DOI:
10.1039/D2RA00657J
(Review Article)
RSC Adv., 2022,
12, 9373-9394
A minireview on the synthesis of single atom catalysts
Received
31st January 2022
, Accepted 14th March 2022
First published on 24th March 2022
Abstract
Single atom catalysis is a prosperous and rapidly growing research field, owing to the remarkable advantages of single atom catalysts (SACs), such as maximized atom utilization efficiency, tailorable catalytic activities as well as supremely high catalytic selectivity. Synthesis approaches play crucial roles in determining the properties and performance of SACs. Over the past few years, versatile methods have been adopted to synthesize SACs. Herein, we give a thorough and up-to-date review on the progress of approaches for the synthesis of SACs, outline the general principles and list the advantages and disadvantages of each synthesis approach, with the aim to give the readers a clear picture and inspire more studies to exploit novel approaches to synthesize SACs effectively.
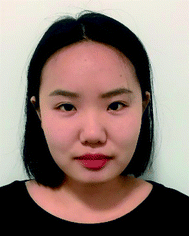 Jiawen Guo | Jiawen Guo graduated from Liaoning University of Technology with a bachelor's degree in 2021. She is currently studying for a master's degree in Liaoning University of Technology, with a specialty of catalysis design. |
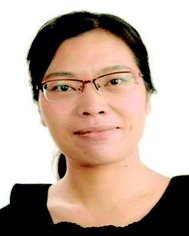 Huimin Liu | Prof. Huimin Liu received her PhD degree from Tsinghua University, China (2013), and then joined Kansai University (2013–2014), National Institute of Materials Science (NIMS, 2014–2017) and the University of Sydney (2017–2019) as post-doctoral researcher and Lecturer. Now she is working in Liaoning University of Technology as a professor. Her research interests are photochemistry, environmental chemistry and heterogeneous catalyst design. |
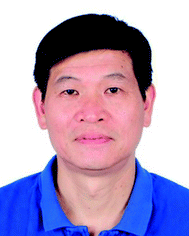 Dehua He | Prof. Dehua He received his PhD degree from Tokyo Institute of Technology, Japan (2013), and then joined Sagami Chemical Research Center (1991–1994) and Japan Institute of Earth Environmental Industry Technology (1999–2000) as guest researcher. He was a visiting scholar at the University of Virginia in 2003. Now he is working at Tsinghua University as a professor. His research interests are heterogeneous catalyst and homogeneous catalysis design. |
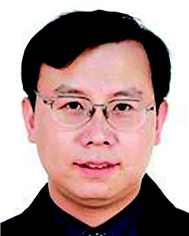 Qijian Zhang | Prof. Qijian Zhang received his PhD degree from Tsinghua University, China (1990), and then joined Kitakyushu University (2003–2005) as post-doctoral researcher. Now he is working in Liaoning University of Technology as the director of science and technology division. His research interests are hydrogen production from dimethyl ether reforming, photothermal catalytic reaction, etc. |
1. Introduction
Single atom catalysis describes a process in which a single atom on a catalyst surface drives a catalytic reaction.1,2 The catalyst with a single atom on its surface is called a single atom catalyst (SAC). The concept of SAC was raised up by Zhang et al. in 2011, based on a study that a catalyst with Pt atoms singly dispersed on FeOx (named as Pt1/FeOx) was much superior in CO oxidation reaction than its nanoparticle counterpart.3 The individually dispersed active sites on SACs can ① tune electronic states, which directly or indirectly regulates the catalytic activity, and ② minimize the number of binding configuration choices between reactants and SACs, which consequently leads to an extremely high selectivity towards the target product.4–9
Initiated by this seminal work, the nomenclature of SACs (M1/Support) has become widely accepted, which sparked researchers' interest and boosted extensive studies.10–20 It has been proved that synthesis approaches play crucial roles in determining the properties of SACs, such as crystallinity, composition, morphology, pore structure and valence states, which eventually contributes to the performance of SACs.
Over the past few years, significant efforts have been devoted to the synthesis of SACs. To the best of our knowledge, tens of methods have been adopted to synthesize SACs. In this review, we summarize the scientific achievements of synthesis approaches of SACs to the catalysis community, aiming at providing a clear picture and inspiring more studies to exploit novel and effective approaches to synthesize SACs.
2. Synthesis approaches of SACs
In this section, we divide the synthesis approaches into dry chemistry route and wet chemistry route (Scheme 1), review the general principles of the approaches to synthesize SACs and compare the advantages and disadvantages of each synthesis approach.
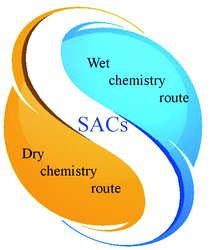 |
| Scheme 1 Classification of the synthesis approaches of SACs. | |
2.1 Dry chemistry routes
2.1.1 Atomic layer deposition (ALD) method. ALD method also referred as atomic layer epitaxy. It was originally proposed by Finnish scientists in 1970s and developed for the preparation of polycrystalline fluorescent materials ZnS
:
Mn21–28 and amorphous Al2O3 insulating film,29–31 and recently it has been successfully used for the preparation of SACs.An ALD reactor is indispensable for the synthesis of SACs via ALD method. The schematic illustration of a typical ALD reactor is displayed in Fig. 1. Its core is a chamber consisting of a static or rotary bed of substrates. The precursor vapor is pulsed into the chamber, where it diffuses and permeates to reach and coat the substrate surfaces.
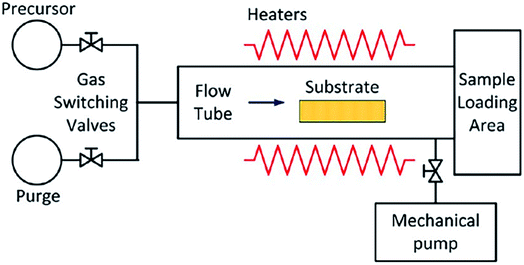 |
| Fig. 1 Schematic illustration of an ALD reactor. This figure has been adapted/reproduced from ref. 32 with permission from American Chemical Society, copyright 2021. | |
The most common mechanism for synthesizing SACs via ALD method is the ligand exchange reactions, in which the ligands or functional groups on the substrate react with the precursor. When ALD method is used to synthesize SACs, catalyst support is the substrate placed in the chamber, into which the pulse stream of precursor containing the atomically dispersed active component diffuses and reacts with the ligands or functional groups on support surface (the schematic illustration is displayed in Fig. 2).33–40 The stream of precursor is stopped when the reaction between the precursor and the substrate completes. The residuals of the precursor vapor and byproducts are purged with an inert gas or evacuated at high vacuum. These two steps are called the first half-reaction. After the first half-reaction, one ALD cycle is finished. If multiple ALD cycles are needed, a second stream of precursor is pulsed into the chamber to regenerate the ligands or functional groups on support surface. After purging to remove the residuals and byproducts, it is ready for next ALD cycle (the second half-reaction in Fig. 2).
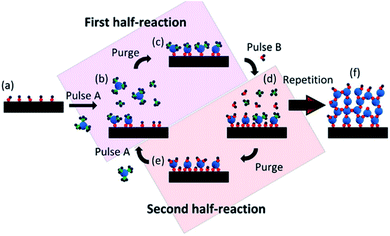 |
| Fig. 2 Schematic illustration of the preparation of SACs by dosing two distinct precursors sequentially to the matrix. This figure has been adapted/reproduced from ref. 32 with permission from American Chemical Society, copyright 2021. | |
According to the mechanism for the synthesis of SACs via ALD method, ligands or functional groups on support are necessary. Carbon, especially graphene, has been proved as excellent candidate support for anchoring metal atoms separately due to its unique electronic and structural properties, which facilitate the bonding between the singly dispersed atoms and carbon support through Metal–C and Metal–O–C coordination.41 Using the ALD method, a number of graphene supported SACs, such as Pt1/graphene, Pd1/graphene and Co1/graphene, have been successfully prepared.42–48
Here the synthesis of Co1/SiO2 is taken as an example.36 Gorte et al. utilized tris (2,2,6,6-tetramethyl-3,5-heptanedionato) cobalt(III) as ALD precursor, 0.7 g SiO2 were evacuated and exposed to 5 Torr of precursor vapor at 250 °C for 5 min, after removing the excessive precursors via evacuation and removing the ligands via oxidizing it in a muffle furnace at 500 °C for 7 min, one ALD cycle is finished and Co1/SiO2 is obtained. The number of singly dispersed atoms on SACs could be controlled by adjusting the number of ALD cycles. Gorte et al. further investigated the effects of the number of ALD cycles on the properties of the as-prepared catalysts. They discovered that isolated Co catalyst could be obtained with only one ALD cycle. Co loadings increased linearly with the number of ALD cycles. After several cycles, the single dispersion state of Co remained. Further increasing the number of ALD cycles, Co gradually transformed from the single dispersion state to clusters and eventually to nanoparticles.36
ALD possesses the following advantages, (1) the deposition parameters could be controlled precisely, (2) the desired thickness or size of the deposited precursor could be achieved by adjusting the number of cycles, (3) the deposition uniformity and reproducibility are excellent in spite that slight deviations from the ideal conditions are sometimes observed, and (4) different deposition materials can be controlled precisely at the atomic level to form composites with various morphologies.31,49–54 The advantages of ALD make it possible for providing ideal model catalysts for the basic studies of catalysis, which include exploring the effects of particle sizes, surface properties of catalyst supports and encapsulation (or surface coating) of metal or alloy nanoparticles, on the catalytic performance. In conclusion, ALD is an important approach to synthesize SACs and study the structure–activity relationship over SACs. The drawback is that, only materials with suitable ligands or functional groups could be chosen as support to disperse the metal atoms separately via ALD method, meanwhile, this technology is not applicable for the commercial preparation of SACs currently, because of the stability and cost issues (Table 1).
Table 1 The metals used in the synthesis of SACs by ALD
Method |
Singly dispersed metals |
ALD |
Pt, Pd, Co, Cu, Fe, Ni, Ti, Zn32 |
2.1.2 Pyrolysis synthesis method. Pyrolysis synthesis, as the name suggested, is a strategy to synthesize SACs by thermochemically decomposing the organic precursors of the catalysts at elevated temperatures under a specific atmosphere. A series of metal–nitrogen–carbon SACs have been successfully synthesized via this method in the past decade.55–76At present, the precursors for the preparation of SACs by this method are mainly divided into metal–organic frameworks (MOFs), metal phthalocyanine–silica colloid composites, metal–phenanthroline complexes on carbon supports and amino-functionalized rigid molecules. Here, several examples are elaborated to illustrate the preparation procedures for SACs via the pyrolysis of the several above-mentioned precursors. (1) For the preparation of stable Co–nitrogen–carbon SAC, bimetallic Zn/Co MOF is an excellent precursor. During the following pyrolysis process, Zn could be selectively evaporated away at high temperatures above 800 °C and Co could be reduced by the carbonization of the organic linker. The loading of singly dispersed Co atoms achieved via this method could be high up to 4 wt%.77 Other metal–nitrogen–carbon SACs (M = W, Mo, Cu, Zn, Cr, Mn, Fe and Ni) could also be obtained via the pyrolysis synthesis method with bimetallic-organic frameworks as precursors.78–83 (2) When Co phthalocyanine–silica colloid composite was used as precursor, singly dispersed Co catalyst Co–Nx/C could be prepared by the pyrolysis of the precursor and the subsequent removal of silica template and cobalt nanoparticles.84 The as prepared Co–Nx/C SAC showed extremely high activity, chemoselectivity and stability toward the reduction of nitro compounds by H2. (3) The noble-metal-free Co–N–C catalyst could also be derived from the pyrolysis of cobalt–phenanthroline complexes on a mesoporous carbon support at high temperatures,85 and this Co–N–C catalyst was reported to show high catalytic activity (turnover frequency, TOF, of 3.8 s−1 based on Co single atoms) and good recyclability in the aerobic oxidation of over 28 examples of diverse substrates. (4) The preparation of Pd1/N-graphene from amino-functionalized rigid molecules is another example for pyrolysis synthesis method. In the preparation procedure, a precursor was firstly obtained by functionalizing the palladium phthalocyanines with four aminophenoxy groups at the periphery of the benzene ring of graphene, which was then pyrolyzed to anchor the isolated Pd atoms.86
Taking the Co–N–C catalyst derived from the pyrolysis of cobalt–phenanthroline complexes on a mesoporous carbon support and Pd1/N-graphene prepared from amino-functionalized rigid molecules as typical examples, high angle annular dark field scanning transmission electron microscopy (HAADF-STEM) images clearly indicated that the precursors were successfully transformed into singly dispersed atoms (Fig. 3a–d).85,86
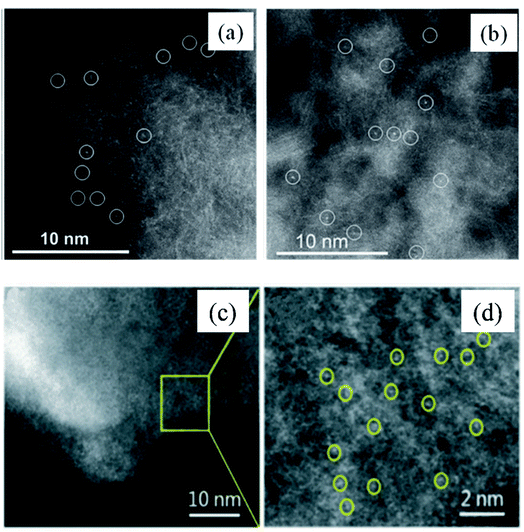 |
| Fig. 3 HAADF-STEM image of Co–N–C catalyst prepared by the pyrolysis synthesis method (a and b) and Pd1/N-graphene catalyst prepared by the pyrolysis synthesis method (c and d). These figures have been adapted/reproduced from ref. 85 and 86 with permission from American Chemical Society and Royal Society of Chemistry, copyright 2015 and 2022. | |
The pyrolysis synthesis method is a straightforward route for the preparation of SACs. The singly dispersed atoms could be derived from precursors, in which there are suitable interactions between the metal precursor and support. Generally, the singly dispersed atoms are transitional metals, such as W, Mo, Cu, Zn, Cr, Mn, Fe and Ni, while the supports are originated from MOFs, silica colloids, carbon or amino-functionalized rigid molecules. Upon pyrolysis in a furnace under a specific atmosphere, the precursors gradually decompose, lead to the transformation of precursor to the desired support and single atoms. However, high pyrolysis temperatures are generally required, which makes it an energy intensive process. In addition, up to now, there have been no reports on the synthesis of non-metal-based SACs via this method (Table 2).
Table 2 Summary of precursors and metals for pyrolysis synthesis of SACs
Method |
Precursors |
Single-atom metals |
Pyrolysis synthesis |
MOFs |
W, Mo, Cu, Zn, Cr, Mn, Fe, Ni78–83 |
Silica colloids |
Carbon or amino-functionalized rigid molecules77 |
2.1.3 Atom trapping method. Atom trapping method is a promising approach to synthesize singly dispersed catalysts by utilizing the migration property of metal atoms at elevated temperatures. It was initially adopted for the synthesis of SACs in 2016 by Jones et al., who reported that a thermally stable single atom Pt1/CeO2 catalyst was successfully achieved by aging a mixture of Pt/Al2O3 and CeO2 in air at 800 °C to promote the transfer of Pt from Al2O3 to CeO2, where it was strongly anchored as single site (Fig. 4).87 CeO2 nanorods were more effective than CeO2 cubes at trapping the Pt atoms. Aging at high temperatures ensured that only the most stable binding sites on CeO2 were occupied, and thus yielded a sintering-resistant atomically dispersed catalyst.87 The transformation of nanoparticles to stable single atoms could be directly observed via HAADF-STEM. Fig. 5a–d displayed the evolution of Pd nanoparticles on zinc 2-methylimidazole metal organic framework (ZIF-8) to single atoms. Fig. 5a was the HAADF-STEM image of ZIF-8. During the first stage of heating, the crystalline Pd nanoparticles became larger and the size distribution became inhomogeneous (Intermediate I, Fig. 5b). Upon heating the second stage, the number of nanoparticles was significantly reduced, and the crystalline Pd nanoparticles were transformed into an amorphous state (Intermediate II, Fig. 5c). With the further extension of heating time, the remaining nanoparticles were fully digested within the substrate. At the same time, ZIF-8 was decomposed and converted into SACs (Fig. 5d).88
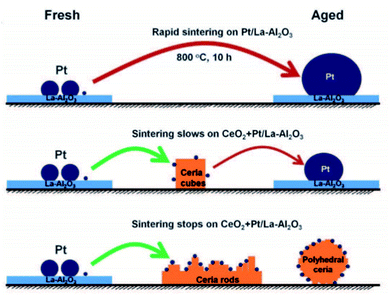 |
| Fig. 4 Illustration of the synthesis of Pt1/CeO2 SAC via the trapping of mobile Pt by CeO2 due to the migration of Pt at high temperatures. This figure has been adapted/reproduced from ref. 87 with permission from American Association for the Advancement of Science, copyright 2016. | |
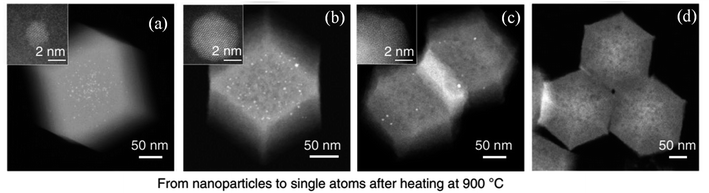 |
| Fig. 5 Evolution of Pd nanoparticles on ZIF-8 to single atoms via HAADF-STEM. (a) Pd nanoparticles on ZIF-8, (b) intermediate I, (c) intermediate II and (d) Pd single atoms. This figure has been adapted/reproduced from ref. 88 with permission from Nature Publishing Group, copyright 2018. | |
The atom trapping method is applicable to synthesize a series of SACs, including Pd,88 Au,88 Cu89 and Ni90 SACs. These SACs could be synthesized by transforming the relevant nanoparticles in an inert atmosphere above 900 °C, when nitrogen-doped carbon is used as support to capture the mobile atoms.88
The atom trapping method is a simple approach for synthesizing SACs. Nevertheless, the approach requires a supply of mobile atoms and a support that can trap the mobile species. Additionally, the very high synthesis temperatures are not favorable from an energy perspective. However, if the catalyst preparation process is applied to high-speed moving automobile exhaust, its temperature can be easily reached under operation, which makes this method very practical (Table 3).
Table 3 Summary of metals used in the synthesis of SACs by atomic trapping
Method |
Synthesis of single-atom metals |
Atom trapping |
Pt,87 Ru,87 Co,87 Pd,88 Au,88 Cu,89 Ni90 |
2.1.4 Two-step doping method. Two-step process is effective for doping metal elements atomically into graphene. It is carried out via two-steps. Firstly, vacancies are created via high energy atom/ion bombardment; then, the vacancies are filled with desired dopants (Fig. 6). Several different elements (including Pt, Co, and In) have been introduced as dopants in the single atom form into graphene via the two-step doping method.91,92
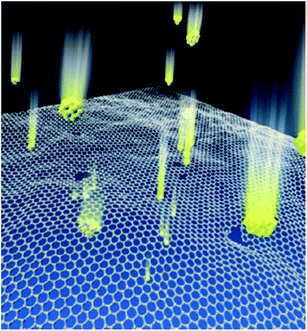 |
| Fig. 6 Schematic illustration of the high-energy particle bombardment process. This figure has been adapted/reproduced from ref. 91 and 92 with permission from American Chemical Society, copyright 2012 and 2015. | |
Two-step doping method takes advantages of the easy creation of vacancies on support and the high binding energies between the dopant and the vacancies. To the best of our knowledge, the support of SACs prepared by the two-step doping method is strictly limited to graphene, while the non-dependence on the type of dopants endows wider applications. The catalysts prepared by this method are highly stable due to the high binding energy between the dopant metal and the vacancy; however, high energy atom/ion generator is required to create vacancies if SACs are synthesized through this method (Table 4).
Table 4 Summary of metals used in the synthesis of SACs by two-step doping
Method |
Synthesis of single-atom metals |
Two-step doping |
Pt, Co, In,91,92 Fe,93 Cu,93 Ni93 |
2.1.5 Ball-milling method. As a mechanochemical technique, ball milling method takes advantages of mechanical interactions to convert reactants into products during the reaction process. It plays an important role in synthetic organic chemistry. For example, Gan et al. used a simple, green and scalable ball milling method to synthesize a cobalt alloy Pt SAC (Pt1/Co) in a kilogram-scale. It is reported that the as-prepared Pt1/Co catalyst gave excellent performance in the hydrodeoxygenation of 5-hydroxymethylfurfural.94 The HAADF-STEM image clearly revealed that the platinum atoms in the Pt1/Co catalyst were finely dispersed on the Co sup5port without obvious Pt aggregation (Fig. 7a), indicating that the ball milling method can realize the scale-up production of SACs.
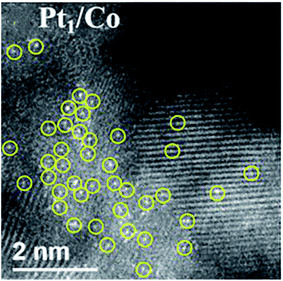 |
| Fig. 7 HAADF-STEM image of Pt1/Co. This figure has been adapted/reproduced from ref. 94 with permission from American Chemical Society, copyright 2020. | |
This work enables kilogram-scale production of Pt1/Co without scale-up effects, with great potential for practical applications. It provides a facile and scalable method for the synthesis of single-atom alloy catalysts.95
2.2 Wet chemistry routes
2.2.1 Methods taking advantages of the adsorption between single atom precursor and support.
2.2.1.1 Facile adsorption method. Facile adsorption method, as the name indicates, the singly dispersed active component is anchored onto the catalyst support via a simple adsorption mode. Zhang et al. used the facile adsorption method to synthesize Au1/FeOx catalyst.96 It was reported that, over Au1/FeOx catalyst, Au single atoms were separately dispersed on iron oxide crystallites,96 and the Au atoms were positively charged and anchored covalently to FeOx.96 The as-prepared Au1/FeOx catalyst exhibited ultrahigh stability and remarkable catalytic performance for CO oxidation at a wide temperature range.96The facile adsorption method is an approach to synthesis SACs utilizing the interactions between the active metal element and the support. It is easy and simple to operate, and could be achieved in a common chemistry lab. However, the metals are generally randomly absorbed on the surface of support, therefore, appropriate interaction between the active metal component and catalyst support is required to synthesize SACs successfully. In addition, due to the heterogeneity of support, the dispersion state of the active metal elements could not be well controlled.
2.2.1.2 Strong electrostatic adsorption method. The surface of oxide materials is often covered with a layer of hydroxyl groups. The pH at which the aqueous solution is neutral is termed the point of zero charge (PZC). The oxides are positively charged in aqueous solutions when pH values are below its isoelectric point and negatively charged at the cases when pH values are above its isoelectric point. By adjusting pH values, a monolayer of O−, OH or OH2+ could be formed on the surface of oxides. They are capable of anchoring various metal ion complexes via strong electrostatic interaction. Taking Pt salt as an example, the metal ion complexes are [(NH3)4Pt]2+, [H]+ or [PtCl6]2− (Fig. 8). SACs could be obtained after carefully removing the ligands of the complexes via post-treatment.97
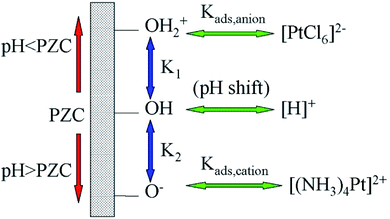 |
| Fig. 8 Mechanism of strong electrostatic adsorption method in the preparation of SACs. This figure has been adapted/reproduced from ref. 98 with permission from American Chemical Society, copyright 2008. | |
It is expected that pH, ionic strength as well as the properties of the metal ion complexes play critical roles on the properties of catalysts prepared by the strong electrostatic adsorption method.98–100 Morales-Garcia et al. computationally investigated the interaction between the singly dispersed Pd atoms and silicalite-1 as well as that between the Pdn clusters and silicalite-1.101 The interaction between a single Pd atom and silicalite-1 was strong with dominant contribution coming from the electrostatic effects, which resulted in a preferable binding of the isolated Pd atoms to the silanol groups on the external surface of silicalite-1.99 On the other hand, the interaction between Pd clusters and silicalite-1 was mainly dispersion interactions, with only a small contribution from electrostatic adsorption, which made Pd clusters preferably located inside the channels of silicalite-1. Pillay et al. adopted density functional theory (DFT) study to simulate the grafting of sulfur single atom onto Pt(111) and Pt3Ni(111) surfaces via the strong electrostatic adsorption method.102,103 From both the experimental and theoretical reports, it was found that the potential energy surfaces, appropriate functionalization of the support surface and the utilization of metal ion complexes with strong electrostatic interaction to the support, are key factors for the successful preparation of stable SACs.102,103
The strong electrostatic adsorption method is discovered to be effective for preparing high quality SACs, particularly for the preparation of singly dispersed noble metal catalysts. However, the adsorption behavior of metal complexes is influenced greatly by the heterogeneity of functional groups and the presence of various defects on the surface of the oxide matrix. In addition, the pH values of the aqueous solution change with the prolonging of the adsorption time, which might further affect the quality of the resulting SACs.
2.2.1.3 Wetness impregnation method. The wetness impregnation method is one of the typical preparation methods for traditional heterogeneous catalysts. In this approach, an aqueous or organic metal salt solution impregnates the catalyst, with the metal salt adsorbing on support surface. The residual solvent is removed, commonly by evaporation, to obtain the salt impregnated catalyst. The impregnation process relies greatly on the adsorption capacity of the surface of the support towards the organometallic complexes or inorganic salts.104–106 Therefore, the metal salt–support interaction is critical, and significantly influences the amount of metal loading and the dispersion of the metal anchored on the support surface.When an oxide is employed as the catalyst support and impregnated with an aqueous solution, polarization occurs similarly to that for the strong electrostatic method. In an acidic solution, the surface adsorption sites on the support (M–OH) are positively charged and attract anions. In the alkaline solution, the surface adsorption sites (M–OH) are negatively charged and attract cations. According to the Brunelle adsorption model, the key parameters for controlling the metal dispersion include (1) the type and concentration of the metal salt, (2) the pH value of the aqueous solution, and (3) the type of catalyst support and the functional groups on its surface.
The Al atom in γ-Al2O3 is hexa-coordinated and exhibits a penta-coordinated structure after calcination at high temperatures, resulting from the dehydration and removal of hydroxyl groups on the surface of γ-Al2O3 and leading to the generation of unsaturated Al sites.106 By utilizing these unsaturated Al sites on γ-Al2O3, which possess the capacity to stabilize single Pt atoms, Kwak et al. successfully prepared a single atom Pt1/γ-Al2O3 catalyst by impregnating γ-Al2O3 with an aqueous solution of Pt salt.107 After introducing the singly dispersed Pt atoms on γ-Al2O3 by the impregnation method, the number of penta-coordinated Al atoms was significantly reduced. With the increase of Pt loading, the penta-coordinated Al atom sites were not sufficient to stabilize all Pt single atoms, thus resulting in the formation of larger Pt particles.107 The HAADF-STEM images of 1 wt% Pt/γ-Al2O3 revealed that most of the Pt species were atomically dispersed and the HAADF-STEM images of 10 wt% Pt/γ-Al2O3 demonstrated the existence of both atomically dispersed Pt and Pt cluster/nanoparticles (Fig. 9).105
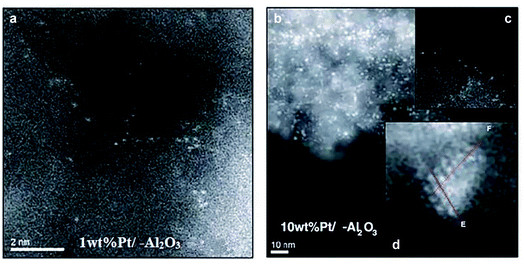 |
| Fig. 9 HAADF-STEM images of (a) 1 wt% Pt/γ-Al2O3 and (b) 10 wt% Pt/γ-Al2O3. The insets in Fig. 8b showed the presence of (c) atomically dispersed Pt and (d) Pt cluster/nanoparticles. This figure has been adapted/reproduced from ref. 107 with permission from American Association for the Advancement of Science, copyright 2009. | |
The wetness impregnation method is simple to operate, but it is difficult to ensure uniform dispersion of the metal atoms on the surface of the support and it is not suitable for the preparation of SACs with high metal loadings.108,109
2.2.2 Methods taking advantages of the photochemical and electrochemical properties of single atom precursors/support.
2.2.2.1 Photoreduction method. Photoreduction is a method to prepare SACs by reducing the metal salts into their metallic states with light as the driving force. Zheng et al.'s work in Science is one of the presentative studies on adopting the photoreduction method to synthesize SACs.110 The authors used photochemical reduction assisted wet chemical method to prepare Pd1/TiO2. The surface of the TiO2 film was positively charged and attracted a large amount of [PdCl4]2− anions, which enriched on the surface of the TiO2 sheet by carefully controlling the pH of the solution. Extended X-ray absorption fine structure (EXAFS) analysis (Fig. 10a) and HAADF-STEM image (Fig. 10b) suggested that a single atom Pd catalyst with a loading high up to 1.5 wt% could be obtained by UV light irradiation. Pd1/TiO2 could activate hydrogen via a pathway different from those over traditional heterogeneous catalysts, and exhibited a good activity for the hydrogenation of C
C and C
O bonds (Fig. 10c and d).
 |
| Fig. 10 (a) EXAFS analysis, (b) HAADF-STEM image as well as (c and d) catalytic performance of 1.5 wt%Pd/TiO2 catalyst in styrene hydrogenation reaction. This figure has been adapted/reproduced from ref. 110 with permission from American Association for the Advancement of Science, copyright 2016. | |
On one hand, no special equipment is needed for the photoreduction method, so it is easy and can be achieved in conventional chemical laboratories. On the other hand, the catalytic active sites of the catalysts prepared by this method generally are not uniform, because of the co-existing of singly dispersed sites and continuously packed sites (Table 5).
Table 5 Summary of metals used in the synthesis of SACs by photoreduction
Method |
Synthesis of single-atom metals |
Photoreduction |
Pt, Pd, Au, Co, Ni111 |
2.2.2.2 Galvanic replacement method. Galvanic replacement is a technique which takes the advantage of the reduction potential differences between a metal in a template and metal ions in a solution.112,113 Given proper potential differences, the galvanic replacement reactions could occur spontaneously, with the metal atoms in the template being oxidized and dissolved into the solution while the metal ions in the solution being reduced and plated on the surface of the metal template (Fig. 11). The galvanic replacement method has been successfully adopted in the synthesis of atomic layer deposited PtRu,114,115 PdCu,116–118 PdAu,119–121 PtPd,122–124 and Hg(1−x)CdxTe.125
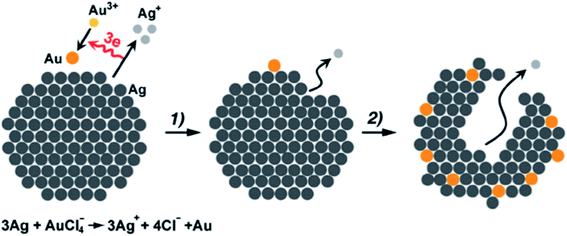 |
| Fig. 11 Schematic illustration of the galvanic replacement process (here the galvanic replacement of Ag by Au is shown as an example). This figure has been adapted/reproduced from ref. 126 with permission from Wiley-Blackwell, copyright 2013. | |
In occasional cases, to protect the intact structure of the template metal, a ternary metal might be introduced as sacrificial metal template for the galvanic replacement method. For example, to deposit atomic monolayer Pt on polycrystalline Au, a layer of Ni could be pre-electrodeposited on Au, which serves as the sacrificial metal template in the following galvanic replacement reaction. After exposing the resultant Ni-rich surface to Pt solution, Pt atomic monolayer is controllably grown on Au by galvanically replacing Ni by Pt.127 The utilization of sacrificial metal template offers an environmentally friendly and cost-effective approach for adopting the galvanic replacement method in atomic layer metal synthesis.
This galvanic replacement method is simple, versatile and could be used to synthesize SACs with a wide variety of metal templates and metal ions in the solution. At the same time, it is limited by the requirement of an appropriate difference in electrochemical potentials between the two metals (Table 6).
Table 6 Summary of metals used in the synthesis of SACs by galvanic replacement method
Method |
Synthesis of single-atom metals |
Galvanic replacement |
Pt, Ru,114,115 Pd, Cu, Au,116,121 Ag128 |
2.2.2.3 Cyclic voltammetry (CV) method. CV method is an approach to synthesize SACs in an electrochemical working station equipped with counter electrode (CE), reference electrode (RE) and a working electrode (WE). In case that CE is a Pt foil, upon potential cycling operation, trace amount of Pt on CE dissolves and is atomically deposited on RE. By assuming RE as a support, singly dispersed Pt catalyst is achieved (Schematic illustration is shown in Fig. 12). Luo et al.'s work is a typical example. They used a saturated calomel electrode as RE, a Ni foam (NF) with CoP-based nanorod/nanosheet arrays on its surface as WE and phosphate as butter solution, and successfully synthesized Pt1/CoP/NF catalyst via CV method.129 Pd1–N8/CNT, a N8− Lewis base catalyst, was synthesized via a similar procedure, where Pd foil is used as CE.130
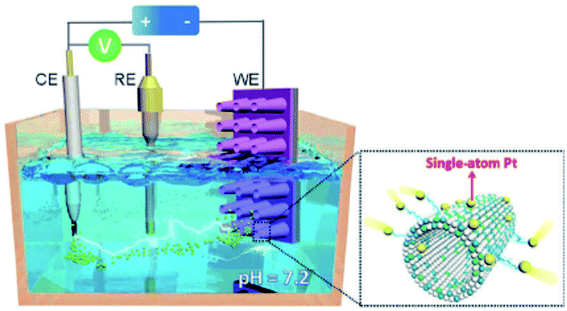 |
| Fig. 12 Schematic illustration for the synthesis of Pt SAC via CV method. This figure has been adapted/reproduced from ref. 129 with permission from John Wiley and Sons Ltd, copyright 2017. | |
In CV method, less additives are demanded and no interfering products are generated, which make it easily scalable. However, an electrochemical station is needed, indicating the operation cost is high.
2.2.3 Other wet chemistry routes to synthesize SACs.
2.2.3.1 Ion exchange method. The ion exchange method is an approach for introducing a foreign precursor from aqueous solution onto the support in a controlled way. The term ion exchange has been adopted to describe all processes where ionic species from aqueous solution exchange with the charged sites on the support.131 This approach has been commonly used for the preparation of catalysts with easily exchangeable ions on supports, such as those in zeolites, heteropoly acids, or MOF, but has only recently been applied for the synthesis of SACs.132,133A representative example of SACs prepared by the ion exchange method was demonstrated by Zhang et al., who utilized ion exchange followed by NaBH4 reduction method to synthesize ion exchange resin supported AuPd alloy SAC.134 With the increase of Au/Pd molar ratio, the continuous Pd ensembles on the ion exchange resin surface were gradually separated and eventually isolated by Au atoms, forming the Au alloyed Pd SAC (evidenced by the appearance of CO adsorption peaks on diffuse reflectance infrared Fourier transform spectroscopy (DRIFT) spectra shown in Fig. 13).This catalyst was active without leaching for the Ullmann reaction of aryl halides under mild conditions in aqueous media, particularly effective for the activation of less reactive aryl chlorides.134
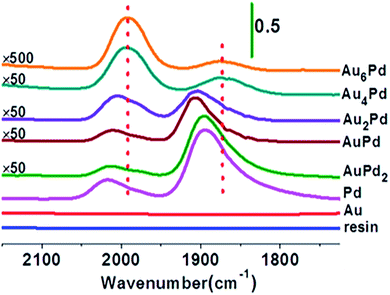 |
| Fig. 13 DRIFT spectra of CO adsorption on Au–Pd/resin catalysts with different Au/Pd atomic ratios. This figure has been adapted/reproduced from ref. 134 with permission from American Chemical Society, copyright 2014. | |
The ion exchange method is effective in producing high loadings of single atoms on the surface of a support; however, only limited types of SACs can be synthesized via this method.
2.2.3.2 Templated hydrothermal method. The templated hydrothermal method was first adopted to synthesize SACs by the Thomas group. The authors used AlPO-18, a framework-substituted aluminophosphate, which could be hydrothermally treated with various divalent ions, such as Mn, Co and Zn,135–139 as a precursor for the synthesis of SACs.The Co cations mainly existed in the following two states when they were incorporated into AlPO-18 with a high loading (about 10 at%): a substantial amount of Co3+ replacing the Al3+ sites in AlPO-18 and the other portion of Co3+ ions located at the opposite ends of each AlPO-18 cage.139 The existing states of Co3+ were detected via EXAFS, which suggested that they were singly dispersed. Similar arguments pertaining to an ionic active site were also obtained for a Fe–AlPO-31 catalyst, which behaved multi-functionally for the selective oxidation of cyclohexane to adipic acid in O2.135,139 In both of the cases, Co2+/Co3+ and Fe2+/Fe3+ functioned predominantly as ionic oxidation catalysts. It has been shown that a combination of coordinative unsaturation at the ionic site and its ability to exhibit redox behaviour are essential requirements for its role as a catalytically active centre.
For the catalysts prepared by templated hydrothermal method, again, there is little doubt that in each case a single atom does act as a catalytically active site for reactions such as the selective oxidation of hydrocarbons.135–140 At the same time, unfortunately, only limited types of SACs could be synthesized via the templated hydrothermal method (Table 7).
Table 7 Summary of precursors and metals for templated hydrothermal synthesis of SACs
Method |
Precursor |
Synthesis of single-atom metals |
Templated hydrothermal |
Mn2+, Co2+, Zn2+ (ref. 135–139) |
Pt, Pd,141 Rh, Ru, Au, Ir142 |
2.2.3.3 Co-precipitation method. The co-precipitation method is another typical catalyst preparation approach, in which a soluble mixture of precursors for the active component, support and even promoter are co-precipitated by a suitable precipitant. SACs can be obtained by drying or calcining the resulting sediments.The adoption of the co-precipitation method to synthesize SACs was initiated by Zhang et al.3,143 In their work, the mixture solution of chloroplatinic acid and ferric nitrate was co-precipitated in an alkaline environment, and the resulting precipitate was filtered, washed and calcined to obtain Pt1/FeOx catalyst as shown in Fig. 14a (Sample A: Pt loading was 0.17 wt% and Pt/Fe atomic ratio was 1/1430. Sample B: Pt loading was 2.5 wt% and Pt/Fe atomic ratio was 1/95). Pt1/FeOx exhibited remarkably high catalytic performance in CO oxidation and nitroarene hydrogenation reactions.3,143 Following the successful preparation of Pt1/FeOx, the co-precipitation method was extended to synthesize other metallic catalysts, such as atomically dispersed Ir144–146 and Pd147 (the existence of Pd single atoms was confirmed via HAADF-STEM, X-ray absorption near edge structure (XANES), EXAFS and corresponding energy dispersive X-ray spectroscopy (EDX) mapping, as shown in Fig. 14c–i).
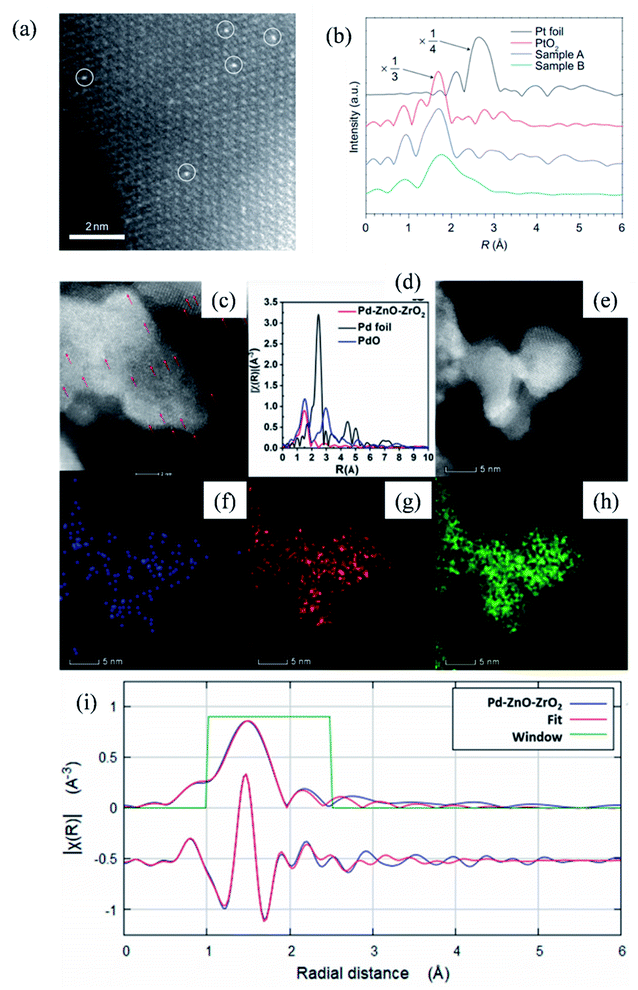 |
| Fig. 14 (a) HAADF-STEM image and (b) EXAFS analysis of Pt1/FeOx catalyst; (c)–(i) HAADF-STEM, XANES, EXAFS and corresponding EDX mapping of Pd SAC. This figure has been adapted/reproduced from ref. 3 and 147 with permission from Nature Publishing Group and Springer Nature, copyright 2011 and 2020. | |
The co-precipitation method is important for preparing composite oxides containing two or more uniformly dispersed metal elements.148 However, the metal loading is low. Moreover, many of the parameters in the catalyst preparation process including the solution addition speed, droplet size, stirring degree, reaction temperature, pH value and reaction time, have considerable influence on the properties and performance of the catalyst and need to be strictly controlled. In addition, some of the active metal atoms of catalysts prepared by the co-precipitation method are not exposed and cannot participate in the reaction, which reduces the overall performance of SACs (Table 8).
Table 8 Summary of metals used in the synthesis of SACs by co-precipitation method
Method |
Synthesis of single-atom metals |
Co-precipitation |
Pt,143 Ir,144–146 Pd147 |
2.2.3.4 One-pot wet chemistry method. The one-pot synthesis method is a strategy to improve the efficiency of the synthesis approach whereby the catalyst precursors are subjected to successive chemical reactions within one single reactor.149–151 The one-pot wet chemistry method can be used to synthesize atomically dispersed Ru on ultrathin Pd nanoribbons via a two-step process, in which Pd nanoribbons are firstly obtained by heating a mixture of Pd(acac)2, polyvinylpyrrolidone, dimethylformamide and water in a CO atmosphere. Subsequently, a Ru salt is injected into the suspension of Pd nanoribbons to allow Ru to be singly dispersed onto the ultrathin Pd nanoribbons.152 Through this method, the content of singly dispersed Ru sites on Pd nanoribbons could be as high as 5.9%. The ultrathin Ru1/Pd nanoribbons exhibited good catalytic selectivity for the hydrogenation of C
C bonds due to the remarkable inhibition of the hydrogenolysis pathway.152In addition, a series of gold pseudo-atom supported MOF catalysts (PSAC-Au/MOFs) could be prepared via the one-pot wet chemistry method on a large scale. The as prepared PSAC-Au/MOFs exhibited excellent catalytic activity in the hydrogenation of p-nitrophenol.153
The one-pot wet chemistry method does not require special experimental setup and multistep reaction conditions; thus, it favours for large scale production. Moreover, high loading density of isolated metal is achievable via this approach. However, the one-pot synthesis approach is still challenging for the preparation of SACs due to the potential solubility limitations of the various precursors in the required reaction solvents and the influence of impurities and by-products generated in each separate step.
2.2.3.5 Metal leaching method. The metal leaching method is generally carried out by immersing the conventional supported metal catalysts in a diluted aqueous NaCN solution and then rinsing at a high pH NaOH solution at room temperature in the presence of O2. In this process, the metal nanoparticles are selectively eluted, with the metal cations remaining intact. This method was firstly adopted for extracting Au from gold mine154,155 and further developed to prepare metal oxides supported metal cation catalysts.156,157Via the leaching method, a series of singly dispersed Au catalysts could be prepared via a two-step process (deposition–precipitation followed by leaching approach), including Au/CeO2,158–166 Au/Al2O3,167 Au/Fe2O3,165 AuLaCeOx,161 Au/La2O3,168 Au/La2O2SO4 (ref. 168) and Au(OH)x/TiO2.156 Not only limited to Au, the leaching method has successfully extended its application to the synthesis of several other SACs, with singly dispersed Pt/CeO2 catalyst as a typical example.160,166
The metal leaching method is only applicable to a few certain metals and supports, and it is not suitable for the large-scale preparation of SACs in the foreseeable future due to the possible environmental pollution during the catalyst preparation process.
2.2.3.6 Flame spray pyrolysis method. Flame spray pyrolysis is an efficient technique for synthesizing metal catalysts with uniform size and has significant advantages in the preparation of supported metal catalysts. For instance, Muravev et al. used two methods, the traditional impregnation and flame spray pyrolysis, to synthesize Pd1/CeO2 catalysts.169 For both of the two Pd1/CeO2 catalysts, there were no Pd or PdO clusters on the surface of CeO2 (as evidenced by HAADF-STEM, Fig. 15). However, during its evaluation in CO oxidation reaction, the Pd atoms in Pd1/CeO2 catalyst prepared by the traditional impregnation method aggregated into metal clusters and deactivated rapidly. As for Pd1/CeO2 catalyst prepared by flame spray pyrolysis method, Pd2+ penetrated into the lattice of CeO2 (as shown in Fig. 15b). The surface Pd single atoms exhibited high sintering resistance under CO oxidation conditions.
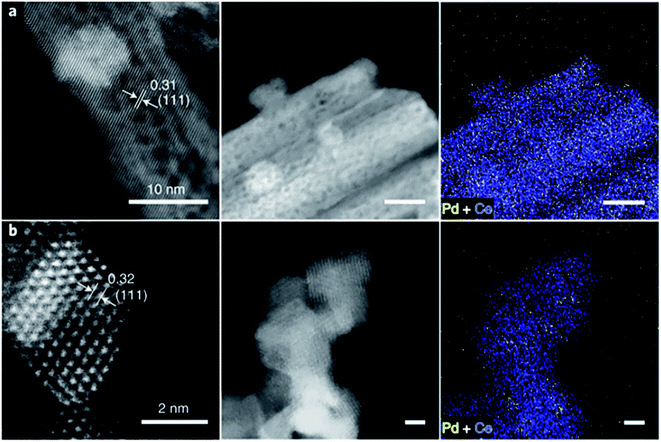 |
| Fig. 15 Structure HAADF-STEM image of Pd1/CeO2 catalyst. (a) By impregnation method and (b) by flame spray pyrolysis. This figure has been adapted/reproduced from ref. 169 with permission from Nature Publishing Group, copyright 2021. | |
Flame spray pyrolysis method has some advantages in the preparation of supported metal catalysts.170 ① It could mix all precursors at the atomic level. ② By changing the synthesis conditions, the shape and particle size of the catalysts can be effectively controlled. ③ The catalysts could be produced on a large scale, with a single step and high efficiency. However, so far, there are few examples of high-temperature catalytic applications for the synthesis of SACs by flame spray pyrolysis, and it is impossible to infer from the existing literature whether flame spray pyrolysis is indeed a feasible method for high-temperature SACs.
2.2.3.7 Other wet chemistry strategies for the synthesis of SACs. In addition to the above methods, several other wet chemistry strategies have also been predicted or proved capable of synthesizing SACs, such as metal–ligand self-assembly method, electrochemical potential window strategy and so on. For example, electrochemical potential window strategy is proposed to be effective in producing high-loading and high-purity SACs, such as Pt1/N-graphene, Pd1/N-graphene, Ni1/N-graphene, Pt1/Fe3O4, Pd1/Fe3O4 and Ni1/Fe3O4;171 Spatial sites separation strategy could be used to fabricate carbon-supported atomically isolated Ni catalyst, which is highly active in electrochemical CO2 reduction reaction;172 Post-synthesis method, including spatial confining, freeze-drying and reducing steps, has been verified efficient for the construction of Pd1/silicoaluminophosphate-31 (Pd1/SAPO-31) catalyst.173
3. Summary and outlooks
SACs are an important category of catalysts. To date, significant efforts have been devoted to develop SACs and tens of methods have been adopted for the synthesis of different types of catalysts at an atomic scale. Based on the above discussions, a summary of the advantages and disadvantages of wet and dry chemical routes is listed in Table 9.
Table 9 Summary of the advantages and disadvantages of wet and dry chemical routes
Classification |
Synthesis method |
Advantages |
Disadvantages |
Dry chemistry routes |
Atomic layer deposition |
(1) Precise control of parameters |
(1) Only materials with suitable ligands or functional groups could be chosen as support |
(2) Excellent deposition uniformity and reproducibility |
(2) Not applicable for the commercial preparation |
(3) The loading of the singly dispersed atoms could be adjusted via regulating the number of cycles |
|
Pyrolysis synthesis |
Straightforward for the preparation of SACs |
Requires high pyrolysis temperature |
Atom trapping |
The operation is simple |
(1) Need to provide mobile atoms and support that could capture mobile species |
(2) High temperature synthesis |
Two-step doping |
High stability |
Requires high energy atom/ion generator |
Ball-milling |
Simple, green, scalable production |
The catalysts are prone to agglomeration |
Wet chemistry routes |
Facile adsorption |
Simple and easy to operate |
Proper interaction between active metal components and catalyst support is required |
Strong electrostatic adsorption |
Applicable for the preparation of high-quality precious metal SACs |
Adsorption behavior is affected by many factors |
Wetness impregnation |
Simple to operate |
Generally metal atoms are not uniformly dispersed on the surface of support |
Photoreduction |
No special equipment is required, easy to implement |
Catalytic active center generally is not uniform |
Galvanic replacement |
Simple, versatile |
Limited by the requirement of an appropriate difference in electrochemical potentials between the two metals |
Cyclic voltammetry |
Less additives are demanded and no interfering products are generated |
An electrochemical station is needed and the operation cost is high |
Ion exchange |
It could produce highly loaded single atoms on the surface of the support |
Only applicable for the synthesis of limited types of SACs |
Templated hydrothermal |
Simple, easy to operate |
Only applicable for the synthesis of limited types of SACs |
Co-precipitation |
Applicable for preparing composite oxides containing two or more uniformly dispersed metal elements |
(1) Preparation parameters have a great impact on performance |
(2) Some active atoms are not exposed and cannot participate in the reaction |
One-pot wet chemistry |
(1) No special experimental setup and multi-step reaction conditions are required, which is beneficial for large-scale production |
(1) Limited by the solubility of various precursors |
(2) High loading of isolated metals can be achieved |
(2) Impurities and by-products of individual steps have an impact |
Metal leaching |
Preparation of monodispersed catalysts by a two-step method |
(1) Suitable for a small number of certain metals and supports |
(2) Environmental pollution during the preparation process |
Flame spray pyrolysis |
(1) It could mix all precursors at the atomic level |
Few application examples |
(2) The shape and particle size of the catalysts can be effectively controlled |
(3) Large-scale production |
In spite of the advantages and the rapid progress, challenges and tasks remain.
3.1 It is challenging to exclude the co-existence of continuously packed sites
Due to the un-uniformity nature of heterogeneous catalysts, over most of the as-prepared SACs, continuously packed sites coexist with singly dispersed sites (Fig. 16). It is challenging to obtain SACs with uniform-structured active sites. Therefore, there are great significance and challenges to develop novel approaches to synthesize SACs effectively.
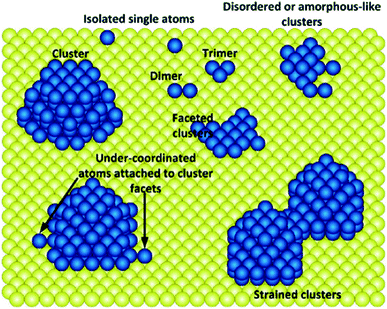 |
| Fig. 16 Schematic illustration showing the co-existence of singly dispersed sites and continuously packed sites. This figure has been adapted/reproduced from ref. 32 with permission from American Chemical Society, copyright 2021. | |
3.2 Technologies for the characterization of SACs need innovation
The most important thing in the study of SACs is to determine the existence and spatial distribution of isolated single atoms. The intuitive method is to directly image the single atoms on the surface of the support via electron microscopy, such as HAADF-STEM. However, only a limited portion of the catalysts is observed, which could not fully reflect the properties of SACs. EXAFS and XANES techniques have been widely used to obtain information on the structural details of SACs, nevertheless, the operation is quite complicated and the analysis of EXAFS and XANES data are complex, which pose obstacles to the reproducibility of the final results. In addition, DRIFT could also be used to assess the presence of individual metal atoms using suitable probe molecules such as CO, NH3, pyridine, etc. Especially, CO FTIR/DRIFT spectroscopy is very useful for detecting noble metals, whereas its application is limited by the interactions between the probe molecules and SACs. Raman spectrum is susceptible to errors caused by external influences, but it could be used to identify M–O–Ce (M = Pt, Rh, Ru) linkages. In conclusion, there is no such a technique that could directly and fully tell the structure of SACs. It is necessary to combine multiple techniques to characterize SACs accurately and effectively. Moreover, the development of new characterization techniques is of great significance for the development of SACs.
3.3 The singly dispersed sites over SACs might undergo transformation under the realistic reaction atmosphere
Over SACs, the singly dispersed atoms are regarded as the active sites to drive reactions. Some catalysts might undergo transformations under the realistic reaction atmosphere; therefore, it is necessary to confirm and determine the singly dispersed sites over SACs via in situ/operando techniques. Although several in situ/operando techniques have been used for the characterization of SACs, including HAADF-STEM, EXAFS, XANES, DRIFT, atmospheric pressure X-ray photoelectron spectroscopy, and high energy resolution fluorescence detected XANES, each technique has strengths and limitations. There is still lack of techniques which can readily provide information on the structure of each singly dispersed atoms on SACs. Therefore, the combination of these advanced techniques is highly desired to characterize SACs readily and precisely.
3.4 Application areas and development prospects of SACs
The applications of SACs span from traditional thermal-driven catalysis, photocatalysis as well as electrochemical catalysis, including CO oxidation,174 hydrogenation,175,176 CO2 reduction,177,178 NOx reduction,179 hydrogen evolution reaction,180,181 oxygen reduction reaction182,183 and so on. The common characteristics of these reactions is that even a singly dispersed atom could drive its occurrence. With the development of SACs, it is prospected that the applications of SACs in more other reactions would be explored and the performance of SACs could be further enhanced.
In conclusion, SACs are a promising research area. Even though the research on SACs has made great progress, there is lack of techniques which could readily provide information on the nature of SACs under operation conditions and the synthesis of SACs with unified structures remains challenging. Therefore, more efforts are still needed and there is enough space to advance this research further.
Conflicts of interest
There are no conflicts to declare.
Acknowledgements
This work received financial support from the National Natural Science Foundation of China (21902116) and the Education Department of Liaoning Province (JQL202015401).
References
- M. K. Samantaray, V. D'Eia, E. Pump, L. Falivene, M. Harb, S. O. Chikh, L. Cavallo and J. M. Basset, The comparison between single atom catalysis and surface organometallic catalysis, Chem. Rev., 2020, 120, 734–813 Search PubMed.
- A. Wang, J. Li and T. Zhang, Heterogeneous single-atom catalysis, Nat. Rev. Chem., 2018, 2, 65–81 Search PubMed.
- B. Qiao, A. Wang, X. Yang, L. F. Allard, Z. Jiang, Y. Cui, J. Liu, J. Li and T. Zhang, Single-atom catalysis of CO oxidation using Pt1/FeOx, Nat. Chem., 2011, 3, 634–641 Search PubMed.
- Y. Chen, R. Gao, S. Ji, H. Li, K. Tang, P. Jiang, H. Hu, Z. Zhang, H. Hao, Q. Qu, X. Liang, W. Chen, J. Dong, D. Wang and Y. Li, Atomic-level modulation of electronic density at cobalt single-atom sites derived from metal-organic frameworks: enhanced oxygen reduction performance, Angew. Chem., Int. Ed., 2021, 60, 3212–3221 Search PubMed.
- T. Zhang, A. G. Walsh, J. Yu and P. Zhang, Single-atom alloy catalysts: structural analysis, electronic properties and catalytic activities, Chem. Soc. Rev., 2021, 50, 569–588 Search PubMed.
- S. Ren, Q. Yu, X. Yu, P. Rong, L. Jiang and J. Jiang, Graphene-supported metal single-atom catalysts: a concise review, Sci. China Mater., 2020, 63, 903–920 Search PubMed.
- J. Liu, C. Cao, X. Liu, L. Zheng, X. Yu, Q. Zhang, L. Gu, R. Qi and W. Song, Direct observation of metal oxide nanoparticles being transformed into metal single atoms with oxygen-coordinated structure and hgh-loadings, Angew. Chem., Int. Ed., 2021, 60, 15248–15253 Search PubMed.
- H. Wu, X. Yang, S. Zhao, L. Zhai, G. Wang, B. Zhang and Y. Qin, Encapsulation of atomically dispersed Pt clusters in porous TiO2 for semi-hydrogenation of phenylacetylene, Chem. Commun., 2019, 9, 2898–2905 Search PubMed.
- S. H. Talib, Z. Lu, X. Yu, K. Ahmad, B. Bashir, Z. Yang and J. Li, Theoretical inspection of M1/PMA single-atom electrocatalyst: ultra-high performance for water splitting (HER/OER) and oxygen reduction reactions (OER), ACS Catal., 2021, 11, 8929–8941 Search PubMed.
- Z. Han, C. Tang, J. Wang, L. Li and C. Li, Atomically dispersed Ptn+ species as highly active sites in Pt/In2O3 catalysts for methanol synthesis from CO2 hydrogenation, J. Catal., 2021, 394, 236–244 Search PubMed.
- W. Xie, H. Li, G. Cui, J. Li, Y. Song, S. Li, X. Zhang, J. Y. Lee, M. Shao and M. Wei, NiSn atomic pair on an integrated electrode for synergistic electrocatalytic CO2 reduction, Angew. Chem., Int. Ed., 2021, 60, 7382–7388 Search PubMed.
- L. Zhou, P. Zhou, Y. Zhang, B. Liu, P. Gao and S. Guo, 3D star-like atypical hybrid MOF derived single-atom catalyst boosts oxygen reduction catalysis, J. Energy Chem., 2021, 55, 355–360 Search PubMed.
- S. H. Talib, X. Yu, Z. Lu, K. Ahmad, T. Yang, H. Xiao and J. Li, A polyoxometalate cluster-based single-atom catalyst for NH3 synthesis via an enzymatic mechanism, J. Mater. Chem. A, 2022, 10, 6165–6177 Search PubMed.
- L. Shi, Y. Huang, Z.-H. Lu, W. Cen, X. Yu, S. Qing, Z. Gao, R. Zhang and G. Feng, Surface property of the Cu doped gamma-Al2O3: a density functional theory study, Appl. Surf. Sci., 2021, 535, 147651 Search PubMed.
- S. H. Talib, S. Baskaran, X. Yu, Q. Yu, B. Bashir, S. Muhammad, S. Hussain, X. Chen and J. Li, Non-noble metal single-atom catalyst of Co1/MXene (Mo2CS2) for CO oxidation, Sci. China Mater., 2021, 64, 651–663 Search PubMed.
- K. Harrath, X. Yu, H. Xiao and J. Li, The key role of support surface hydrogenation in the CH4 to CH3OH selective oxidation by a ZrO2-supported single-atom catalyst, ACS Catal., 2019, 9, 8903–8909 Search PubMed.
- X. Yu, X. Tian and S. Wang, Adsorption of Ni, Pd, Pt, Cu, Ag and Au on the Fe3O4(111) surface, Surf. Sci., 2014, 628, 141–147 Search PubMed.
- X. Yu, S.-G. Wang, Y.-W. Li, J. Wang and H. Jiao, Single gold atom adsorption on the Fe3O4(111) surface, J. Phys. Chem. C, 2012, 116, 10632–10638 Search PubMed.
- X. Yu, X. Zhang, S. Wang and G. Feng, Adsorption of Aun (n=1-4) clusters on Fe3O4(001) B-termination, RSC Adv., 2015, 5, 45446–45453 Search PubMed.
- S. H. Talib, X. Yu, Q. Yu, S. Baskaran and J. Li, Non-noble metal single-atom catalysts with phosphotungstic acid (PTA) support: a theoretical study of ethylene epoxidation, Sci. China Mater., 2020, 63, 1003–1014 Search PubMed.
- J. Ihanus, M. P. Lankinen, M. Kemell, M. Ritala and M. Leskela, Aging of electroluminescent ZnS: Mn thin films deposited by atomic layer deposition processes, J. Appl. Phys., 2005, 98, 113526 Search PubMed.
- M. A. Tagliente, M. Penza, M. Gusso and A. Quirini, Characterisation of ZnS: Mn thin films by Rietveld refinement of bragg-Brentano X-ray diffraction patterns, Thin Solid Films, 1999, 353, 129–136 Search PubMed.
- J. W. Lim and S. J. Yun, Characterization of AlON-TiON stacked insulators for ZnS: Mn thin film electroluminescent devices, Electrochem. Solid State Lett., 2004, 7, H33–H35 Search PubMed.
- C. Chen, S. Husurianto, X. Lu and M. D. Koretsky, The effect of processing conditions on crystal orientation and structure in ZnS: Mn thin films, J. Electrochem. Soc., 1998, 145, 226–229 Search PubMed.
- A. Goudarzi, G. M. Aval, S. S. Park, M. C. Choi, R. Sahraei, M. H. Ullah, A. Avane and C. S. Ha, Low-temperature growth of nanocrystalline Mn-doped ZnS thin films prepared by chemical bath deposition and optical properties, Chem. Mater., 2009, 21, 2375–2385 Search PubMed.
- Q. Zhai, J. Li, J. S. Lewis, K. A. Waldrip, K. Jones, P. H. Holloway, M. Davidson and N. Evans, Microstructure and electroluminescence of ZnS: Mn doped with KCl, Thin Solid Films, 2002, 414, 105–112 Search PubMed.
- R. Sahraei, A. Daneshfar, A. Goudarzi, S. Abbasi, M. H. M. Ara and F. Rahimi, Optical properties of nanocrystalline ZnS: Mn thin films prepared by chemical bath deposition method, J. Mater. Sci. Mater. Electron., 2013, 24, 260–266 Search PubMed.
- J. A. Ruffner, R. T. Tuenge, S. S. Sun, P. D. Grandon and P. F. Hlava, Sputter deposition of ZnS: Mn/SrS: Ce multilayered thin film white phosphor, Thin Solid Films, 1997, 310, 123–131 Search PubMed.
- L. Zhang, H. C. Jiang, C. Liu, J. W. Dong and P. Chow, Annealing of Al2O3 thin films prepared by atomic layer deposition, J. Phys. D Appl. Phys., 2007, 40, 3707–3713 Search PubMed.
- A. G. Silva, K. Pedersen, Z. S. Li, J. Hvam, R. Dhiman and P. Morgen, Growth of aluminum oxide on silicon carbide with an atomically sharp interface, J. Vac. Sci. Technol., A, 2017, 35, 01B142 Search PubMed.
- Y. S. Kim, J. S. Kang, S. J. Yun and K. I. Cho, Multilayered tantalum-aluminum oxide films grown by atomic layer deposition, J. Korean Phys. Soc., 1999, 35, S216–S220 Search PubMed.
- J. Fonseca and J. L. Lu, Single-atom catalysts designed and prepared by the atomic layer deposition technique, ACS Catal., 2021, 11, 7018–7059 Search PubMed.
- M. Fenker, M. Balzer and H. Kappl, Corrosion protection with hard coatings on steel: past approaches and current research efforts, Surf. Coat. Technol., 2014, 257, 182–205 Search PubMed.
- H. W. Wang and J. L. Lu, Atomic layer deposition: a gas phase route to bottom-up precise synthesis of heterogeneous catalyst, Acta Phys.-Chim. Sin., 2018, 34, 1334–1357 Search PubMed.
- K. Cao, J. M. Cai, X. Liu and R. Chen, Catalysts design and synthesis via selective atomic layer deposition, J. Vac. Sci. Technol., A, 2018, 36, 010801 Search PubMed.
- R. Huang, Y. Cheng, Y. Ji and R. J. Gorte, Atomic layer deposition for preparing isolated Co sites on SiO2 for ethane dehydrogenation catalysis, Nanomaterials, 2020, 10, 244 Search PubMed.
- K. Cao, J. Cai and R. Chen, Inherently selective atomic layer deposition and applications, Chem. Mater., 2020, 32, 2195–2207 Search PubMed.
- J. Lu, A perspective on new opportunities in atom-by-atom synthesis of heterogeneous catalysts using atomic layer deposition, Catal. Lett., 2021, 151, 1535–1545 Search PubMed.
- M. Zhao, J. Feng, W. Yang, S. Song and H. Zhang, Recent advances in graphitic carbon nitride supported single-atom catalysts for energy conversion, Chemcatchem, 2021, 13, 1250–1270 Search PubMed.
- C. Wang, H. Tissot, M. Soldemo, J. Lu and J. Weissenrieder, Inverse single-site Fe1(OH)x/Pt(111) model catalyst for preferential oxidation of CO in H2, Nano Res., 2022, 15, 709–715 Search PubMed.
- H. Yan, H. F. Lv, H. Yi, W. Liu, Y. J. Xia, X. H. Huang, W. X. Huang, S. Q. Wei, X. J. Wu and J. L. Lu, Understanding the underlying mechanism of improved selectivity in pd1 single-atom catalyzed hydrogenation reaction, J. Catal., 2018, 366, 70–79 Search PubMed.
- S. H. Sun, G. X. Zhang, N. Gauquelin, N. Chen, J. G. Zhou, S. L. Yang, W. F. Chen, X. B. Meng, D. S. Geng, M. N. Banis, R. Y. Li, S. Y. Ye, S. Knights, G. A. Botton, T. K. Sham and X. L. Sun, Single-atom catalysis using Pt/graphene achieved through atomic layer deposition, Sci. Rep., 2013, 3, 1775 Search PubMed.
- S. Stambula, N. Gauquelin, M. Bugnet, S. Gorantla, S. Turner, S. H. Sun, J. Liu, G. X. Zhang, X. L. Sun and G. A. Botton, Chemical structure of nitrogen-doped graphene with single platinum atoms and atomic clusters as a platform for the PEMFC electrode, J. Phys. Chem. C, 2014, 118, 3890–3900 Search PubMed.
- N. C. Cheng, S. Stambula, D. Wang, M. N. Banis, J. Liu, A. Riese, B. W. Xiao, R. Y. Li, T. K. Sham, L. M. Liu, G. A. Botton and X. L. Sun, Platinum single-atom and cluster catalysis of the hydrogen evolution reaction, Nat. Commun., 2016, 7, 13638 Search PubMed.
- H. Yan, H. Cheng, H. Yi, Y. Lin, T. Yao, C. L. Wang, J. J. Li, S. Q. Wei and J. L. Lu, Single-atom Pd1/graphene catalyst achieved by atomic layer deposition: remarkable
performance in selective hydrogenation of 1,3-butadiene, J. Am. Chem. Soc., 2015, 137, 10484–10487 Search PubMed.
- J. Ji, Z. Li, C. Hu, Y. Sha, S. Li, X. Gao, S. Zhou, T. Qiu, C. Liu, X. Su, Y. Hou, Z. Lin, S. Zhou, M. Ling and C. Liang, Platinum atomic clusters embedded in defects of anatase/graphene for efficient electro- and photocatalytic hydrogen evolution, ACS Appl. Mater. Interfaces, 2020, 12, 40204–40212 Search PubMed.
- J. Qi, Q. Xu, J. Sun, D. Zhou and J. Yin, Synthesis, characterization and analysis of graphene-supported single-atom catalysts, Prog. Chem., 2020, 32, 505–518 Search PubMed.
- D. Xu, J. Yin, Y. Gao, D. Zhu and S. Wang, Atomic-scale designing of zeolite based catalysts by atomic layer deposition, Chemphyschem, 2021, 22, 1287–1301 Search PubMed.
- J. L. Lu, J. W. Elam and P. C. Stair, Atomic layer deposition-Sequential self-limiting surface reactions for advanced catalyst "bottom-up" synthesis, Surf. Sci. Rep., 2016, 71, 410–472 Search PubMed.
- P. Thissen, A. Vega, T. Peixoto and Y. J. Chabal, Controlled, low-coverage metal oxide activation of silicon for organic functionalization: unraveling the phosphonate bond, Langmuir, 2012, 28, 17494–17505 Search PubMed.
- C. Y. Kim, J. W. Elam, M. J. Pellin, D. K. Goswami, S. T. Christensen, M. C. Hersam, P. C. Stair and M. J. Bedzyk, Imaging of atomic layer deposited (ALD) tungsten monolayers on alpha-TiO2(110) by X-ray standing wave Fourier inversion, J. Phys. Chem. B, 2006, 110, 12616–12620 Search PubMed.
- A. J. M. Mackus, M. A. Verheijen, N. Leick, A. A. Bol and W. M. M. Kessels, Influence of oxygen exposure on the nucleation of platinum atomic layer deposition: consequences for film growth, nanopatterning, and nanoparticle synthesis, Chem. Mater., 2013, 25, 1905–1911 Search PubMed.
- M. Jafari and P. M. Zimmerman, Reliable and efficient reaction path and transition state finding for surface reactions with the growing string method, J. Comput. Chem., 2017, 38, 645–658 Search PubMed.
- L. Jeloaica, A. Esteve, A. Dkhissi, D. Esteve and M. Djafari-Rouhani, Three-step mechanism of the water recombination reactions on SiO2/Si surface in the first stage of ZrO2 atomic layer deposition, Comput. Mater. Sci., 2005, 33, 59–65 Search PubMed.
- T. Sun, Q. Wu, O. Zhuo, Y. F. Jiang, Y. F. Bu, L. J. Yang, X. Z. Wang and Z. Hu, Manganese oxide-induced strategy to high-performance iron/nitrogen/carbon electrocatalysts with highly exposed active sites, Nanoscale, 2016, 8, 8480–8485 Search PubMed.
- N. Hering, K. Schreiber, R. Riedel, O. Lichtenberger and J. Woltersdorf, Synthesis of polymeric precursors for the formation of nanocrystalline Ti-C-N/amorphous Si-C-N composites, Appl. Organomet. Chem., 2001, 15, 879–886 Search PubMed.
- P. Subramanian and A. Schechter, Electrochemical oxygen reduction activity of cobalt-nitrogen-carbon composite catalyst prepared by single precursor pyrolysis under autogenic pressure, J. Electrochem. Soc., 2016, 163, F428–F436 Search PubMed.
- A. Videla, L. Osmieri, M. Armandi and S. Specchia, Varying the morphology of Fe-N-C electrocatalysts by templating iron phthalocyanine precursor with different porous SiO2 to promote the oxygen reduction reaction, Electrochim. Acta, 2015, 177, 43–50 Search PubMed.
- H. R. Chen, K. Shen, J. Y. Chen, X. D. Chen and Y. W. Li, Hollow-ZIF-templated formation of a ZnO@C-N-Co core-shell nanostructure for highly efficient pollutant photodegradation, J. Mater. Chem. A, 2017, 5, 9937–9945 Search PubMed.
- W. X. Wang, Y. W. Li, R. J. Zhang, D. H. He, H. L. Liu and S. J. Liao, Metal-organic framework as a host for synthesis of nanoscale Co3O4 as an active catalyst for CO oxidation, Catal. Commun., 2011, 12, 875–879 Search PubMed.
- R. Jiang, L. Li, T. Sheng, G. F. Hu, Y. G. Chen and L. Y. Wang, Edge-site engineering of atomically dispersed Fe-N-4 by selective C-N bond cleavage for enhanced oxygen reduction reaction activities, J. Am. Chem. Soc., 2018, 140, 11594–11598 Search PubMed.
- G. X. Zhang, Y. Jia, C. Zhang, X. Y. Xiong, K. Sun, R. D. Chen, W. X. Chen, Y. Kuang, L. R. Zheng, H. L. Tang, W. Liu, J. F. Liu, X. M. Sun, W. F. Lin and H. J. Dai, A general route via formamide condensation to prepare atomically dispersed metal-nitrogen-carbon electrocatalysts for energy technologies, Energy Environ. Sci., 2019, 12, 1317–1325 Search PubMed.
- A. Zitolo, N. Ranjbar-Sahraie, T. Mineva, J. K. Li, Q. Y. Jia, S. Stamatin, G. F. Harrington, S. M. Lyth, P. Krtil, S. Mukerjee, E. Fonda and F. Jaouen, Identification of catalytic sites in cobalt-nitrogen-carbon materials for the oxygen reduction reaction, Nat. Commun., 2017, 8, 957 Search PubMed.
- S. F. Fu, C. Z. Zhu, D. Su, J. H. Song, S. Y. Yao, S. Feng, M. H. Engelhard, D. Du and Y. H. Lin, Porous carbon-hosted atomically dispersed iron-nitrogen moiety as enhanced electrocatalysts for oxygen reduction reaction in a wide range of pH, Small, 2018, 14, 1703118 Search PubMed.
- C. Zhang, W. Zhang and W. T. Zheng, Transition metal-nitrogen-carbon active site for oxygen reduction electrocatalysis: beyond the fascinations of TM-N-4, Chemcatchem, 2019, 11, 655–668 Search PubMed.
- L. Peng, X. Duan, Y. Shang, B. Gao and X. Xu, Engineered carbon supported single iron atom sites and iron clusters from Fe-rich enteromorpha for Fenton-like reactions via nonradical pathways, Appl. Catal. B Environ., 2021, 287, 119963 Search PubMed.
- F. Xiao, X. Liu, C.-J. Sun, I. Hwang, Q. Wang, Z. Xu, Y. Wang, S. Zhu, H.-W. Wu, Z. Wei, L. Zheng, D. Cheng, M. Gu, G.-L. Xu, K. Amine and M. Shao, Solid-state synthesis of highly dispersed nitrogen-coordinated single iron atom electrocatalysts for proton exchange membrane fuel cells, Nano Lett., 2021, 21, 3633–3639 Search PubMed.
- W. Chen, X. Luo, T. J. A. Slater, Y. Zhou, S. Ling, R. Bao, J. Alves Fernandes, J. Wang and Y. Shen, General synthesis of single atom electrocatalysts via a facile condensation-carbonization process, J. Mater. Chem. A, 2020, 8, 25959–25969 Search PubMed.
- E. Luo, Y. Chu, J. Liu, Z. Shi, S. Zhu, L. Gong, J. Ge, C. H. Choi, C. Liu and W. Xing, Pyrolyzed M-N-x catalysts for oxygen reduction reaction: progress and prospects, Energy Environ. Sci., 2021, 14, 2158–2185 Search PubMed.
- Z. Zhang, S. Yao, X. Hu, F. Okejiri, K. He, P. Liu, Z. Tian, V. P. Dravid, J. Fu, X. Zhu and S. Dai, Sacrificial synthesis of supported Ru single atoms and clusters on N-doped carbon derived from covalent triazine frameworks: a charge modulation approach, Adv. Sci., 2021, 8, 2001493 Search PubMed.
- X. Cui, X. Dai, A.-E. Surkus, K. Junge, C. Kreyenschulte, G. Agostini, N. Rockstroh and M. Beller, Zinc single atoms on N-doped carbon: an efficient and stable catalyst for CO2 fixation and conversion, Chin. J. Catal., 2019, 40, 1679–1685 Search PubMed.
- X. Q. Tao, R. Long, D. Wu, Y. G. Hu, G. H. Qiu, Z. M. Qi, B. X. Li, R. B. Jiang and Y. J. Xiong, Anchoring positively charged Pd single atoms in ordered porous ceria to boost catalytic activity and stability in suzuki coupling reactions, Small, 2020, 16, 2001782 Search PubMed.
- Y. Han, J. Dai, R. Xu, W. Ai, L. Zheng, Y. Wang, W. Yan, W. Chen, J. Luo, Q. Liu, D. Wang and Y. Li, Notched-polyoxometalate strategy to fabricate atomically dispersed Ru catalysts for biomass conversion, ACS Catal., 2021, 11, 2669–2675 Search PubMed.
- L. Huang, K. Wu, Q. He, C. Xiong, T. Gan, X. He and H. Ji, Quasi-continuous synthesis of iron single atom catalysts via a microcapsule pyrolysis strategy, AIChE J., 2021, 67, e17197 Search PubMed.
- D. Wu, J. Hu, C. Zhu, J. Zhang, H. Jing, C. Hao and Y. Shi, Salt melt synthesis of chlorella-derived nitrogen-doped porous carbon with atomically dispersed CoN4 sites for efficient oxygen reduction reaction, J. Colloid Interface Sci., 2021, 586, 498–504 Search PubMed.
- H. Xu, S. Zhang, J. Geng, G. Wang and H. Zhang, Cobalt single atom catalysts for the efficient electrosynthesis of hydrogen peroxide, Inorg. Chem. Front., 2021, 8, 2829–2834 Search PubMed.
- P. Q. Yin, T. Yao, Y. Wu, L. R. Zheng, Y. Lin, W. Liu, H. X. Ju, J. F. Zhu, X. Hong, Z. X. Deng, G. Zhou, S. Q. Wei and Y. D. Li, Single cobalt atoms with precise N-coordination as superior oxygen reduction reaction catalysts, Angew. Chem., Int. Ed., 2016, 55, 10800–10805 Search PubMed.
- B. Volosskiy, H. L. Fei, Z. P. Zhao, S. Lee, M. F. Li, Z. Y. Lin, B. Papandrea, C. Wang, Y. Huang and X. F. Duan, Tuning the catalytic activity of a metal-organic framework derived copper and nitrogen Co-doped carbon composite for oxygen reduction reaction, ACS Appl. Mater. Interfaces, 2016, 8, 26769–26774 Search PubMed.
- U. Tylus, Q. Y. Jia, K. Strickland, N. Ramaswamy, A. Serov, P. Atanassov and S. Mukerjee, Elucidating oxygen reduction active sites in pyrolyzed metal-nitrogen coordinated non-precious-metal electrocatalyst systems, J. Phys. Chem. C, 2014, 118, 8999–9008 Search PubMed.
- Y. Hou, T. Z. Huang, Z. H. Wen, S. Mao, S. M. Cui and J. H. Chen, Metal-organic framework-derived nitrogen-doped core-shell-structured porous Fe/Fe3C@C nanoboxes supported on graphene sheets for efficient oxygen reduction reactions, Adv. Energy Mater., 2014, 4, 1400337 Search PubMed.
- B. L. Chen, G. P. Ma, Y. Q. Zhu and Y. D. Xia, Metal-organic-frameworks derived cobalt embedded in various carbon structures as bifunctional electrocatalysts for oxygen reduction and evolution reactions, Sci. Rep., 2017, 7, 5266 Search PubMed.
- H. S. Lu, H. M. Zhang, R. R. Liu, X. Zhang, H. J. Zhao and G. Z. Wang, Macroscale cobalt-MOFs derived metallic Co nanoparticles embedded in N-doped porous carbon layers as efficient oxygen electrocatalysts, Appl. Surf. Sci., 2017, 392, 402–409 Search PubMed.
- A. Morozan, V. Goellner, Y. Nedellec, J. Hannauer and F. Jaouen, Effect of the transition metal on metal-nitrogen-carbon catalysts for the hydrogen evolution reaction, J. Electrochem. Soc., 2015, 162, H719–H726 Search PubMed.
- P. Zhou, L. Jiang, F. Wang, K. J. Deng, K. L. Lv and Z. H. Zhang, High performance of a cobalt-nitrogen complex for the reduction and reductive coupling of nitro compounds into amines and their derivatives, Sci. Adv., 2017, 3, e1601945 Search PubMed.
- L. L. Zhang, A. Q. Wang, W. T. Wang, Y. Q. Huang, X. Y. Liu, S. Miao, J. Y. Liu and T. Zhang, Co-N-C catalyst for C-C coupling reactions: on the catalytic performance and active sites, ACS Catal., 2015, 5, 6563–6572 Search PubMed.
- Q. Liu, J. Wang, J. Zhang, Y. Yan, X. Qiu, S. Wei and Y. Tang, In situ immobilization of isolated Pd single-atoms on graphene by employing amino-functionalized rigid molecules and their prominent catalytic performance, Catal. Sci. Technol., 2020, 10, 450–457 Search PubMed.
- J. Jones, H. F. Xiong, A. T. Delariva, E. J. Peterson, H. Pham, S. R. Challa, G. S. Qi, S. Oh, M. H. Wiebenga, X. I. P. Hernandez, Y. Wang and A. K. Datye, Thermally stable single-atom platinum-on-ceria catalysts via atom trapping, Science, 2016, 353, 150–154 Search PubMed.
- S. J. Wei, A. Li, J. C. Liu, Z. Li, W. X. Chen, Y. Gong, Q. H. Zhang, W. C. Cheong, Y. Wang, L. R. Zheng, H. Xiao, C. Chen, D. S. Wang, Q. Peng, L. Gu, X. D. Han, J. Li and Y. D. Li, Direct observation of noble metal nanoparticles transforming to thermally stable single atoms, Nat. Nanotechnol., 2018, 13, 856–861 Search PubMed.
- Y. T. Qu, Z. J. Li, W. X. Chen, Y. Lin, T. W. Yuan, Z. K. Yang, C. M. Zhao, J. Wang, C. Zhao, X. Wang, F. Y. Zhou, Z. B. Zhuang, Y. Wu and Y. D. Li, Direct transformation of bulk copper into copper single sites via emitting and trapping of atoms, Nat. Catal., 2018, 1, 781–786 Search PubMed.
- J. Yang, Z. Y. Qiu, C. M. Zhao, W. C. Wei, W. X. Chen, Z. J. Li, Y. T. Qu, J. C. Dong, J. Luo, Z. Y. Li and Y. Wu, In situ thermal atomization to convert supported nickel nanoparticles into surface-bound nickel single-atom catalysts, Angew. Chem., Int. Ed., 2018, 57, 14095–14100 Search PubMed.
- H. T. Wang, Q. X. Wang, Y. C. Cheng, K. Li, Y. B. Yao, Q. Zhang, C. Z. Dong, P. Wang, U. Schwingenschlogl, W. Yang and X. X. Zhang, Doping monolayer graphene with single atom substitutions, Nano Lett., 2012, 12, 141–144 Search PubMed.
- Z. T. Bai, L. Zhang and L. Liu, Bombarding graphene with oxygen ions: combining effects of incident angle and ion energy to control defect generation, J. Phys. Chem. C, 2015, 119, 26793–26802 Search PubMed.
- A. Baby, L. Trovato and C. D. Valentin, Single atom catalysts (SAC) trapped in defective and nitrogen-doped graphene supported on metal substrates, Carbon, 2021, 174, 772–788 Search PubMed.
- T. Gan, Y. Liu, Q. He, H. Zhang, X. He and H. Ji, Facile synthesis of kilogram-scale Co alloyed Pt single-atom catalysts via ball milling for hydrodeoxygenation of 5-hydroxymethylfurfural, ACS Sustainable Chem. Eng., 2020, 8, 8692–8699 Search PubMed.
- T. R. Shojaei, M. Tabatabaei, H. Mobli, M. Aghbashlo, S. A. Rashid and T. Tan, Applications of nanotechnology and carbon nanoparticles in agriculture, Synthesis, Technology and Applications of Carbon Nanomaterials, 2019, vol. 11, pp. 247–277 Search PubMed.
- B. T. Qiao, J. X. Liang, A. Q. Wang, C. Q. Xu, J. Li, T. Zhang and J. Y. Liu, Ultrastable single-atom gold catalysts with strong covalent metal-support interaction (CMSI), Nano Res., 2015, 8, 2913–2924 Search PubMed.
- P. Munnik, P. E. de Jongh and K. P. de Jong, Recent developments in the synthesis of supported catalysts, Chem. Rev., 2015, 115, 6687–6718 Search PubMed.
- L. Jiao and J. R. Regalbuto, The synthesis of highly dispersed noble and base metals on silica via strong electrostatic adsorption: I. amorphous silica, J. Catal., 2008, 260, 329–341 Search PubMed.
- S. Lambert, N. Job, L. D'Souza, M. F. R. Pereira, R. Pirard, B. Heinrichs, J. L. Figueiredo, J. P. Pirard and J. R. Regalbuto, Synthesis of very highly dispersed platinum catalysts supported on carbon xerogels by the strong electrostatic adsorption method, J. Catal., 2009, 261, 23–33 Search PubMed.
- N. Job, S. Lambert, M. Chatenet, C. J. Gommes, F. Maillard, S. Berthon-Fabry, J. R. Regalbuto and J. P. Pirard, Preparation of highly loaded Pt/carbon xerogel catalysts for proton exchange membrane fuel cells by the strong electrostatic adsorption method, Catal. Today, 2010, 150, 119–127 Search PubMed.
- A. Morales-Garcia, M. Rubes and P. Nachtigall, The interaction of Pd clusters with the bulk and layered two-dimensional silicalite-1 supports, Catal. Today, 2016, 277, 108–117 Search PubMed.
- D. Pillay and M. D. Johannes, Comparison of sulfur interaction with hydrogen on Pt(111), Ni(111) and Pt3Ni(111) surfaces: the effect of intermetallic bonding, Surf. Sci., 2008, 602, 2752–2757 Search PubMed.
- D. Pillay and M. D. Johannes, Effect of S on Pt(111) and Pt3Ni(111) surfaces: a first principles study, J. Phys. Chem. C, 2008, 112, 1544–1551 Search PubMed.
- X. J. Cui, K. Junge, X. C. Dai, C. Kreyenschulte, M. M. Pohl, S. Wohlrab, F. Shi, A. Bruckner and M. Beller, Synthesis of single atom based heterogeneous platinum catalysts: high selectivity and activity for hydrosilylation reactions, ACS Cent. Sci., 2017, 3, 580–585 Search PubMed.
- Z. L. Zhang, Y. H. Zhu, H. Asakura, B. Zhang, J. G. Zhang, M. X. Zhou, Y. Han, T. Tanaka, A. Q. Wang, T. Zhang and N. Yan, Thermally stable single atom Pt/m-Al2O3 for selective hydrogenation and CO oxidation, Nat. Commun., 2017, 8, 16100 Search PubMed.
- G. Vile, D. Albani, M. Nachtegaal, Z. P. Chen, D. Dontsova, M. Antonietti, N. Lopez and J. Perez-Ramirez, A stable single-site palladium catalyst for hydrogenations, Angew. Chem., Int. Ed., 2015, 54, 11265–11269 Search PubMed.
- J. H. Kwak, J. Z. Hu, D. Mei, C. W. Yi, D. H. Kim, C. H. F. Peden, L. F. Allard and J. Szanyi, Coordinatively unsaturated Al3+ centers as binding sites for active catalyst phases of platinum on gamma-Al2O3, Science, 2009, 325, 1670–1673 Search PubMed.
- H. Xiong, A. K. Datye and Y. Wang, Thermally stable single-atom heterogeneous catalysts, Adv. Mater., 2021, 33, 2004319 Search PubMed.
- F. Yang, S. Ding, H. Song and N. Yan, Single-atom Pd dispersed on nanoscale anatase TiO2 for the selective hydrogenation of phenylacetylene, Sci. China Mater., 2020, 63, 982–992 Search PubMed.
- P. X. Liu, Y. Zhao, R. X. Qin, S. G. Mo, G. X. Chen, L. Gu, D. M. Chevrier, P. Zhang, Q. Guo, D. D. Zang, B. H. Wu, G. Fu and N. F. Zheng, Photochemical route for synthesizing atomically dispersed palladium catalysts, Science, 2016, 352, 797–801 Search PubMed.
- B. Wang, H. Cai and S. H. Shen, Single metal atom photocatalysis, Small Methods, 2019, 3, 180047 Search PubMed.
- N. Dimitrov, Recent advances in the growth of metals, alloys, and multilayers by surface limited
redox replacement (SLRR) based approaches, Electrochim. Acta, 2016, 209, 599–622 Search PubMed.
- S. Bhanushali, S. Mahasivam, R. Ramanathan, M. Singh, E. L. H. Mayes, B. J. Murdoch, V. Bansal and M. Sastry, Photomodulated spatially confined chemical reactivity in a single silver nanoprism, ACS Nano, 2020, 14, 11100–11109 Search PubMed.
- H. Xu, K. Zhang, B. Yan, J. T. Zhong, S. M. Li and Y. K. Du, Facile synthesis of Pd-decorated Pt/Ru networks with highly improved activity for methanol electrooxidation in alkaline media, New J. Chem., 2017, 41, 3048–3054 Search PubMed.
- L. Han, P. F. Wang, H. Liu, Q. Q. Tan and J. Yang, Balancing the galvanic replacement and reduction kinetics for the general formation of bimetallic CuM (M = Ru, Rh, Pd, Os, Ir, and Pt) hollow nanostructures, J. Mater. Chem. A, 2016, 4, 18354–18365 Search PubMed.
- C. X. Xu, Y. Q. Liu, J. P. Wang, H. R. Geng and H. J. Qiu, Fabrication of nanoporous Cu-Pt(Pd) core/shell structure by galvanic replacement and its application in electrocatalysis, ACS Appl. Mater. Interfaces, 2011, 3, 4626–4632 Search PubMed.
- R. Ojani, Z. Abkar, E. Hasheminejad and J. B. Raoof, Rapid fabrication of Cu/Pd nano/micro-particles porous-structured catalyst using hydrogen bubbles dynamic template and their enhanced catalytic performance for formic acid electrooxidation, Int. J. Hydrogen Energy, 2014, 39, 7788–7797 Search PubMed.
- X. X. Cao, A. Mirjalili, J. Wheeler, W. Xie and B. W. L. Jang, Investigation of the preparation methodologies of Pd-Cu single atom alloy catalysts for selective hydrogenation of acetylene, Front. Chem. Sci. Eng., 2015, 9, 442–449 Search PubMed.
- C. J. Hsu, C. W. Huang, Y. W. Hao and F. Q. Liu, Au/Pd core-shell nanoparticles for enhanced electrocatalytic activity and durability, Electrochem. Commun., 2012, 23, 133–136 Search PubMed.
- B. Rezaei, E. Havakeshian and A. A. Ensafi, Electrocatalytic activity of bimetallic Pd-Au nanostructure supported on nanoporous stainless steel surface using galvanic replacement reaction toward the glycerol oxidation in alkaline media, J. Electroanal. Chem., 2016, 782, 108–116 Search PubMed.
- J. H. Shim and J. Son, Fabrication of sponge-like nanoporous Pd/Au electrocatalysts for oxygen reduction through a galvanic replacement reaction, presented at 246th National Meeting of the American-Chemical-Society, Indianapolis, 2013 Search PubMed.
- R. Ojani, J. B. Raoof and E. Hasheminejad, One-step electroless deposition of Pd/Pt bimetallic microstructures by galvanic replacement on copper substrate and investigation of its performance for the hydrogen evolution reaction, Int. J. Hydrogen Energy, 2013, 38, 92–99 Search PubMed.
- B. Rezaei, M. Molzhtarianpour and A. A. Ensafi, Fabricated of bimetallic Pd/Pt nanostructure deposited on copper nanofoam substrate by galvanic replacement as an effective electrocatalyst for hydrogen evolution reaction, Int. J. Hydrogen Energy, 2015, 40, 6754–6762 Search PubMed.
- R. Wu, Q. C. Kong, C. L. Fu, S. Q. Lai, C. Ye, J. Y. Liu, Y. X. Chen and J. Q. Hu, One-pot synthesis and enhanced catalytic performance of Pd and Pt nanocages via galvanic replacement reactions, RSC Adv., 2013, 3, 12577–12580 Search PubMed.
- V. Venkatasamy, N. Jayaraju, S. M. Cox, C. Thambidurai and J. L. Stickney, Studies of Hg((1-x))Cd(x)Te formation by electrochemical atomic layer deposition and investigations into bandgap engineering, J. Electrochem. Soc., 2007, 154, H720–H725 Search PubMed.
- X. H. Xia, Y. Wang, A. Ruditskiy and Y. N. Xia, 25th anniversary article: galvanic replacement: a simple and versatile route to hollow nanostructures with tunable and well-controlled properties, Adv. Mater., 2013, 25, 6313–6333 Search PubMed.
- R. E. Rettew, J. W. Guthrie and F. M. Alamgir, Layer-by-layer Pt growth on polycrystalline Au: surface-limited redox replacement of overpotentially deposited Ni monolayers, J. Electrochem. Soc., 2009, 156, D513–D516 Search PubMed.
- F. Merkoci, J. Patarroyo, L. Russo, J. Piella and A. Genc, Understanding galvanic replacement reactions: the case of Pt and Ag, Mater. Today Adv., 2020, 5, 100037 Search PubMed.
- L. Zhang, L. Han, H. Liu, X. Liu and J. Luo, Potential-cycling synthesis of single platinum atoms for efficient hydrogen evolution in neutral media, Angew. Chem., Int. Ed., 2017, 56, 13694–13698 Search PubMed.
- M. C. Hu, Z. Y. Wu, Z. H. Yao, J. S. Young, L. L. Luo, Y. G. Du, C. M. Wang, Z. Iqbal and X. Q. Wang, N-8 stabilized single-atom Pd for highly selective hydrogenation of acetylene, J. Catal., 2021, 395, 46–53 Search PubMed.
- H. Small, T. S. Stevens and W. C. Bauman, Novel ion-exchange chromatographic method using conductimetric detection, Anal. Chem., 1975, 47, 1801–1809 Search PubMed.
- A. D. Yapryntsev, A. E. Baranchikov and V. K. Ivanov, Layered rare-earth hydroxides: a new family of anion-exchangeable layered inorganic materials, Russ. Chem. Rev., 2020, 89, 629–666 Search PubMed.
- L.-Q. Yu, W.-J. Xia, W.-J. Ma, T.-E. Wen, S.-L. Chen, F. Jin, B.-C. Huang and R.-C. Jin, Universal method to fabricate transition metal single-atom-anchored carbon with excellent oxygen reduction reaction activity, ACS Appl. Mater. Interfaces, 2021, 13, 13534–13540 Search PubMed.
- L. Zhang, A. Wang, J. T. Miller, X. Liu, X. Yang, W. Wang, L. Li, Y. Huang, C. Y. Mou and T. Zhang, Efficient and durable Au alloyed Pd single-atom catalyst for the ullmann reaction of aryl chlorides in water, ACS Catal., 2014, 4, 1546–1553 Search PubMed.
- J. M. Thomas and R. Raja, The advantages and future potential of single-site heterogeneous catalysts, Top. Catal., 2006, 40, 3–17 Search PubMed.
- J. M. Thomas, R. Raja, G. Sankar and R. G. Bell, Molecular-sieve catalysts for the selective oxidation of linear alkanes by molecular oxygen, Nature, 1999, 398, 227–230 Search PubMed.
- M. Dugal, G. Sankar, R. Raja and J. M. Thomas, Designing a heterogeneous catalyst for the production of adipic acid by aerial oxidation of cyclohexane, Angew. Chem., Int. Ed., 2000, 39, 2310–2313 Search PubMed.
- R. Raja, G. Sankar and J. M. Thomas, Designing a molecular sieve catalyst for the aerial oxidation of n-hexane to adipic acid, Angew. Chem., Int. Ed., 2000, 39, 2313–2316 Search PubMed.
- J. M. Thomas, R. Raja, G. Sankar and R. G. Bell, Molecular sieve catalysts for the regioselective and shape-selective oxyfunctionalization of alkanes in air, Acc. Chem. Res., 2001, 34, 191–200 Search PubMed.
- C. Peng, R. Yan, H. G. Peng, Y. Y. Mi, J. Liang, W. M. Liu, X. Wang, G. Song, P. Wu and F. D. Liu, One-pot synthesis of layered mesoporous ZSM-5 plus Cu ion-exchange: enhanced NH3-SCR performance on Cu-ZSM-5 with hierarchical pore structures, J. Hazard. Mater., 2020, 385, 121593 Search PubMed.
- M. N. Garcia, P. Verma, D. S. Torres, R. Raja, K. Mori and H. Yamashita, Single-site heterogeneous catalysts and photocatalysts for emerging applications, Advanced Heterogeneous Catalysts Volume 2: Applications at the Single-Atom Scale, 2020, vol. 7, pp. 151–188 Search PubMed.
- S. F. Yuk, G. Collinge, M. Nguyen, M. S. Lee and R. Rousseau, Single-atom catalysis: An analogy between heterogeneous and homogeneous catalysts, Advanced Heterogeneous Catalysts Volume 2: Applications at the Single-Atom Scale, 2020, vol. 1, pp. 1–15 Search PubMed.
- H. S. Wei, X. Y. Liu, A. Q. Wang, L. L. Zhang, B. T. Qiao, X. F. Yang, Y. Q. Huang, S. Miao, J. Y. Liu and T. Zhang, FeOx-supported platinum single-atom and pseudo-single-atom catalysts for chemoselective hydrogenation of functionalized nitroarenes, Nat. Commun., 2014, 5, 5634 Search PubMed.
- J. X. Liang, J. Lin, X. F. Yang, A. Q. Wang, B. T. Qiao, J. Y. Liu, T. Zhang and J. Li, Theoretical and experimental investigations on single-atom catalysis: Ir-1/FeOx for CO oxidation, J. Phys. Chem. C, 2014, 118, 21945–21951 Search PubMed.
- J. X. Liang, X. F. Yang, A. Q. Wang, T. Zhang and J. Li, Theoretical investigations of non-noble metal single-atom catalysis: Ni1/FeOx for CO oxidation, Catal. Sci. Technol., 2016, 6, 6886–6892 Search PubMed.
- T. Yang, R. Fukuda, S. Hosokawa, T. Tanaka, S. Sakaki and M. Ehara, A theoretical investigation on CO oxidation by single-atom catalysts M1/Al2O3 (M=Pd, Fe, Co, and Ni), Chemcatchem, 2017, 9, 1222–1229 Search PubMed.
- G. Ding, L. Hao, H. Xu, L. Wang, J. Chen, T. Li, X. Tu and Q. Zhang, Atomically dispersed palladium catalyses Suzuki-Miyaura reactions under phosphine-free conditions, Commun. Chem., 2020, 3, 43 Search PubMed.
- Y. Lou and J. Y. Liu, CO oxidation on metal oxide supported single Pt atoms: the role of the support, Ind. Eng. Chem. Res., 2017, 56, 6916–6925 Search PubMed.
- V. R. Akhmetova and E. B. Rakhimova, One-pot cyclothiomethylation of amines as efficient method for the synthesis of saturated five-, six-, seven-, and eight-membered S,N-heterocycles, Russ. J. Org. Chem., 2014, 50, 1711–1731 Search PubMed.
- S. Y. Liu, L. Zhou, L. Y. Yao, L. Y. Chai, L. Li, G. Zhang, Kankan and K. Y. Shi, One-pot reflux method synthesis of cobalt hydroxide nanoflake-reduced graphene oxide hybrid and their NOx gas sensors at room temperature, J. Alloys Compd., 2014, 612, 126–133 Search PubMed.
- T. Miyazaki, H. Sato, T. Sakakibara and Y. Kajihara, An approach to the precise chemoenzymatic synthesis of C-13-labeled sialyloligosaccharide on an intact glycoprotein: a novel one-pot 3-C-13-labeling method for sialic acid analogues by control of the reversible aldolase reaction, enzymatic synthesis of 3-C-13 -NeuAc-alpha-(2 -> 3)- U-C-13 -Gal-beta-(1 -> 4)-GlcNAc-beta- sequence onto glycoprotein, and its conformational analysis by developed NMR techniques, J. Am. Chem. Soc., 2000, 122, 5678–5694 Search PubMed.
- J. J. Ge, D. S. He, W. X. Chen, H. X. Ju, H. Zhang, T. T. Chao, X. Q. Wang, R. You, Y. Lin, Y. Wang, J. F. Zhu, H. Li, B. Xiao, W. X. Huang, Y. Wu, X. Hong and Y. D. Li, Atomically dispersed Ru on ultrathin Pd nanoribbons, J. Am. Chem. Soc., 2016, 138, 13850–13853 Search PubMed.
- Z. Wang, L. Gu, L. Song, H. Wang and R. Yu, Facile one-pot synthesis of MOF supported gold pseudo-single-atom catalysts for hydrogenation reactions, Mater. Chem. Front., 2018, 2, 1024–1030 Search PubMed.
- A. Muezzinoglu, A review of environmental considerations on gold mining and production, Crit. Rev. Environ. Sci. Technol., 2003, 33, 45–71 Search PubMed.
- S. S. Konyratbekova, A. Baikonurova and A. Akcil, Non-cyanide leaching processes in gold hydrometallurgy and iodine-iodide applications: a review, Miner. Process. Extr. Metall. Rev., 2015, 36, 198–212 Search PubMed.
- M. Yang, L. F. Allard and M. Flytzani-Stephanopoulos, Atomically dispersed Au-(OH)x species bound on titania catalyze the low-temperature water-gas shift reaction, J. Am. Chem. Soc., 2013, 135, 3768–3771 Search PubMed.
- M. Flytzani-Stephanopoulos, Gold atoms stabilized on various supports catalyze the water-gas shift reaction, Acc. Chem. Res., 2014, 47, 783–792 Search PubMed.
- Y. Guan and E. J. M. Hensen, Cyanide leaching of Au/CeO2: highly active gold clusters for 1,3-butadiene hydrogenation, Phys. Chem. Chem. Phys., 2009, 11, 9578–9582 Search PubMed.
- Y. J. Guan, D. A. J. M. Ligthart, O. Pirgon-Galin, J. A. Z. Pieterse, R. A. van Santen and E. J. M. Hensen, Gold stabilized by nanostructured ceria supports: nature of the active sites and catalytic performance, Top. Catal., 2011, 54, 424–438 Search PubMed.
- Q. Fu, H. Saltsburg and M. Flytzani-Stephanopoulos, Active nonmetallic Au and Pt species on ceria-based water-gas shift catalysts, Science, 2003, 301, 935–938 Search PubMed.
- W. Deng, A. I. Frenkel, R. Si and M. Flytzani-Stephanopoulos, Reaction-relevant gold structures in the low temperature water-gas shift reaction on Au-CeO2, J. Phys. Chem. C, 2008, 112, 12834–12840 Search PubMed.
- R. Si and M. Flytzani-Stephanopoulos, Shape and crystal-plane effects of nanoscale ceria on the activity of Au-CeO2 catalysts for the water-gas shift reaction, Angew. Chem., Int. Ed., 2008, 47, 2884–2887 Search PubMed.
- N. Yi, R. Si, H. Saltsburg and M. Flytzani-Stephanopoulos, Active gold species on cerium oxide nanoshapes for methanol steam reforming and the water gas shift reactions, Energy Environ. Sci., 2010, 3, 831–837 Search PubMed.
- D. Tibiletti, A. Amieiro-Fonseca, R. Burch, Y. Chen, J. M. Fisher, A. Goguet, C. Hardacre, P. Hu and A. Thompsett, DFT and in situ EXAFS investigation of gold/ceria-zirconia low-temperature water gas shift catalysts: identification of the nature of the active form of gold, J. Phys. Chem. B, 2005, 109, 22553–22559 Search PubMed.
- W. L. Deng, C. Carpenter, N. Yi and M. Flytzani-Stephanopoulos, Comparison of the activity of Au/CeO2 and Au/Fe2O3 catalysts for the CO oxidation and the water-gas shift reactions, Top. Catal., 2007, 44, 199–208 Search PubMed.
- W. L. Deng and M. Flytzani-Stephanopoulos, On the issue of the deactivation of Au-ceria and Pt-ceria water-gas shift catalysts in practical fuel-cell applications, Angew. Chem., Int. Ed., 2006, 45, 2285–2289 Search PubMed.
- G. A. Filonenko, W. L. Vrijburg, E. J. M. Hensen and E. A. Pidko, On the activity of supported Au catalysts in the liquid phase hydrogenation of CO2 to formates, J. Catal., 2016, 343, 97–105 Search PubMed.
- J. D. Lessard, I. Valsamakis and M. Flytzani-Stephanopoulos, Novel Au/La2O3 and Au/La2O2SO4 catalysts for the water-gas shift reaction prepared via an anion adsorption method, Chem. Commun., 2012, 48, 4857–4859 Search PubMed.
- V. Muravev, G. Spezzati, Y.-Q. Su and A. Parastaev, Interface dynamics of Pd–CeO2 single-atom catalysts during CO oxidation, Nat. Catal., 2021, 4, 469–478 Search PubMed.
- S. Ding, H.-A. Chen, X. Fu, Q. He, J. Panpranot, C.-M. Yang and N. Yan, High-temperature flame spray pyrolysis induced stabilization of Pt single-atom catalysts, Appl. Catal., B, 2021, 281, 119471 Search PubMed.
- J.-C. Liu, H. Xiao and J. Li, Constructing high-loading single-atom/cluster catalysts via an electrochemical potential window strategy, J. Am. Chem. Soc., 2020, 142, 3375–3383 Search PubMed.
- Q. Wu, J. Liang, Z.-L. Xie, Y.-B. Huang and R. Cao, Spatial sites separation strategy to fabricate atomically isolated nickel catalysts for efficient CO2 electroreduction, ACS Mater. Lett., 2021, 3, 454–461 Search PubMed.
- J. Wang, Q. Kuang, X. Su, X. Lu, L. Leng, M. Zhang, C. Guo, T. Li, Q. Xu, S. Sun, J. H. Horton, W. Wu and Z. Li, Isolated palladium atoms dispersed on silicoaluminophosphate-31 (SAPO-31) for the semihydrogenation of alkynes, ACS Appl. Nano Mater., 2021, 4, 861–868 Search PubMed.
- X.-P. Zou, L.-N. Wang, X.-N. Li, Q.-Y. Liu, Y.-X. Zhao, T.-M. Ma and S.-G. He, Noble-metal-free single-atom catalysts CuAl4O7-9− for CO oxidation by O2, Angew. Chem., Int. Ed., 2018, 57, 10989–10993 Search PubMed.
- R. Lin, D. Albani, E. Fako, S. K. Kaiser, O. V. Safonova, N. Lopez and J. Perez-Ramirez, Design of single gold atoms on nitrogen-doped carbon for molecular recognition in alkyne semi-hydrogenation, Angew. Chem., Int. Ed., 2019, 58, 504–509 Search PubMed.
- J. Fu, J. Dong, R. Si, K. Sun, J. Zhang, M. Li, N. Yu, B. Zhang, M. G. Humphrey, Q. Fu and J. Huang, Synergistic effects for enhanced catalysis in a dual single-atom catalyst, ACS Catal., 2021, 11, 1952–1961 Search PubMed.
- J. Wang, E. Kim, D. P. Kumar, A. P. Rangappa, Y. Kim, Y. Zhang and T. K. Kim, Highly durable and fully dispersed cobalt diatomic site catalysts for CO2 photoreduction to CH4, Angew. Chem., Int. Ed., 2021, 60, 2–12 Search PubMed.
- J. Wang, T. Heil, B. Zhu, C.-W. Tung, J. Yu, C. Hao Ming, M. Antonietti and S. Cao, A single Cu-center containing enzyme-mimic enabling full photosynthesis under CO2 reduction, ACS Nano, 2020, 14, 8584–8593 Search PubMed.
- K. Fujiwara and S. E. Pratsinis, Single Pd atoms on TiO2 dominate photocatalytic NOx removal, Appl. Catal. B Environ., 2018, 226, 127–134 Search PubMed.
- Y. Xue, B. Huang, Y. Yi, Y. Guo, Z. Zuo, Y. Li, Z. Jia, H. Liu and Y. Li, Anchoring zero valence single atoms of nickel and iron on graphdiyne for hydrogen evolution, Nat. Commun., 2018, 9, 1460 Search PubMed.
- D. Yi, F. Lu, F. Zhang, S. Liu, B. Zhou, D. Gao, X. Wang and J. Yao, Regulating charge transfer of lattice oxygen in single-atom-doped titania for hydrogen evolution, Angew. Chem., Int. Ed., 2020, 59, 15855–15859 Search PubMed.
- C.-C. Hou, L. Zou, L. Sun, K. Zhang, Z. Liu, Y. Li, C. Li, R. Zou, J. Yu and Q. Xu, Single-atom iron catalysts on overhang-eave carbon cages for high-performance oxygen reduction reaction, Angew. Chem., Int. Ed., 2020, 59, 7384–7389 Search PubMed.
- P. Song, M. Luo, X. Liu, W. Xing, W. Xu, Z. Jiang and L. Gu, Zn single atom catalyst for highly efficient oxygen reduction reaction, Adv. Funct. Mater., 2017, 27, 1700802 Search PubMed.
|
This journal is © The Royal Society of Chemistry 2022 |
Click here to see how this site uses Cookies. View our privacy policy here.