnetic resonance imaging (MRI) drug delivery system (BSA–MnO2/Ce6@ZIF-8).94 This system was formed via a one-pot route in which Ce6 was integrated into the ZIF-8 matrix followed by loading bovine serum albumin (BSA)–MnO2 NPs with CAT-like activity on the surface of ZIF-8. MnO2 generated Mn2+ by reacting with H2O2 in the acidic TME for T1-weighted MRI (Fig. 5A and B).95,96 Meanwhile, the concentration of O2 increased with H2O2 decomposition (Fig. 5C). This strategy integrates MRI with Ce6-mediated fluorescence imaging based on O2 production to achieve multi-modal imaging-guided PDT.
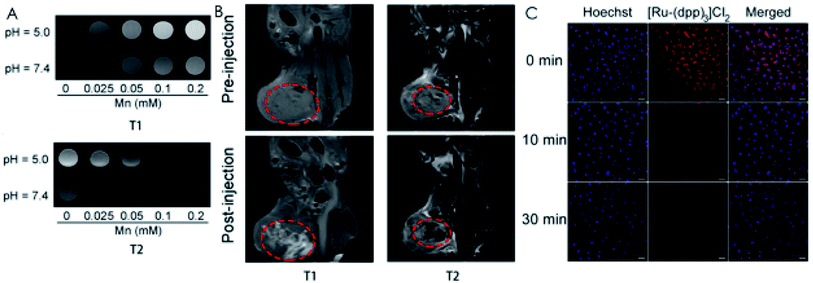 |
| Fig. 5 (A) T1-and T2-weighted MRI images of NPs at pH 5.0 and 7.4. (B) In vivo T1-and T2-weighted MRI images before and after injection with NPs. (C) CLSM images of HeLa cells incubated with BSA–MnO2/Ce6@ZIF-8 for different times after staining with Hoechst 33342 and [Ru-(dpp)3]Cl2. [This figure has been reproduced from ref. 94 with permission from American Chemical Society, copyright 2019]. | |
Photoacoustic (PA) imaging has become a promising technique for deep tumors due to the great acoustic depth of penetration and optical resolution.97,98 Qu et al. designed ZIF-8–IR820–MnPc–HA (ZIMH) NPs that combined PA imaging with NIR-II fluorescence imaging to improve the visualization and localization of tumors (Fig. 6).99 To form the ZIMH NPs, IR820 and MnPc were encapsulated uniformly in the porous structure of ZIF-8 to reduce quenching and produce 1O2 under 808 nm irradiation. This multi-modal imaging-guided PDT provides a new strategy for the early and accurate diagnosis and treatment of tumors.
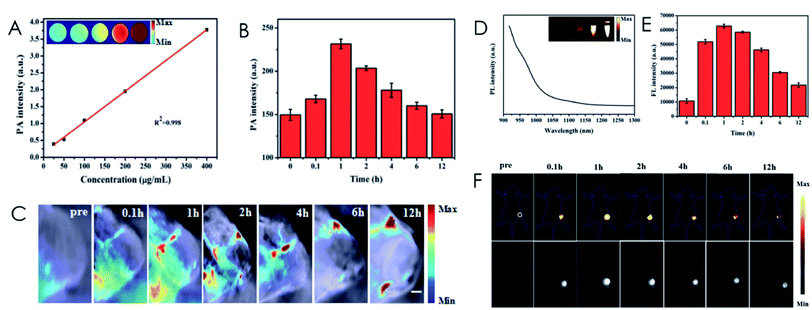 |
| Fig. 6 The linear relationships between the PA (A) and fluorescence (D) intensity and the ZIMH concentration. PA (B) and fluorescence (E) intensity of the tumor sites of mice in vivo at different times after the injection of ZIMH. PA (C) and fluorescence (F) images of the tumors at different time points after ZIMH injection. [This figure has been reproduced from ref. 99 with permission from Royal Society of Chemistry, copyright 2021]. | |
Synergistic PDT nanoplatforms
Synergistic PDT and chemotherapy. Chemotherapy is one of the most widely used treatment modalities. However, the multiple disadvantages of chemotherapeutic drugs, including serious side effects, drug tolerance, and nonselective drug distribution and release, severely hinder their practical application.100,101 Currently, most clinical-stage nanomedicines for chemotherapy are based on liposomes.7 However, considering the hypersensitivity and immune suppression of liposomes, nMOFs and particularly ZIF-8 may be better carriers for chemotherapeutic drugs because of their high biodegradability and low biological toxicity. Since Chen et al. first synthesized photo-chemo combination NPs (g-C3N4@ZIF-8),102 other reports have indicated that chemotherapeutic drugs including doxorubicin (DOX) can be co-encapsulated with PSs in ZIF-8. Gold nanocluster (AuNC) PSs with the capacity for ROS generation have also realized efficient photo-chemo effects.103,104 CAT and CAT-like materials including MnO2 nanodots can be further introduced to relieve hypoxia during photo-chemotherapy via the catalysis of endogenous H2O2.105 In a photo-chemotherapeutic nanosystem containing two kinds of MOFs, Ren et al. utilized ZIF-67 as an analog of CAT to encapsulate DOX.106Amphiphilic block copolymer was combined with ZIF-8 as a drug carrier to construct a pH/H2O2 dual-controlled associative PDT and chemotherapy nanoplatform (termed BCP/Cit-Fe(III)@ZIF-8).107,108 To form this nanoplatform, DOX and poly(L-lactic acid)-block-poly(sodium 4-styrenesulfonate) (BCP) were self-assembled in aqueous solution followed by the attachment of ferric citrate [Cit-Fe(III)] through electrostatic interaction. Finally, ZIF-8 was grown on the surfaces of the NPs. Under co-stimulation by H2O2 and visible light and the catalysis of Cit-Fe(III), sufficient ROS were released (Fig. 7).109–111 At the same time, the ROS were oxidized and decomposed sulfonate-containing polymeric vesicles, causing the encapsulated DOX to spread into ZIF-8. Under low pH, ZIF-8 disintegrated to achieve controlled drug release and an excellent anti-cancer effect.
 |
| Fig. 7 (A) Anti-tumor mechanism of BCP/Cit-Fe(III)@ZIF-8 in vivo. (B) Cell viability in the presence of different concentrations of the nanoplatform in the dark and under visible light. (C) Cell viability in the presence of various DOX concentrations after different treatments. (D) Intracellular hydroxyl radicals detected by incubating MCF-7 cells with coumarin under visible light. [This figure has been reproduced from ref. 108 with permission from American Chemical Society, copyright 2019]. | |
In another photo-chemotherapy nanosystem, Qin and his colleagues loaded Gd-doped silicon NPs (Si–Gd NPs) into the ZIF-8 matrix to endow it with MRI properties.112 In this system, the combination of MRI/fluorescence imaging modalities and folic acid polyethylene glycol-maleimide (MaL-PEG-FA) as a cancer target molecule allows for a precise treatment effect.113
Synergistic PDT and photothermal therapy (PTT). PTT is an alternative approach to phototherapy in which photothermal agents (PTAs) are used to convert NIR light into heat.114 PTT usually employs NIR light with a longer wavelength than PDT, resulting in deeper penetration.115 PTAs and PSs can be co-loaded into ZIF-8 using appropriate synthetic methods to form photothermal and photodynamic systems.48,116 Yang et al. co-encapsulated Fe3O4 nanocrystals and Au25(SR)18− clusters in ZIF-8 as PTAs to produce hyperthermal effects and realize MRI.117 The ultrasmall Au25(SR)18− clusters loaded on Fe3O4/ZIF-8-Au25 enhanced the photothermal effect while producing singlet oxygen.48,116 Another reported co-delivery method is to make ZIF-8 encapsulating small molecule PSs grow in situ on the surfaces of large-molecule PTAs to overcome the small pore diameter of ZIF-8.118In addition, ZIF-8 has been directly pyrolyzed to obtain ZIF-8-derived carbon nanoparticles that served as both a PTA and PS rather than using two different drugs.119
Synergistic PDT and chemodynamic therapy (CDT). Previous studies have demonstrated that O2 can be absorbed and stored by ZIF-8.120 Compared with other MOFs with coordination-unsaturated Cu sites (HKUST-1, MCM-152), unmodified ZIF-8 has poor O2 storage capacity.121–123 To address this issue, Cu2+ was doped into the ZIF-8 matrix to construct a pH-responsive nanoplatform (O2–Cu/ZIF-8@Ce6/ZIF-8@F127, designated as OCZCF) to enhance O2 adsorption (Fig. 8A).71 The OCZCF nanoplatform can carry O2 by itself via Cu2+-doped ZIF-8 (Cu/ZIF-8), and the quantity of O2 loaded in Cu/ZIF-8 is twice that loaded in the ordinary ZIF-8 matrix (Fig. 8B). In addition, Cu2+ and the byproduct Cu+ can participate in Fenton-like reactions to realize CDT, which transforms intracellular H2O2 into highly cytotoxic hydroxyl radicals (˙OH) to eliminate tumor cells containing excessive glutathione (GSH) (Fig. 8C).124,125 Based on experimental results, OCZCF is a promising, highly efficient nanoplatform for synergetic PDT and CDT (Fig. 8D).
 |
| Fig. 8 (A) Schematic illustration of the synthesis and therapeutic mechanism of OCZCF. (B) O2 adsorption isotherms of ZIF-8 and Cu/ZIF-8. (C) The correlation between GSH concentration and OCZCF concentration. (D) Photographs of mice who received different treatments. [This figure has been reproduced from ref. 71 with permission from American Chemical Society, copyright 2019]. | |
Synergistic PDT and starvation therapy (ST). Cascade reactions can be employed to amplify the anticancer efficacy of existing treatments.126 Glucose oxidase (GOx), a popular drug used for ST, catalyzes the oxidization of glucose into gluconic acid and H2O2.127 As the catalysis of GOx causes the TME to become more acidic and hypoxic, GOx-based nanocomposites usually have the capacity for cascade reaction.128,129 You et al. constructed a core–shell, self-driven UCNPs/TAPP@ZIF-8@catalase nanomotors and loaded GOx and CAT on their surfaces.73 CAT catalyzed the decomposition of GOx-catalyzed and endogenous H2O2 to provide a driving force for GOx catalysis. The experimental data indicated that the nanomotors generated positive feedback into the therapeutic process under cascade reactions driven by two enzymes, vastly improving the synergistic PDT and ST effect. While the combination of GOx and CAT, 2,4-dinitrobenzenesulfonyl chloride-quenched porphyrin (TPP–DNB) as a GSH-activated generator was loaded into ZIF-8.130,131 ZIF-8 and TPP-DNB realized the drug release at low pH and antitumor treatment activated by excessive GSH in the TME.
Synergistic PDT and protein therapy. Hydrolysis and denaturation in vivo and the immunogenicity of proteins hinder the clinical applications of protein therapy.132,133 Biomineralization is a useful strategy to combine biomacromolecules, including therapeutic proteins, and MOFs.38 Cytochrome c (Cyt c) and Ce6 were encapsulated together into ZIF-8 via a one-pot biomimetic mineralization strategy to convey and protect therapeutic proteins.134 Cyt c can induce tumor cell apoptosis and retain the peroxidase/CAT-like activity under hypoxic conditions to alleviate tumor hypoxia and enhance PDT.135
Synergistic PDT and gene therapy. Although a promising tumor therapy, gene therapy, which can induce gene silencing, antisense therapy, RNA interference, gene and genome editing, has been limited to inefficient delivery to tumor tissue. Metallic NPs, silica NPs, carbon-derived NPs, liposomes, and polymeric NPs have been designed for gene therapy.136,137 However, the cytotoxicity, biocompatibility, biodegradability, and anaphylactic effect of these NPs require further study. Wang and colleagues loaded Ce6-modified DNAzymes as a gene therapy agent into ZIF-8 (ref. 138 and 139) to achieved simultaneous gene therapy and PDT. Under low pH, the release of Ce6-DNAzyme was accompanied by the disintegration of ZIF-8, and the byproduct Zn2+ acted as a cofactor of DNAzyme to overcome the low efficiency of intracellular delivery and insufficient supply of cofactor.140
Synergistic PDT, PTT, and chemotherapy. ZIF-8 was reported to be carbonized to produce fluorescent carbon nanodots for biosensing via low-temperature annealing.141 ZIF-8-derived nanomaterials are still rarely studied in cancer therapies.142 Sui et al. designed a simple multi-functional nanoplatform (FeNC@PAA) based on a ZIF-8 derivative for cooperative tri-modal therapy.143 The precursor ZIF-8 was simply pyrolyzed to synthesize Fe–N co-doped carbon (FeNC) NPs (Fig. 9A). Metal–nitrogen–carbon NPs derived from MOFs, including Zn–N–C nanomaterials synthesized via the pyrolysis of ZIF-8, have porphyrin-like zinc centers and can be used as PSs for PDT.144 The porphyrin-like metal center of the FeNC@PAA nanoplatform can be used for PDT145 and boost the Fenton-like reaction to produce ·OH for CDT via Fe2+ (Fig. 9B). Experimental results suggest that FeNC@PAA has a high NIR absorption rate and a photothermal conversion efficiency of up to 29.15% (Fig. 9C).
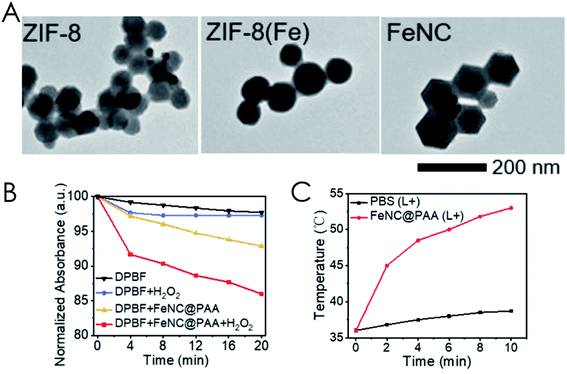 |
| Fig. 9 (A) TEM images of ZIF-8 (Zn), ZIF-8 (Fe/Zn), and FeNC NPs. (B) Decay curves of DPBF-normalized absorption at 410 nm under different conditions and 808 nm laser irradiation (0.5 W cm−2). (C) Temperature change of the tumor site during irradiation (0.3 W cm−2, 10 min). [This figure has been reproduced from ref. 143 with permission from American Chemical Society, copyright 2021]. | |
Synergistic PDT, CDT, and ST. ST-triggered glucose decomposition consumes O2 at tumor sites while providing H2O2, which is necessary for CDT but limits PDT. MnO2 can both provide O2 by mimicking CAT and mediate the Fenton reaction via Mn2+ to facilitate simultaneous PDT and CDT. In consideration of this characteristic, Zhang et al. combined MnO2 and GOx to construct intelligent cascade nanoparticles with O2 production and GSH/glucose consumption capacity CGZPM (Fig. 10).146 In this design, ZIF-8 not only serves as a nanocarrier of Ce6 and Gox, it also directly initiates the surface polymerization of dopamine to prevent the premature release of Ce6 and GOx. As a therapeutic nanosystem, CGZPM reached the goal of overcoming limitations of hypoxia and GSH overexpression in TME and conducting MRI.
 |
| Fig. 10 Schematic of the reaction mechanism of CGZPM in vivo. [This figure has been reproduced from ref. 146 with permission from Elsevier Inc., copyright 2021]. | |
Synergistic PDT, CDT, and chemotherapy. Different kinds of drugs loaded onto the same carrier may interact with each other, affecting the drug efficacy. Therefore, the use of various carriers to transport different drugs in a drug delivery system can be considered in combined anticancer treatments. Wu et al. built core–shell tri-modal therapeutic nanoparticles (MIL-88-ICG@ZIF-8-DOX) based on MIL-88 as the core and ZIF-8 as the shell.147 MIL-88 can intelligently adjust the aperture size to adapt to the size of indocyanine green (ICG), thereby improving the drug stability without harming the framework topology.148,149 In addition, the iron ions in MIL-88 can be used for CDT and also continuously generate O2 to enhance the efficacy of PDT through a Fenton-like reaction. Another method of carrying ICG/DOX is with the help of hollow porphyrinic MOF (H-PMOF) NPs which were synthesized by a ZIF-8 template used to load ICG (Fig. 11A and B).150 The loading capacity of H-PMOF for DOX and ICG was over six times that of non-hollow porphyrinic MOF NPs. The nanoplatform was also covered with Mem to provide homologous tumor-targeting and immune-escaping ability for precise synergistic therapy (Fig. 11C and D).
 |
| Fig. 11 (A) Schematic of the synthesis and reaction mechanism of DIHPm. (B) TEM images of DIHPm. (C) Relative cellular uptake of DIHPm against various cell lines (A549, U87MG, and 4T1). (D) Fluorescence images of 4T1 tumor-bearing mice at different timepoints after the intravenous injection of DIHPm or DIHP. [This figure has been adapted from ref. 150 with permission from American Chemical Society, copyright 2021]. | |
Synergistic PDT, PTT, chemotherapy, and immunotherapy. The synthesis of nanomaterials that integrate multiple agents into therapeutic multifunctional NPs is limited by the interactions between different drugs and the complex synthetic procedures. Using a simple one-pot route, Yang et al. co-loaded the ZIF-8 matrix with a variety of therapeutic agents, including PpIX for PDT, CuS NPs for PTA, and DOX for chemotherapy, for synergistic PDT, PTT, and chemotherapy (Fig. 12).151 Negative cytosine–phosphate guanine (CpG), which is recognized by Toll-like receptor 9 (TLR9),152 was adsorbed onto the surface of positive ZIF-8 via electrostatic interactions for immunotherapy, which is a promising auxiliary therapy for controlling cancer recurrence and metastasis.153,154 A polydopamine (PDA) layer was then grown to enhance the PTT effect and protect negative CpG. The system was covered by an outer layer of MnO2, which served as a GSH-responsive MRI contrast agent. Experiments confirmed that this synergistic nanoplatform achieved MRI-guided multi-modality therapy and inhibited tumor recurrence and metastasis via immune memory.
 |
| Fig. 12 Schematic diagram of the synthesis and applications of CuZPMn@CpG nanocomposite. [This figure has been reproduced from ref. 151 with permission from Royal Society of Chemistry, copyright 2018]. | |
Conclusion and outlook
Multifunctional nanoplatforms based on ZIF-8 and its derivatives have shown outstanding application prospects in PDT. The porosity and easy modification of the ZIF-8 skeleton largely overcome the low photosensitizer efficiency and hypoxia in conventional PDT. The reaction of ZIF-8 in acidic conditions also contributes to the precise release of drugs in tumor sites. ZIF-8 can also be used as an intermediary to realize synergistic PDT and other anti-tumor therapies by delivering different small-molecule therapeutic agents. However, ZIF-8 still has some weaknesses.
(1) As a carrier in PDT, ZIF-8 still needs to face challenges of low accumulation at tumor sites and unclear metabolic mechanisms in vivo. Without any surface modification, ZIF-8 has a short blood circulation time and low accumulation in tumor cells.155 Thus, modifiers must be added to ZIF-8 to overcome these limitations. In addition, most studies on ZIF-8-based nanomaterials have not explored the metabolic mechanisms and long-term side effects, and the effects of these nanomaterials on the immune system remain unknown.
(2) Although zinc, a component of ZIF-8, is one of the vital transition metal in organisms and essential for life, the accumulation of zinc in cells can damage the cells.156 Chen et al. thought that smaller ZIF-8 nanoplatforms would lead to more zinc accumulation. Although more zinc can provide more ROS, excess zinc can result in cytotoxicity.157 Further studies are needed to design ZIF-8 nanoplatforms with suitable sizes to decrease toxicity to normal tissues.
(3) The design of synergistic therapeutic nanoplatforms based on ZIF-8 remains complicated. Although there is a universal one-pot route to encapsulate small molecules, a simple and versatile way to integrate multiple therapeutic drugs into nanosystems has not been identified.
(4) Most ZIF-8 nanoplatforms for PDT are designed to alleviate hypoxia and deliver PSs. Thus, low tissue penetration depth in PDT remains a problem. Future work could focus on designing better ZIF-8-based PSs to extend the excitation wavelength and increase the penetration depth in PDT.
(5) PDT based on ZIF-8 has been primarily studied at the cell level and/or animal level, and human clinical data are lacking. Whether these nanoplatforms can pass rigorous clinical evaluation is still uncertain. We anticipate that researchers can overcome the remaining problems to build ZIF-8-based nanoplatforms for application in clinical treatment in the near future.
Author contributions
Wen Kang: conceptualization, methodology, software, validation, formal analysis, investigation, resources, data curation, writing – original draft, writing – review & editing.
Ying Tian: conceptualization, methodology, software, validation, data curation, writing – original draft, writing – review & editing, project administration, funding acquisition.
Ying Zhao: software, validation, formal analysis, resources, data curation, writing – review & editing, visualization, project administration, funding acquisition.
Xindao Yin: conceptualization, software, investigation, resources, data curation, writing – review & editing, visualization, supervision, project administration, funding acquisition.
Zhaogang Teng: conceptualization, methodology, validation, investigation, writing – review & editing, supervision, project administration.
Conflicts of interest
The authors declare that they have no known competing financial interests or personal relationships that could have appeared to influence the work reported in this article.
Acknowledgements
This work was supported by National Natural Science Foundation of China (grant numbers 81901806 and 81971681).
References
- R. L. Siegel, K. D. Miller and A. Jemal, Ca-Cancer J. Clin., 2020, 70, 7–30 CrossRef PubMed.
- M. Jablonická, L. Žideková and B. Mladosievičová, Vnitr. Lek., 2021, 67, 26–31 CrossRef.
- M. Dominguez and R. Malani, Curr. Pain Headache Rep., 2021, 25, 33 CrossRef PubMed.
- P. Iglesias, J. C. Sánchez and J. J. Díez, Pituitary, 2021, 24, 630–643 CrossRef CAS PubMed.
- J. Yu, Y. Ju, L. Zhao, X. Chu, W. Yang, Y. Tian, F. Sheng, J. Lin, F. Liu, Y. Dong and Y. Hou, ACS Nano, 2016, 10, 159–169 CrossRef CAS PubMed.
- O. C. Farokhzad and R. Langer, ACS Nano, 2009, 3, 16–20 CrossRef CAS PubMed.
- J. Shi, P. W. Kantoff, R. Wooster and O. C. Farokhzad, Nat. Rev. Cancer, 2017, 17, 20–37 CrossRef CAS PubMed.
- F. Wang, C. Li, J. Cheng and Z. Yuan, Int. J. Environ. Res. Public Health, 2016, 13, 1182 CrossRef PubMed.
- J. L. Paris, A. Baeza and M. Vallet-Regí, Expert Opin. Drug Delivery, 2019, 16, 1095–1112 CrossRef CAS PubMed.
- M. Yokoyama, J. Drug Targeting, 2014, 22, 576–583 CrossRef CAS PubMed.
- L. Larue, B. Myrzakhmetov, A. Ben-Mihoub, A. Moussaron, N. Thomas, P. Arnoux, F. Baros, R. Vanderesse, S. Acherar and C. Frochot, Pharmaceuticals, 2019, 12, 163 CrossRef CAS PubMed.
- N. Thotakura, P. Parashar and K. Raza, Expert Opin. Drug Metab. Toxicol., 2021, 17, 323–332 CrossRef CAS PubMed.
- H.-C. Zhou, J. R. Long and O. M. Yaghi, Chem. Rev., 2012, 112, 673–674 CrossRef CAS PubMed.
- J. L. C. Rowsell and O. M. Yaghi, Microporous Mesoporous Mater., 2004, 73, 3–14 CrossRef CAS.
- K. K. Tanabe, Z. Wang and S. M. Cohen, J. Am. Chem. Soc., 2008, 130, 8508–8517 CrossRef CAS PubMed.
- C. Wang, D. Liu and W. Lin, J. Am. Chem. Soc., 2013, 135, 13222–13234 CrossRef CAS PubMed.
- M. Eddaoudi, J. Kim, N. Rosi, D. Vodak, J. Wachter, M. O'Keeffe and O. M. Yaghi, Science, 2002, 295, 469–472 CrossRef CAS PubMed.
- J. Li, P. M. Bhatt, J. Li, M. Eddaoudi and Y. Liu, Adv. Mater., 2020, 32, 2002563 CrossRef CAS PubMed.
- W.-Q. Tang, J.-Y. Xu and Z.-Y. Gu, Chem.–Asian J., 2019, 14, 3462–3473 CrossRef CAS PubMed.
- H. Wan, Y. Wang, J. Chen, H. M. Meng and Z. Li, Mikrochim. Acta, 2021, 188, 130 CrossRef CAS PubMed.
- Y.-B. Huang, J. Liang, X.-S. Wang and R. Cao, Chem. Soc. Rev., 2016, 46, 126–157 RSC.
- L. Du, W. Chen, P. Zhu, Y. Tian, Y. Chen and C. Wu, Biotechnol. J., 2021, 16, 1900424 CrossRef CAS PubMed.
- J. W. M. Osterrieth and D. Fairen-Jimenez, Biotechnol. J., 2021, 16, 2000005 CrossRef CAS PubMed.
- D. Gao, Y. Gao, J. Shen and Q. Wang, Photodiagn. Photodyn. Ther., 2020, 32, 102026 CrossRef CAS PubMed.
- M.-X. Wu and Y.-W. Yang, Adv. Mater., 2017, 29, 1606134 CrossRef PubMed.
- K. S. Park, Z. Ni, A. P. Côté, J. Y. Choi, R. Huang, F. J. Uribe-Romo, H. K. Chae, M. O'Keeffe and O. M. Yaghi, Proc. Natl. Acad. Sci. U. S. A., 2006, 103, 10186–10191 CrossRef CAS PubMed.
- C. Andreini, L. Banci, I. Bertini and A. Rosato, J. Proteome Res., 2006, 5, 3173–3178 CrossRef CAS PubMed.
- J. Zhuang, C.-H. Kuo, L.-Y. Chou, D.-Y. Liu, E. Weerapana and C.-K. Tsung, ACS Nano, 2014, 8, 2812–2819 CrossRef CAS PubMed.
- J. Yan, C. Liu, Q. Wu, J. Zhou, X. Xu, L. Zhang, D. Wang, F. Yang and H. Zhang, Anal. Chem., 2020, 92, 11453–11461 CrossRef CAS PubMed.
- H.-Y. Cho, J. Kim, S.-N. Kim and W.-S. Ahn, Microporous Mesoporous Mater., 2013, 169, 180–184 CrossRef CAS.
- Q. Shi, Z. Chen, Z. Song, J. Li and J. Dong, Angew. Chem., Int. Ed. Engl., 2011, 50, 672–675 CrossRef CAS PubMed.
- F. Hillman, J. Brito and H.-K. Jeong, ACS Appl. Mater. Interfaces, 2018, 10, 5586–5593 CrossRef CAS PubMed.
- R. Banerjee, A. Phan, B. Wang, C. Knobler, H. Furukawa, M. O'Keeffe and O. M. Yaghi, Science, 2008, 319, 939–943 CrossRef CAS PubMed.
- P. J. Beldon, L. Fábián, R. S. Stein, A. Thirumurugan, A. K. Cheetham and T. Friščić, Angew. Chem., Int. Ed. Engl., 2010, 49, 9640–9643 CrossRef CAS PubMed.
- M. Faustini, J. Kim, G.-Y. Jeong, J. Y. Kim, H. R. Moon, W.-S. Ahn and D.-P. Kim, J. Am. Chem. Soc., 2013, 135, 14619–14626 CrossRef CAS PubMed.
- Y.-R. Lee, M.-S. Jang, H.-Y. Cho, H.-J. Kwon, S. Kim and W.-S. Ahn, Chem. Eng. J., 2015, 271, 276–280 CrossRef CAS.
- A. Dhakshinamoorthy, A. M. Asiri and H. Garcia, Dalton Trans., 2020, 49, 11059–11072 RSC.
- C. Chu, M. Su, J. Zhu, D. Li, H. Cheng, X. Chen and G. Liu, Theranostics, 2019, 9, 3134–3149 CrossRef CAS PubMed.
- Y. Wang, X. Dai, Y. Zhan, X. Ding, M. Wang and X. Wang, Int. J. Biol. Macromol., 2019, 137, 77–86 CrossRef CAS PubMed.
- C. Hu, Y. Yu, S. Chao, H. Zhu, Y. Pei, L. Chen and Z. Pei, Molecules, 2021, 26, 3878 CrossRef CAS PubMed.
- O. Karagiaridi, M. B. Lalonde, W. Bury, A. A. Sarjeant, O. K. Farha and J. T. Hupp, J. Am. Chem. Soc., 2012, 134, 18790–18796 CrossRef CAS PubMed.
- M. C. DeRosa and R. J. Crutchley, Coord. Chem. Rev., 2002, 233–234, 351–371 CrossRef CAS.
- M. L. Agarwal, M. E. Clay, E. J. Harvey, H. H. Evans, A. R. Antunez and N. L. Oleinick, Cancer Res., 1991, 51, 5993–5996 CAS.
- P. Vijayaraghavan, C.-H. Liu, R. Vankayala, C.-S. Chiang and K. C. Hwang, Adv. Mater., 2014, 26, 6689–6695 CrossRef CAS PubMed.
- D. Kessel and N. L. Oleinick, Photochem. Photobiol., 2018, 94, 213–218 CrossRef CAS PubMed.
- D. Van Straten, V. Mashayekhi, H. S. De Bruijn, S. Oliveira and D. J. Robinson, Cancers, 2017, 9, 19 CrossRef PubMed.
- W. M. Star, H. P. A. Marijnissen, A. E. van den Berg-Blok, J. A. C. Versteeg, K. A. P. Franken and H. S. Reinhold, Cancer Res., 1986, 46, 2532–2540 CAS.
- D. Yang, G. Yang, S. Gai, F. He, G. An, Y. Dai, R. Lv and P. Yang, Nanoscale, 2015, 7, 19568–19578 RSC.
- S. Kwiatkowski, B. Knap, D. Przystupski, J. Saczko, E. Kędzierska, K. Knap-Czop, J. Kotlińska, O. Michel, K. Kotowski and J. Kulbacka, Biomed. Pharmacother., 2018, 106, 1098–1107 CrossRef CAS PubMed.
- I. S. Mfouo-Tynga, L. D. Dias, N. M. Inada and C. Kurachi, Photodiagn. Photodyn. Ther., 2021, 34, 102091 CrossRef CAS PubMed.
- R. Baskaran, J. Lee and S.-G. Yang, Biomater. Res., 2018, 22, 25 CrossRef PubMed.
- S. Wang, X. Wang, L. Yu and M. Sun, Photodiagn. Photodyn. Ther., 2021, 34, 102254 CrossRef CAS PubMed.
- D. Xu, Y. You, F. Zeng, Y. Wang, C. Liang, H. Feng and X. Ma, ACS Appl. Mater. Interfaces, 2018, 10, 15517–15523 CrossRef CAS PubMed.
- M.-R. Song, D.-Y. Li, F.-Y. Nian, J.-P. Xue and J.-J. Chen, J. Mater. Sci., 2018, 53, 2351–2361 CrossRef CAS.
- A. Narumi, R. Rachi, H. Yamazaki, S. Kawaguchi, M. Kikuchi, H. Konno, T. Osaki, Y. Okamoto, X. Shen, T. Kakuchi, H. Kataoka, A. Nomoto, T. Yoshimura and S. Yano, ACS Omega, 2021, 6, 7023–7033 CrossRef CAS PubMed.
- R. Bonnett, R. D. White, U. J. Winfield and M. C. Berenbaum, Biochem. J., 1989, 261, 277–280 CrossRef CAS PubMed.
- X. Fu, Z. Yang, T. Deng, J. Chen, Y. Wen, X. Fu, L. Zhou, Z. Zhu and C. Yu, J. Mater. Chem. B, 2020, 8, 1481–1488 RSC.
- K. Valachová and L. Šoltés, Molecules, 2021, 26, 1195 CrossRef PubMed.
- M. Hassn Mesrati, S. E. Syafruddin, M. A. Mohtar and A. Syahir, Biomolecules, 2021, 11, 1850 CrossRef CAS PubMed.
- G. Mattheolabakis, L. Milane, A. Singh and M. M. Amiji, J. Drug Targeting, 2015, 23, 605–618 CrossRef CAS PubMed.
- G. Borland, J. A. Ross and K. Guy, Immunology, 1998, 93, 139–148 CrossRef CAS PubMed.
- P. Teriete, S. Banerji, M. Noble, C. D. Blundell, A. J. Wright, A. R. Pickford, E. Lowe, D. J. Mahoney, M. I. Tammi, J. D. Kahmann, I. D. Campbell, A. J. Day and D. G. Jackson, Mol. Cell, 2004, 13, 483–496 CrossRef CAS PubMed.
- D.-H. Wan, B.-Y. Zheng, M.-R. Ke, J.-Y. Duan, Y.-Q. Zheng, C.-K. Yeh and J.-D. Huang, Chem. Commun., 2017, 53, 4112–4115 RSC.
- D. Chen, M. Suo, J. Guo, W. Tang, W. Jiang, Y. Liu and Y. Duo, Adv. Healthcare Mater., 2021, 10, 2001577 CrossRef CAS PubMed.
- M. Benej, X. Hong, S. Vibhute, S. Scott, J. Wu, E. Graves, Q.-T. Le, A. C. Koong, A. J. Giaccia, B. Yu, C.-S. Chen, I. Papandreou and N. C. Denko, Proc. Natl. Acad. Sci. U. S. A., 2018, 115, 10756–10761 CrossRef CAS PubMed.
- S. Liu, H. Zhang, Y. Li, J. Liu, L. Du, M. Chen, R. T. K. Kwok, J. W. Y. Lam, D. L. Phillips and B. Z. Tang, Angew. Chem., Int. Ed., 2018, 57, 15189–15193 CrossRef CAS PubMed.
- J. Zou, Z. Yin, P. Wang, D. Chen, J. Shao, Q. Zhang, L. Sun, W. Huang and X. Dong, Chem. Sci., 2018, 9, 2188–2194 RSC.
- Y. Wang, L. Shi, D. Ma, S. Xu, W. Wu, L. Xu, M. Panahandeh-Fard, X. Zhu, B. Wang and B. Liu, ACS Nano, 2020, 14, 13056–13068 CrossRef CAS PubMed.
- L. Tang, X. Yang, Q. Yin, K. Cai, H. Wang, I. Chaudhury, C. Yao, Q. Zhou, M. Kwon, J. A. Hartman, I. T. Dobrucki, L. W. Dobrucki, L. B. Borst, S. Lezmi, W. G. Helferich, A. L. Ferguson, T. M. Fan and J. Cheng, Proc. Natl. Acad. Sci. U. S. A., 2014, 111, 15344–15349 CrossRef CAS PubMed.
- C.-Y. Shih, P.-T. Wang, W.-C. Su, H. Teng and W.-L. Huang, Biomedicines, 2021, 9, 137 CrossRef CAS PubMed.
- Z. Xie, S. Liang, X. Cai, B. Ding, S. Huang, Z. Hou, P. a. Ma, Z. Cheng and J. Lin, ACS Appl. Mater. Interfaces, 2019, 11, 31671–31680 CrossRef CAS PubMed.
- Q. Xu, G. Zhan, Z. Zhang, T. Yong, X. Yang and L. Gan, Theranostics, 2021, 11, 1937–1952 CrossRef CAS PubMed.
- Y. You, D. Xu, X. Pan and X. Ma, Appl. Mater. Today, 2019, 16, 508–517 CrossRef.
- Q. You, K. Zhang, J. Liu, C. Liu, H. Wang, M. Wang, S. Ye, H. Gao, L. Lv, C. Wang, L. Zhu and Y. Yang, Adv. Sci., 2020, 7, 1903341 CrossRef CAS PubMed.
- H. Cheng, J.-Y. Zhu, S.-Y. Li, J.-Y. Zeng, Q. Lei, K.-W. Chen, C. Zhang and X.-Z. Zhang, Adv. Funct. Mater., 2016, 26, 7847–7860 CrossRef CAS.
- Y. Wang, Z. Luan, C. Zhao, C. Bai and K. Yang, Eur. J. Pharm. Sci., 2020, 142, 105136 CrossRef CAS PubMed.
- X. Zhen, P. Cheng and K. Pu, Small, 2019, 15, e1804105 CrossRef PubMed.
- H.-J. Cai, T.-T. Shen, J. Zhang, C.-F. Shan, J.-G. Jia, X. Li, W.-S. Liu and Y. Tang, J. Mater. Chem. B, 2017, 5, 2390–2394 RSC.
- F. Wang and X. Liu, Chem. Soc. Rev., 2009, 38, 976–989 RSC.
- F. Auzel, Chem. Rev., 2004, 104, 139–174 CrossRef CAS PubMed.
- B. del Rosal and D. Jaque, Methods Appl. Fluoresc., 2019, 7, 022001 CrossRef CAS PubMed.
- Y. Huang, F. Qiu, R. Chen, D. Yan and X. Zhu, J. Mater. Chem. B, 2020, 8, 3772–3788 RSC.
- Y. Zou, S. Long, T. Xiong, X. Zhao, W. Sun, J. Du, J. Fan and X. Peng, ACS Cent. Sci., 2021, 7, 327–334 CrossRef CAS PubMed.
- Y.-C. Ma, Y.-H. Zhu, X.-F. Tang, L.-F. Hang, W. Jiang, M. Li, M. I. Khan, Y.-Z. You and Y.-C. Wang, Biomater. Sci., 2019, 7, 2740–2748 RSC.
- W. He, Y.-T. Zhou, W. G. Wamer, X. Hu, X. Wu, Z. Zheng, M. D. Boudreau and J.-J. Yin, Biomaterials, 2013, 34, 765–773 CrossRef CAS PubMed.
- J. R. Starkey, A. K. Rebane, M. A. Drobizhev, F. Meng, A. Gong, A. Elliott, K. McInnerney and C. W. Spangler, Clin. Cancer Res., 2008, 14, 6564–6573 CrossRef CAS PubMed.
- A. V. Kachynski, A. Pliss, A. N. Kuzmin, T. Y. Ohulchanskyy, A. Baev, J. Qu and P. N. Prasad, Nat. Photonics, 2014, 8, 455–461 CrossRef CAS.
- C. Zhang, K. Zhao, W. Bu, D. Ni, Y. Liu, J. Feng and J. Shi, Angew. Chem., Int. Ed., 2015, 54, 1770–1774 CrossRef CAS PubMed.
- D. Yang, G. Yang, S. Gai, F. He, C. Li and P. Yang, ACS Appl. Mater. Interfaces, 2017, 9, 6829–6838 CrossRef CAS PubMed.
- J. Liu, Y. Liu, N. Liu, Y. Han, X. Zhang, H. Huang, Y. Lifshitz, S. T. Lee, J. Zhong and Z. Kang, Science, 2015, 347, 970–974 CrossRef CAS PubMed.
- J. F. Algorri, M. Ochoa, P. Roldán-Varona, L. Rodríguez-Cobo and J. M. López-Higuera, Cancers, 2021, 13, 3484 CrossRef CAS PubMed.
- F. Bolze, S. Jenni, A. Sour and V. Heitz, Chem. Commun., 2017, 53, 12857–12877 RSC.
- Y. Ji, C. Jones, Y. Baek, G. K. Park, S. Kashiwagi and H. S. Choi, Adv. Drug Delivery Rev., 2020, 167, 121–134 CrossRef CAS PubMed.
- Q. Sun, H. Bi, Z. Wang, C. Li, C. Wang, J. Xu, D. Yang, F. He, S. Gai and P. Yang, ACS Appl. Mater. Interfaces, 2019, 11, 36347–36358 CrossRef CAS PubMed.
- P. Prasad, C. R. Gordijo, A. Z. Abbasi, A. Maeda, A. Ip, A. M. Rauth, R. S. DaCosta and X. Y. Wu, ACS Nano, 2014, 8, 3202–3212 CrossRef CAS PubMed.
- D. Wang, N. Zhang, X. Jing, Y. Zhang, Y. Xu and L. Meng, J. Mater. Chem. B, 2020, 8, 8271–8281 RSC.
- X. Ge, Q. Fu, L. Bai, B. Chen, R. Wang, S. Gao and J. Song, New J. Chem., 2019, 43, 8835–8851 RSC.
- A. B. E. Attia, G. Balasundaram, M. Moothanchery, U. S. Dinish, R. Bi, V. Ntziachristos and M. Olivo, Photoacoustics, 2019, 16, 100144 CrossRef PubMed.
- B. Qu, Y. Han, J. Li, Q. Wang, B. Zhao, X. Peng and R. Zhang, RSC Adv., 2021, 11, 5044–5054 RSC.
- C. Maihöfner, I. Diel, H. Tesch, T. Quandel and R. Baron, Support. Care Cancer, 2021, 29, 4223–4238 CrossRef PubMed.
- P. Smith, A. Lavery and R. C. Turkington, Best Pract. Res., Clin. Gastroenterol., 2020, 48–49, 101691 CrossRef PubMed.
- R. Chen, J. Zhang, Y. Wang, X. Chen, J. A. Zapien and C.-S. Lee, Nanoscale, 2015, 7, 17299–17305 RSC.
- L. Zhang, Y. Gao, S. Sun, Z. Li, A. Wu and L. Zeng, J. Mater. Chem. B, 2020, 8, 1739–1747 RSC.
- Y. Tao, M. Li, J. Ren and X. Qu, Chem. Soc. Rev., 2015, 44, 8636–8663 RSC.
- W. Zhang, S. Li, X. Liu, C. Yang, N. Hu, L. Dou, B. Zhao, Q. Zhang, Y. Suo and J. Wang, Adv. Funct. Mater., 2018, 28, 1706375 CrossRef.
- S.-Z. Ren, B. Wang, X.-H. Zhu, D. Zhu, M. Liu, S.-K. Li, Y.-S. Yang, Z.-C. Wang and H.-L. Zhu, ACS Appl. Mater. Interfaces, 2020, 12, 24662–24674 CrossRef CAS PubMed.
- H. Phan, V. Taresco, J. Penelle and B. Couturaud, Biomater. Sci., 2021, 9, 38–50 RSC.
- Z. Lei, Y. Ju, Y. Lin, X. Bai, W. Hu, Y. Wang, H. Luo and Z. Tong, ACS Appl. Bio Mater., 2019, 2, 3648–3658 CrossRef CAS PubMed.
- A. K. Jacob, R. S. Hotchkiss, S. L. DeMeester, M. Hiramatsu, I. E. Karl, P. E. Swanson, J. P. Cobb and T. G. Buchman, Surgery, 1997, 122, 243–254 CrossRef CAS PubMed.
- X. Feng, Z. Wang, Y. Chen, T. Tao, F. Wu and Y. Zuo, Ind. Eng. Chem. Res., 2012, 51, 7007–7012 CrossRef CAS.
- Y. Chen, Z. Liu, Z. Wang, M. Xue, X. Zhu and T. Tao, J. Hazard. Mater., 2011, 194, 202–208 CrossRef CAS PubMed.
- Y.-T. Qin, H. Peng, X.-W. He, W.-Y. Li and Y.-K. Zhang, ACS Appl. Mater. Interfaces, 2019, 11, 34268–34281 CrossRef CAS PubMed.
- A. DeCarlo, C. Malardier-Jugroot and M. R. Szewczuk, Bioconjugate Chem., 2021, 32, 512–522 CrossRef CAS PubMed.
- Y. J. Hou, X. X. Yang, R. Q. Liu, D. Zhao, C. X. Guo, A. C. Zhu, M. N. Wen, Z. Liu, G. F. Qu and H. X. Meng, Int. J. Nanomed., 2020, 15, 6827–6838 CrossRef CAS PubMed.
- S. Cui, D. Yin, Y. Chen, Y. Di, H. Chen, Y. Ma, S. Achilefu and Y. Gu, ACS Nano, 2013, 7, 676–688 CrossRef CAS PubMed.
- H. Kawasaki, S. Kumar, G. Li, C. Zeng, D. R. Kauffman, J. Yoshimoto, Y. Iwasaki and R. Jin, Chem. Mater., 2014, 26, 2777–2788 CrossRef CAS.
- D. Liu, J. Li, C. Wang, L. An, J. Lin, Q. Tian and S. Yang, Nanomedicine, 2021, 32, 102335 CrossRef CAS PubMed.
- J. Feng, W. Yu, Z. Xu and F. Wang, Chem. Sci., 2020, 11, 1649–1656 RSC.
- P. Yang, Y. Tian, Y. Men, R. Guo, H. Peng, Q. Jiang and W. Yang, ACS Appl. Mater. Interfaces, 2018, 10, 42039–42049 CrossRef CAS PubMed.
- B. Russell, J. Villaroel, K. Sapag and A. D. Migone, J. Phys. Chem. C, 2014, 118, 28603–28608 CrossRef CAS.
- W. Cheng, H. Zhang, D. Luan and X. W. Lou, Sci. Adv., 2021, 7, eabg2580 CrossRef CAS PubMed.
- P. Z. Moghadam, T. Islamoglu, S. Goswami, J. Exley, M. Fantham, C. F. Kaminski, R. Q. Snurr, O. K. Farha and D. Fairen-Jimenez, Nat. Commun., 2018, 9, 1378 CrossRef PubMed.
- J. B. DeCoste, M. H. Weston, P. E. Fuller, T. M. Tovar, G. W. Peterson, M. D. LeVan and O. K. Farha, Angew. Chem., Int. Ed. Engl., 2014, 53, 14092–14095 CrossRef CAS PubMed.
- C. Zhang, W. Bu, D. Ni, S. Zhang, Q. Li, Z. Yao, J. Zhang, H. Yao, Z. Wang and J. Shi, Angew. Chem., Int. Ed., 2016, 55, 2101–2106 CrossRef CAS PubMed.
- P. Kuppusamy, H. Li, G. Ilangovan, A. J. Cardounel, J. L. Zweier, K. Yamada, M. C. Krishna and J. B. Mitchell, Cancer Res., 2002, 62, 307–312 CAS.
- Y. Sun, X. Ma and H. Hu, Int. J. Mol. Sci., 2021, 22, 5698 CrossRef CAS PubMed.
- L.-H. Fu, C. Qi, J. Lin and P. Huang, Chem. Soc. Rev., 2018, 47, 6454–6472 RSC.
- C. Wei, Y. Liu, X. Zhu, X. Chen, Y. Zhou, G. Yuan, Y. Gong and J. Liu, Biomaterials, 2020, 238, 119848 CrossRef CAS PubMed.
- X. Wan, L. Song, W. Pan, H. Zhong, N. Li and B. Tang, ACS Nano, 2020, 14, 11017–11028 CrossRef CAS PubMed.
- Y. Ouyang, P. Wang, B. Huang, G. Yang, J. Tian and W. Zhang, ACS Appl. Bio Mater., 2021, 4, 4413–4421 CrossRef CAS PubMed.
- Y. Xue, J. Tian, Z. Liu, J. Chen, M. Wu, Y. Shen and W. Zhang, Biomacromolecules, 2019, 20, 2796–2808 CrossRef CAS PubMed.
- J. Mendelsohn, Clin. Cancer Res., 1997, 3, 2703–2707 CAS.
- H. He, Y. Chen, Y. Li, Z. Song, Y. Zhong, R. Zhu, J. Cheng and L. Yin, Adv. Funct. Mater., 2018, 28, 1706710 CrossRef.
- L. Ding, X. Lin, Z. Lin, Y. Wu, X. Liu, J. Liu, M. Wu, X. Zhang and Y. Zeng, ACS Appl. Mater. Interfaces, 2020, 12, 36906–36916 CrossRef CAS PubMed.
- K. Sinha, J. Das, P. B. Pal and P. C. Sil, Arch. Toxicol., 2013, 87, 1157–1180 CrossRef CAS PubMed.
- C. Roma-Rodrigues, L. Rivas-García, P. V. Baptista and A. R. Fernandes, Pharmaceutics, 2020, 12, 233 CrossRef CAS PubMed.
- P. R. Cullis and M. J. Hope, Mol. Ther., 2017, 25, 1467–1475 CrossRef CAS PubMed.
- H. Wang, Y. Chen, H. Wang, X. Liu, X. Zhou and F. Wang, Angew. Chem., Int. Ed., 2019, 58, 7380–7384 CrossRef CAS PubMed.
- D. A. Baum and S. K. Silverman, Cell. Mol. Life Sci., 2008, 65, 2156–2174 CrossRef CAS PubMed.
- H. Fan, X. Zhang and Y. Lu, Sci. China: Chem., 2017, 60, 591–601 CrossRef CAS.
- A. J. Amali, H. Hoshino, C. Wu, M. Ando and Q. Xu, Chemistry, 2014, 20, 8279–8282 CrossRef CAS PubMed.
- D. Wang, D. Jana and Y. Zhao, Acc. Chem. Res., 2020, 53, 1389–1400 CrossRef CAS PubMed.
- C. Sui, R. Tan, Y. Chen, G. Yin, Z. Wang, W. Xu and X. Li, Bioconjugate Chem., 2021, 32, 318–327 CrossRef CAS PubMed.
- S. Wang, L. Shang, L. Li, Y. Yu, C. Chi, K. Wang, J. Zhang, R. Shi, H. Shen, G. I. Waterhouse, S. Liu, J. Tian, T. Zhang and H. Liu, Adv. Mater., 2016, 28, 8379–8387 CrossRef CAS PubMed.
- Q. Zhang, J. He, W. Yu, Y. Li, Z. Liu, B. Zhou and Y. Liu, RSC Med. Chem., 2020, 11, 427–437 RSC.
- L. Zhang, Z. Yang, W. He, J. Ren and C.-Y. Wong, J. Colloid Interface Sci., 2021, 599, 543–555 CrossRef CAS PubMed.
- B. Wu, J. Fu, Y. Zhou, S. Luo, Y. Zhao, G. Quan, X. Pan and C. Wu, Acta Pharm. Sin. B, 2020, 10, 2198–2211 CrossRef CAS PubMed.
- M. Ma, A. Bétard, I. Weber, N. S. Al-Hokbany, R. A. Fischer and N. Metzler-Nolte, Cryst. Growth Des., 2013, 13, 2286–2291 CrossRef CAS.
- C. Mellot-Draznieks, C. Serre, S. Surblé, N. Audebrand and G. Férey, J. Am. Chem. Soc., 2005, 127, 16273–16278 CrossRef CAS PubMed.
- X. Sun, G. He, C. Xiong, C. Wang, X. Lian, L. Hu, Z. Li, S. J. Dalgarno, Y.-W. Yang and J. Tian, ACS Appl. Mater. Interfaces, 2021, 13, 3679–3693 CrossRef CAS PubMed.
- J.-C. Yang, Y. Shang, Y.-H. Li, Y. Cui and X.-B. Yin, Chem. Sci., 2018, 9, 7210–7217 RSC.
- G. K. Gupta and D. K. Agrawal, BioDrugs, 2010, 24, 225–235 CrossRef CAS PubMed.
- G. Turgeon, A. Weickhardt, A. Azad, B. Solomon and S. Siva, Med. J. Aust., 2019, 210, 47–53 CrossRef PubMed.
- W.-D. Yu, G. Sun, J. Li, J. Xu and X. Wang, Cancer Lett., 2019, 452, 66–70 CrossRef CAS PubMed.
- R. Xie, P. Yang, S. Peng, Y. Cao, X. Yao, S. Guo and W. Yang, J. Mater. Chem. B, 2020, 8, 6128–6138 RSC.
- M. P. Cuajungco, M. S. Ramirez and M. E. Tolmasky, Biomedicines, 2021, 9, 208 CrossRef CAS PubMed.
- P. Chen, M. He, B. Chen and B. Hu, Ecotoxicol. Environ. Saf., 2020, 205, 111110 CrossRef CAS PubMed.
|
This journal is © The Royal Society of Chemistry 2022 |
Click here to see how this site uses Cookies. View our privacy policy here.