DOI:
10.1039/D1RA09396G
(Review Article)
RSC Adv., 2022,
12, 18848-18863
Production of butanol from lignocellulosic biomass: recent advances, challenges, and prospects
Received
28th December 2021
, Accepted 27th May 2022
First published on 29th June 2022
Abstract
Due to energy and environmental concerns, biobutanol is gaining increasing attention as an alternative renewable fuel owing to its desirable fuel properties. Biobutanol production from lignocellulosic biomass through acetone–butanol–ethanol (ABE) fermentation has gained much interest globally due to its sustainable supply and non-competitiveness with food, but large-scale fermentative production suffers from low product titres and poor selectivity. This review presents recent developments in lignocellulosic butanol production, including pretreatment and hydrolysis of hemicellulose and cellulose during ABE fermentation. Challenges are discussed, including low concentrations of fermentation sugars, inhibitors, detoxification, and carbon catabolite repression. Some key process improvements are also summarised to guide further research and development towards more profitable and commercially viable butanol fermentation.
1. Introduction
The dual pressures of international energy security and climate change are encouraging the development of biofuels.1 Butanol, a useful biofuel and important platform chemical, has attracted great interest due to its excellent fuel properties (high energy density, excellent mixability, low volatility and corrosiveness).2,3 However, high substrate cost, solvent toxicity, low butanol titre and poor productivity make butanol fermentation expensive for large-scale practical applications.4 Traditionally, butanol has been produced via acetone–butanol–ethanol (ABE) fermentation from sugars or starch as substrates, but the cost of substrates (∼60% of the total cost of ABE fermentation) significantly decreased the competitiveness of ABE fermentation, so much so that biobutanol produced by Clostridium cannot compete with petrochemical butanol.5,6
Selection of feasible substrates plays an important role in butanol production. At present, four generations of feedstocks have contributed to produce butanol, and their benefits and limitations are listed in Table 1.7 Among them, lignocellulosic biomass has been evaluated as cheap feedstock for biobutanol production, including rice straw,8 corncobs,9 wheat straw,10 barley straw,11 sorghum bagasse,12 and different types of wood such as pine13 and elm.14,15 Lignocellulosic biomass is the most abundant, sustainable, and cost-effective form of biomass, hence valorisation to biofuel could provide notable benefits, including (i) CO2 fixation from the atmosphere, (ii) reduced air pollution from incineration, and (iii) enhanced energy security for oil-importing countries.16,17 More importantly, utilising lignocellulosic materials could also significantly reduce environmental pollution caused by accumulation of agricultural forestry wastes,18–20 hence it represents an alternative strategy for large-scale biobutanol production.
Table 1 Benefits and limitations of biomass21,22,25
Feedstock |
Benefits |
Limitations |
First-generation feedstock |
Starch and sucrose feedstocks |
High butanol yield |
Occupies potential crop land |
Sufficient fermentable substrates through simple pretreatment processes |
Competes with food supply |
Significantly increases the overall cost of fermentation |
![[thin space (1/6-em)]](https://www.rsc.org/images/entities/char_2009.gif) |
Second-generation feedstock |
Lignocellulose biomass |
Cost-effective, huge carbon resources |
Difficult to achieve sufficient fermentable sugars from complex and recalcitrant biomass |
No competition with food supply |
Requires complex pretreatment and detoxification processes |
![[thin space (1/6-em)]](https://www.rsc.org/images/entities/char_2009.gif) |
Third-generation feedstock |
Algal biomass |
No competition with cultivation land and food supply |
Significantly increases the cost of downstream processes due to low production and productivity of butanol |
Fewer or no lignin and fermentation inhibitors |
Difficult to obtain sufficient fermentable sugars |
![[thin space (1/6-em)]](https://www.rsc.org/images/entities/char_2009.gif) |
Fourth-generation feedstock |
Syngas |
Increased CO2 capture ability |
Still at its infancy as a technology |
No complex pretreatment process needed |
Several unknown key parameters that limit butanol production |
Directly utilises clostridia with a high production rate |
Poor mass transfer from gas to liquid |
Recently, several articles have been published that summarise general aspects of lignocellulosic butanol fermentation and focus on the selection of cheap feedstocks, optimisation of cost-efficient processing methods, and the development of improved microbial strains.21–24 Herein, we review advances in the pretreatment and hydrolysis of lignocellulosic biomass into fermentable substrates, focusing on recent research progress in overcoming the factors limiting lignocellulosic butanol fermentation. Additionally, improvement of lignocellulosic butanol fermentation by metabolic engineering and process integration strategies are also discusses. This review aims to provide guidance for improving the overall performance of lignocellulosic butanol fermentation.
2. Overall process for biobutanol production from lignocellulosic biomass
2.1 Microorganisms and metabolic pathways related to lignocellulosic butanol fermentation
Clostridia are obligate anaerobes and spore-forming bacteria with a complex life cycle, among which C. acetobutylicum, C. beijerincki, C. saccharoperbutylacetonicum and C. saccharobutylicum are the most well-known ABE fermenters, sharing a typical life cycle and metabolic pathways for butanol production.26,27 Following the publication of the genomes of several solventogenic clostridia, such as C. acetobutylicum ATCC 824,28 C. acetobutylicum DSM 173129 and C. acetobutylicum EA2018.30 Clostridia are among the most important industrial production strains for biobutanol, and the main advantage is the availability of a wide range of sugar sources, including pentose (xylose and arabinose), hexose (glucose, fructose, mannose and galactose), disaccharides (lactose, sucrose, maltose and cellobiose) and polysaccharides (starch). Lignocellulosic biomass is composed of various monosaccharides, and is the most abundant renewable resource on earth. Producing biobutanol from wood cellulosic biomass with fermentable sugars an economical and effective strategy.
In ABE industrial fermentation processes, bacterial strains use sugar substrates to fuel their rapid growth, and produce acetic acid, butyric acid and other organic acids, while generating ATP (e.g., the ATP yield of butyrate fermentation is 3 ATP per glucose; this is more than has been reported for fermentations to date31). ATP is the essential energy source needed for bacterial growth and fermentation, and this stage is the acid production stage. During acid production, organic acids such as acetic acid and butyric acid rapidly accumulate, and dissociated acids can adhere to the surface of the cell membrane as an unpaired ion, triggering a collapse of the pH gradient of the cell membrane.32 However, solvent production increases only when butyric acid accumulates and pH decreases, due to the essential role of undissociated butyric acid on the induction of solvent production in C. acetobutylicum.33,34 Subsequently, bacteria consume ATP to maintain the pH gradient across the membrane, and synthesise acetone, ethanol and butanol, during the alcohol production stage. This stage is characterised by solvent toxicity, the most serious of which is butanol toxicity,35 since the fermentation concentration is in the range of the inhibitory concentration for this solvent. To produce butanol efficiently, microbes must possess strong physiological robustness and fitness, coupled with strong metabolic capabilities, to enable them to work efficiently during bioprocesses. In some bacteria, the intracellular pH declines as a function of extracellular pH, and these are more resistant to the toxic effects of fermentation acids.34 In addition, higher ATP levels can divert carbon flux toward butanol formation.36
2.2 Substrates for lignocellulosic butanol
Lignocellulosic biomass, mainly comprising of cellulose, hemicellulose and lignin, are the most abundant, sustainable, and cost-effective types of biomass for butanol production.39,40 Among them, cellulose is an unbranched homopolysaccharide consisting of D-glucopyranosyl units that can be broken down to hexoses (C-6 sugars), and hemicellulose are branched heteropolysaccharides consisting of both hexose and pentose sugar residues.41,42 Lignin consists of phenylpropane units linked through different types of interunit linkages, playing a cementing role to connect cells and increasing the mechanical strength properties, which makes lignocellulosic resistant against biodegradation by microorganisms.43 Therefore, removal of lignin from lignocellulose biomass before hydrolysis of cellulose and hemicellulose is highly desirable for successful lignocellulosic ABE fermentation.44 Due to the physiological properties of solventogenic clostridia, a typical lignocellulosic butanol fermentation process can be summarised in four major steps: pretreatment (breaking down the complex structure of lignocellulosic biomass), hydrolysis (providing fermentable monomers), fermentation and distillation8,45 (Fig. 1).
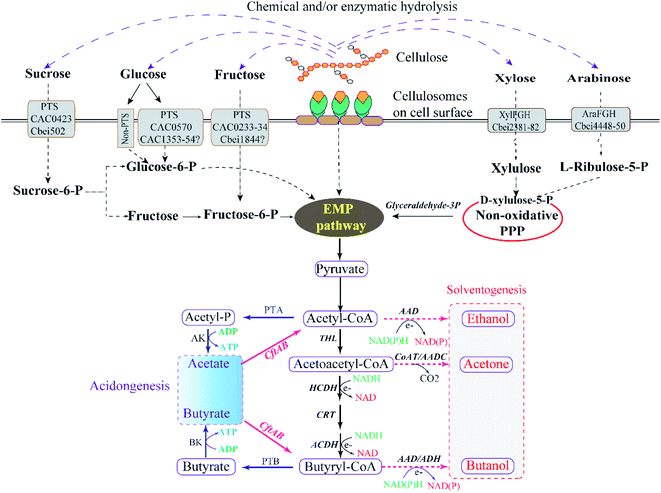 |
| Fig. 1 Schematic diagram of butanol synthesis from lignocellulosic biomass in clostridia.37,38 | |
2.2.1 Pretreatment processes for lignocellulosic biomass. Due to the recalcitrance of lignocellulosic biomass,46 pretreatment is a crucial step that breaks down the complex structure of lignocellulosic materials and increases the surface area and porosity, thereby enhancing saccharification to release fermentable sugars (Fig. 2).47,48 Primarily, pretreatment processes can be classified into four general categories (reviewed in detail by:21,49 (1) physical or mechanical treatment, including milling/grinding, chipping, shredding, extrusion, microwave and ultra-sonication, which effectively breaks down the physical structure of lignocellulosic biomass;50 (2) chemical treatment, including application of concentrated/dilute acid, alkali, ozonolysis, organic solvents and ionic liquids;51,52 (3) physicochemical treatment, involving the use of steam explosion, ammonium fiber explosion, CO2 explosion and hot water, to effectively release monosaccharides and oligosaccharides;53,54 (4) biological treatments, using microorganisms (such as fungi, bacteria, or consortia of fungi and bacteria) to degrade lignin, hemicellulose and cellulose, potentially high selectivity of enzymatic hydrolysis,55 but the effectiveness is influenced by substrate concentration, enzyme loading, pH and temperature.56
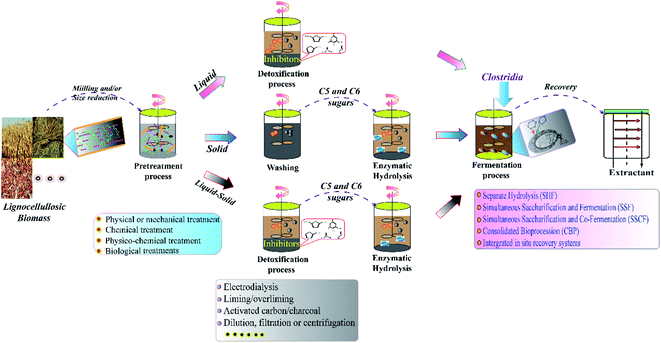 |
| Fig. 2 Schematic diagram of fermentative butanol production from lignocellulosic biomass.8,44 | |
There are several advantages and disadvantages for different pretreatment processes, limiting the economics and feasibility of lignocellulosic butanol. For example, dilute acid and alkali pretreatments are widely used to prepare digestible substrates, but a neutralization step is required to maintain pH before enzymatic hydrolysis. More importantly, salts formed in the neutralisation process may also influence the efficiency of enzymatic hydrolysis and ABE fermentation.57 Recently, deep eutectic solvents have been applied for biomass pre-treatment due to their facile preparation with 100% atom efficiency, low melting point, easy biocompatibility, cost-effectiveness, low viscosity, and environmental friendliness.58,59 In a short time (90 min) and with low energy consumption (120 °C) and a deep eutectic solvent (DES) pretreatment process, total sugars were increased to 37.94 g L−1 (30.59 g L−1 glucose, 7.35 g L−1 xylose) at a low enzyme loading of 7.5 g of filter paper units (FPU) per L of pretreated corn stover (CS), and 13.65 g L−1 total ABE was achieved with high productivity (0.68 g L−1 h−1) and yield (0.38 g g−1) from DES-pretreated CS hydrolysate without any detoxification and sterilisation.60 In addition, combinations of pretreatment method have been also employed and shown to exert synergic effects on the quality of resulting hydrolysate, including a high yield of monomeric sugars and low levels of inhibitors.61,62 For example, after pretreatment with optimised microwave-assisted alkali pretreatment followed by acid hydrolysis, 76.3% lignin removal, 21.1% hemicellulose and 71.9% cellulose were obtained at 640 W microwave power, 2.8% NaOH, and 19 min treatment time, producing 46.2 g L−1 of reducing sugar and 18.7 g L−1 ABE.63
Unfortunately, such pretreatment processes are costly and time-consuming, and pretreating lignocellulosic biomass can also produce several undesirable compounds, in which the concentrations of inhibitors depends on the lignocellulosic biomass and pretreatment methods.17 Therefore, it is desirable to develop suitable pretreatment methods to avoid process inhibition, and thereby decrease costs and energy demands for butanol production from lignocellulosic materials.64 Efforts could be made on the following directions: improving the conversion of lignocellulosic biomass with higher solid loading and low enzyme dosage under mild treat conditions; maximising hemicellulose and cellulose recovery with minimal degradation of lignin; or combining with microbial fermentation process optimization to obtain a more suitable substrate for butanol fermentation without increasing costs and processes. For example, lignocellulosic material wheat straw as a source of fermentable saccharides, and chicken feather as a source of amino acids and peptides, hydrolysis of both materials was carried out simultaneously, resulting in a cultivation medium that was suitable for direct use in biobutanol production.65
2.2.2 Hydrolysis of cellulose and hemicellulose. Cellulose, accounting for ∼40% of lignocellulosic materials, is a long-chain polymer formed by glucose connected by β-1,4-glycosidic bonds,66 and it can be selectively hydrolysed to glucose by cellulolytic enzymes (e.g. exo-1,4-β-glucanases, endo-1,4-β-glucanase and β-glucosidases). The enzymatic hydrolysis efficiency of cellulose is affected by many parameters. For example, when the binding ability of cellulase is weak at high solid-phase load,67 the surface coverage of cellulose is low, which lowers the cellulose hydrolysis efficiency. Improving the enzymatic hydrolysis efficiency of cellulose in fermentation substrates to obtain high levels of fermentable sugars to produce cellulose butanol is a major goal.68Hemicellulose, another cheap and abundant substrate, accounts for ∼25 to 35% of lignocellulose. It is a polyphase polymer composed of several different types of monosaccharides, the main structural units are xylose (>50%), mannose, glucose, arabinose and galactose, and fermenting hemicellulose results in more butanol per unit mass of lignocellulose.41 Compared with the β 1→4 linkages of cellulose, glycoside bonds of hemicellulose are more reactive and can be broken by chemical hydrolysis at milder conditions.69 However, completely hydrolysing different types of glycosidic bonds requires a specific family of glycoside hydrolases, such as endo-β-1,4-xylanase, endo-β-1,4-man-nanase, α-galactosidase, and endo-galactanase. Therefore, although several clostridia can secrete some hemicellulases, the extracellular activity of enzymes is not adequate for direct utilisation of hemicellulose,70 making butanol production from hemicellulose relatively inefficient due to expensive pretreatment processes.71 At present, dilute-acid hydrolysis,72 steam explosion,73 and autohydrolysis/liquid hot water extraction,74 have been evaluated for producing fermentable hemicellulosic sugars. For example, after treatment of hemicellulosic pre-hydrolysates by flocculation, followed by simultaneous detoxification with Ureibacillus thermosphaericus and Cupriavidus taiwanensis co-culture and hydrolysis with Paenibacillus campinasensis, a reduction of phenolic compounds up to 56% was achieved after flocculation, and ABE fermentation could produce 6.8 g L−1 of butanol after 116 h.75
3. Limitations and alleviation strategies for lignocellulosic butanol fermentation
Essential pretreatment and hydrolysis steps limit the fermentation of lignocellulosic biomass, accounting for a large portion of the process cost. Therefore, it is crucial to develop more cost-efficient pretreatment methods that minimise the generation of inhibitors, lower energy consumption, diminish operating costs, and simultaneously maximise fermentable sugar yields with a careful consideration of feedstock properties.76 Due to the low transport and utilization efficiency of fermentable sugars, low butanol yield is another major issue for lignocellulosic fermentation. Additionally, the accumulation of toxic intermediates, or overproduction of foreign proteins during butanol efficient fermentation often caused unwanted cellular stresses, resulting in a decrease in overall cell fitness. Therefore, increase of tolerance and robustness of strain is also important and necessary.77,78
3.1 Lignocellulose fermentation substrate treatment
Catabolite repression, release of inhibitors, and low sugar concentrations have proved to be the limiting factors for lignocellulosic butanol fermentation, and more cost-effective processes are needed to convert hemicellulose and cellulose into fermentable hydrolysates with appropriate concentrations of sugars, inhibitors, and stimulators.
3.1.1 Low-concentration fermentable sugars. During ABE fermentation, the shift from the acidogenesis phase toward the solventogenesis phase is strongly dependent on the concentration of the carbon source after growth phase;79 increasing the concentration of the carbon source can promote fermentation toward the solventogenic phase, and thereby increase the butanol
:
acetone ratio.80 Therefore, mismatching hydrolysis and fermentation processes, in which relatively low levels of sugars can be achieved by typical enzymatic hydrolysis of cellulose, is a major obstacle for lignocellulosic butanol production.68 Achieving high concentrations of fermentable sugars is crucial for lignocellulosic butanol, and several strategies have been developed to increase the total sugar concentration from treatment process:81 (1) using a fractionation pretreatment (e.g., organosolv pretreatment) to generate a cellulose-rich pretreated solid; (2) preparation of hydrolysates by simultaneous enzymatic hydrolysis of cellulose and hemicellulose,82 or supplementing with starch for ABE fermentation,83 but carbon catabolite repression (CCR) may be an issue;84 (3) concentrating hydrolysates by filtration or evaporation, increasing the total sugar concentration to 30–80 g L−1.85
3.1.2 Inhibitors and detoxification processes.
3.1.2.1 Lignocellulose-derived inhibitors. During pretreatment of lignocellulose, several lignocellulose-derived microbial inhibitory compounds (LDMICs)86 are often generated, in which the amounts and species of inhibitors are dependent on raw materials and/or pretreatment processes.54 These inhibitors can be classified into weak organic acids, furan derivatives, and phenolics, with toxicity at the same dose against ABE production ranked formic acid > phenols > furfurals.87 More interestingly, clostridia have different tolerances of and stress responses to these inhibitors.88 Compared with C. beijerinckii,89 C. acetobutylicum is more sensitive to formic acid, and 0.1 M formic acid induces an ‘acid crash’ that completely inhibits butanol production and cell growth.90 However, low concentrations (0.5–2 g L−1) of furfural and 5-hydroxymethyl furfural can stimulate Cupriavidus basilensis, C. beijerinckii BA101,91 C. beijerinckii P260 (ref. 92) and C. acetobutylicum ATCC 824.93
3.1.2.2 Lignin-derived compounds. Compared with weak organic acids and furan derivatives, partial decomposition of (poly)phenolic aromatic compounds in lignin, such as syringealdehyde, vanillic acid and ferulic acid,94 is more toxic to clostridia.95–97 More importantly, combinations of acids and furan aldehydes, as well as different phenolics, can have synergistic effects on ABE fermentation,98 but the sophisticated physiological regulation mechanism remains unclear.99 For example, during ABE fermentation, production of butanol and total solvent was decreased to 6.07 g L−1 from 8.08 g L−1, and to 11.71 g L−1 from 13.95 g L−1, respectively, under 0.5 g L−1 of ferulic acid stress,100 and the biosynthesis of organic acids was severely inhibited at lower concentrations of phenolics (0.2 g L−1 vanillin or/and vanillic acid).98 Furthermore, lignosulfonates, lignin-based organic polymers, can also significantly downregulate glycolysis and the butanol biosynthetic pathway in clostridia due to the unique properties of these anionic, water-soluble, highly acidic compounds.101 For example, augmenting the lignosulfonate concentration >0.5 g L−1 led to a significant decrease in solvent titre (ABE ∼1.50 g L−1), while >1 g L−1 of low-molecular-weight lignosulfonate seriously inhibited solvent synthesis, and even completely blocked the process of ABE fermentation.102
3.1.2.3 Hemicellulose-derived inhibitors. Hexoses and pentoses could be obtained via the chemical hydrolysis of hemicellulose, however, often accompanying with the formation of 5-hydroxymethyl furfural (HMF) and furfural due to dehydration reactions.103,104 More importantly, furan compounds can be also subjected to rehydration reactions to form levulinic acid and formic acid under acidic conditions, providing inhibitory effect on C. acetobutylicum due to the acid crash.67,90 In addition, acetic acid is also produced through hydrolysis of the acetyl group of hemicellulose, and 10 g L−1 of acetic acid slightly increases the ABE fermentation performance, but the higher levels significantly inhibit the fermentation performance of C. acetobutylicum.89 Furthermore, the presence of monosaccharides, oligomers, and some ingredients of hemicellulosic hydrolysates can also inhibit cellulase activity, and then negatively influence the hydrolysis efficiency of cellulose.105
3.1.2.4 Strategies to alleviate inhibition. In general, levels of inhibitors and fermentable sugars in hydrolysates are dependent on the feedstock and the conditions of pretreatment and hydrolysis processes.106 Thus, several strategies have been developed to counteract inhibition problems, including (1) selecting less recalcitrant feedstocks; (2) utilising mild pretreatment conditions; (3) biological hydrolysis of hemicelluloses with specific enzymes, or co-culture of microorganisms producing hydrolysing enzymes107 to effectively decrease the formation of inhibitors (but this can increase several incumbent costs);108 (4) combining pretreatment, hydrolysis, fermentation and product recovery into a single consolidated process;109 (5) integrating several detoxification methods, such as electrodialysis,110 liming/overlimiting,111 activated carbon/charcoal,112 in situ extraction with oleyl alcohol,113 and filtration or centrifugation, and resin treatments114 to remove solids and undissolved lignin in lignocellulosic hydrolysates. However, although these inhibitors can be effectively removed during the detoxification process,115 partial fermentable sugars are also removed from lignocellulosic hydrolysates,116 making the detoxification process impractical for commercial application.117 Thus, it is necessary to develop a cost- and energy-efficient pretreatment stage to improve the conversion of lignocellulosic biomass with higher solid loading and low enzyme dosage under mild treat conditions, thereby maximising hemicellulose and cellulose recovery with minimal degradation of lignin, or minimise inhibitor formation to allow fermentation without detoxification.65
3.2 Transport and utilization efficiency of fermented sugars
The transportation and metabolic pathways of substrates during butanol synthesis have been dissected alongside stoichiometric glucose reactions.30,118 Clostridia use PEP (phosphoenolpyruvate)-dependent PTSs (phosphotransferase systems) and/or non-PTS mechanisms to assimilate glucose during ABE fermentation, and PTSs are predominant in the solventogenic phase. However, some clostridia are dominated by non-PTSs (ATP-dependent glucokinases) during the solventogenic stage, such as the butanol-hyperproducing mutant strain C. beijerinckii BA101, derived from NCIMB 8052.119 PTSs transport and phosphorylate sugar substrates, play a central role in metabolic regulation. For example, there are 13 PTSs in Clostridium related to acetone and butanol,120 among which 12 are encoded by chromosomal genes and can be used to transport glucose, mannose, fructose, lactose, cellulose-disaccharide, sucrose, galactose and maltose. The other PTS is encoded by genes on the large PSOL1 plasmid, and is used for transporting mannose and fructose. Some PTSs can transport multiple sugars simultaneously.121 The uptake of sugars such as pentose proceeds through non-PTS pathway; xylose and arabinose enter the cell through their specific transporters, then converge into xylose-5-phosphate through isomerisation and phosphorylation, respectively, and enter central metabolism through PPP (pentose phosphate pathway) or PK (pyruvate kinase) pathways.122
Apart from dissimilarities in uptake systems utilised for sugars, carbon catabolic repression can also lead to the consumption of sugars at different rates.123 After hydrolysis of lignocellulosic biomass, various ratios of fermentable sugars containing a mixture of pentoses and hexoses are released into the culture broth. Unfortunately, wild-type clostridia are poor at consuming mixed fermentable sugars due to CCR, and the utilisation of pentose sugars can be decreased or inhibited markedly in the presence of glucose.84 Therefore, efficient utilisation of mixtures of sugars has become a prerequisite for effective lignocellulosic butanol fermentation. To alleviate CCR in mixed sugar fermentation systems, several valuable strategies have been employed, including engineering metabolic pathways (such as knocking out ccpA to facilitate xylose and glucose utilisation124), mixed culture consolidated bioprocess,125 exogenous supplementation with trace elements126 and semi-hydrolysis strategies.127 For example, the synergistic effect of calcium and zinc was investigated in ABE fermentation by C. acetobutylicum using glucose, xylose and glucose/xylose mixtures as carbon sources. As a result, glucose/xylose utilisation, cell growth, acid re-assimilation and butanol biosynthesis were significantly improved, with butanol and ABE production increased from 11.7 and 19.4 g L−1 to 16.1 and 25.9 g L−1, respectively, from 69.3 g L−1 glucose.128 Similarly, semi-hydrolysates with low enzyme loading using H2SO4-pretreated rice straw was also employed to further improve butanol fermentation efficiency through preferential production of cellobiose and xylose (instead of glucose). As a result, butanol productivity was correspondingly increased from 0.0628 g L−1 h−1 to 0.265 g L−1 h−1 during fermentation of undetoxified semi-hydrolysates with a high cell density.127
Therefore, efficient transport and utilization of the available sugars in lignocellulosic hydrolysate without causing or alleviating the glucose carbon repression effect could be a potential exploring direction to improve the efficiency of lignocellulosic butanol production in the future.
3.3 Fermentation strain with good physiological characteristics
There are various stress responses under varying environment stresses in the process of butanol production using industrialized fermentation of lignocellulose, such as various inhibitors in the fermentation matrix after cellulose pretreatment and hydrolysis,129 intermediate metabolites (organic acids such as butyric acid and acetic acid accumulate) and fermentation end-products (accumulation of solvents such as butanol, ethanol, and acetone) during ABE fermentation,130 strains exposed to physical pressures such as inappropriate pH, temperature, dissolved oxygen and osmotic pressure due to the inhomogeneity of large-scale fermentation system, and overproduction of exogenous proteins caused by various modifications or accumulation of toxic intermediates for improving the fermentation yield or output.78 In light of it, ideal strains for butanol production by lignocellulose possess should physiological functions such as favorable adaptability and robustness for maintaining highly-active metabolic flux with good substrate and environmental tolerance of butanol during industrial fermentation.
4. Strategy for improving lignocellulosic butanol production
4.1 Improving lignocellulosic fermentation using microbial consortia or utilizing microorganisms cooperate
In lignocellulosic butanol fermentation, the complexity of the lignocellulose degradation process and the butanol metabolic pathway can significantly burden the metabolism of single strains, resulting in low butanol production using mono-cultures. In nature, lignocellulosic biomass can be entirely degraded and assimilated by microbial consortia containing fungi and cellulose-degrading bacteria.131 In this regard, natural microbial communities were mimicked to construct a synthetic ‘Y-shaped’ consortium consisting of two strains with the same butanol biosynthetic pathway and orthogonal capacity for glucose and xylose metabolism. The resulting consortium could not only adapt to environmental perturbations, but also simultaneously utilise C5/C6 sugars in different ratios, with the most efficient butanol production from mixed sugars through equally efficient orthogonal consumption of C5 and C6 sugars.132 Similarly, cellulosic butanol could be produced using anaerobic co-cultures of C. saccharoperbutylacetonicum and white-rot fungus Phlebia sp. MG-60-P2. Following knockout of pyruvate decarboxylase, co-cultures of KO77 and C. saccharoperbutylacetonicum could displayed synergistically enhanced saccharification and butanol production was further increased from 2.5 g L−1 to 3.2 g L−1 compared with co-cultures of MG-60-P2 and C. saccharoperbutylacetonicum.133 Therefore, constructing microbial consortia with the capacity for butanol synthesis and degrading lignocellulosic biomass has potential for improving lignocellulosic butanol production.
4.2 Application of advanced fermentation strategies
Following physical, chemical or biological pretreatment processes, four fermentation processes, hydrolysis and fermentation (SHF), simultaneous saccharification and fermentation (SSF), simultaneous saccharification and co-fermentation (SSCF), and the processes consolidated bioprocessing (CBP), have been applied to overcome the complex characteristics of feedstocks and feedback inhibition for lignocellulosic butanol production (Table 2).134,135
Table 2 Summary of pretreatment and detoxification techniques applied to lignocellulosic materials for ABE fermentationa
|
Pretreatment and/hydrolysis |
Detoxification |
Organism |
Fermentation conditions |
ABE, g L−1 |
Butanol, g L−1 |
Ref |
NA, data not available. |
Rice straw |
Acid hydrolysis + shear stress |
Filtering with a sterile cotton cloth |
C. acetobutylicum NCIM233 |
Batch fermentation in 2 L bioreactor |
20.56 |
13.5 |
162 |
Corn cob |
NaOH + enzymatic hydrolysis |
NA |
C. saccharobutylicum DSM 13864 |
Batch fermentation |
19.44 |
12.27 |
163 |
Corn straw |
Acid pre-impregnated steam explosion (APSE) pretreatment |
Concentrating with Rotavapor; and then lime-treated hydrolysate with Ca(OH)2 |
C. acetobutylicum ATCC 824 |
Batch fermentation |
0.31 g g−1 sugar |
0.18 g g−1 sugar |
164 |
Switchgrass |
Alkali + enzyme |
NA |
C. saccharobutylicum DSM13864 |
Batch fermentation |
22.7 |
13 |
165 |
Switchgrass |
Thermal hydrolysis pretreatment |
Without detoxification process |
C. beijerinckii ATCC 51743 |
Batch fermentations |
18.5 |
NA |
166 |
Wheat straw |
Biological treatment |
NA |
Coculture of C. beijerinckii 10132 with C. cellulovorans 35296 |
Batch fermentation |
23.30 |
14.20 |
167 |
Wheat straw |
Ammonium sulfite pretreatment |
NA |
C. acetobutylicum ATCC 824 |
Simultaneous saccharification and fermentation |
19.83 |
12.64 |
168 |
Barley straw hydrolysate |
Mechanical + acidic pretreatment |
Overliming method |
C. beijerinckii P260 |
Fermentation with product removal by gas stripping |
47.20 |
30.86 |
169 |
Mango peel waste |
Mechanical + enzyme hydrolysis |
NA |
C. acetobutylicum NCIM 2878 |
Batch fermentation |
15.13 |
10.50 |
170 |
Apple peel waste |
Mechanical |
NA |
C. acetobutylicum DSM 792 |
Batch fermentation |
20.00 |
14.00 |
171 |
Apple pomace |
Acid pretreatment + enzymatic hydrolysis |
NA |
C. beijerinckii P260 |
Batch fermentation |
10.8 |
5.6 |
19 |
Sago waste |
Acid hydrolysis + enzyme hydrolysis |
NA |
Clostridium bifermentans and Bacillus coagulans |
Batch fermentation |
9.01 |
3.36 |
172 |
Wheat red dog |
Enzymatic digests |
NA |
C. beijerinckii NCIMB 8052 |
Batch fermentation |
20.0 |
15.0 |
173 |
Spent sulfite liquor (SSL) |
NA |
Coupling nanofiltration, ultrafiltration and ion exchange |
C. acetobutylicum ATCC 824 |
Submerged fermentation |
21.09 |
12.96 |
174 |
Cellulosic material |
NA |
NA |
White-rot fungus Phlebia sp. MG-60-P2 and C. saccharoperbutylacetonicum |
Anaerobic co-culture in consolidated bioprocessing |
NA |
3.2 |
133 |
Sugarcane straw |
Microwave-assisted alkali pretreatment + acid hydrolysis |
NA |
C. beijerinkii NBRC 109359 |
Batch fermentation |
18.7 |
NA |
63 |
Industrial vinegar residue |
Steam explosion pretreatment + enzymatic hydrolysis |
NA |
C. acetobutylicum |
Batch fermentation |
12.59 |
7.98 |
175 |
Food waste |
Liquefied and saccharified |
NA |
C. saccharoperbutylacetonicum |
Continuous immobilized-cell fermentation system at dilution rate of 0.1 h−1 |
19.65 |
NA |
176 |
Yellow top (Physaria fendleri) |
Dilute acid + enzymatic hydrolysis |
NA |
C. beijerinckii P260 |
Batch fermentation |
28.8 |
NA |
177 |
Banana crop residue |
Dilute alkali and acid + enzymatic saccharification |
NA |
C. beijerinckii |
Batch fermentation |
20.5 |
14.0 |
178 |
Sugarcane bagasse |
Hemicellulosic hydrolysate |
Without detoxification process |
C. saccharoperbutylacetonicum |
Batch fermentation |
7.11 |
5.85 |
179 |
Hardwood hemicelluloses |
Hydrolysate + enzyme |
Coagulation–flocculation + inhibitors removal with bacteria |
C. acetobutylicum ATCC 824 |
Batch fermentation |
11.8 |
6.8 |
75 |
Paper pulp |
Enzymatic hydrolysis |
NA |
C. saccharoperbutylacetonicum ATCC 13564 |
Simultaneous saccharification and fermentation with in situ butanol recovery |
NA |
19.2–22.0 |
158 |
4.2.1 Simultaneous saccharification and fermentation (SSF). Compared with SHF, simultaneous saccharification and fermentation (SSF)_has fewer processing steps, lower operating costs, less contamination risk, and lower feedback inhibition of sugars on enzymes, which improves the efficiency of hydrolysis and fermentation, resulting in a higher yield of butanol.68 However, the SSF mode of operation is challenging in a number of ways: (1) during the SSF process, matching the optimum temperatures between enzymolysis (45–50 °C) and ABE fermentation (35–37 °C) is a major hurdle to achieving high cellulase activity, sugar yield, and butanol production.136,137 To this end, C. acetobutylicum with improved thermotolerance at 39–45 °C was engineered and used in high-temperature SSF of pretreated corn stover, and the optimised SSF process at 42 °C with a 12 h pre-hydrolysis yielded 10.8 g L−1 of butanol and 18.2 g L−1 of ABE from overlimited acid-treated liquid;138 (2) an imbalance in the rates of sugar formation by enzymatic hydrolysis and sugar consumption by clostridia is another challenge.139 In this regard, water-soluble cellulose oligomers have been used to modify the SSF process, in which chemically prepared water-soluble oligomers and pretreated cellulose were simultaneously subjected to enzymatic hydrolysis and ABE fermentation in a process named simultaneous co-saccharification and fermentation (SCSF).140 The SCSF process achieved a higher yield of butanol/lignocellulosic biomass (g/g), and is suited for pretreatment and enzymatic hydrolysis using lignocellulosic biomass as the substrate.141 Recently, a novel fed-batch SSFR (simultaneous saccharification, fermentation and recovery) strategy was developed and applied for ABE fermentation from alkaline-pretreated rice straw. After optimisation of initial solids and enzyme loadings by batch SSF assays, the maximum butanol concentration and butanol productivity were increased to 24.80 g L−1 and 0.344 g L−1 h−1, respectively, through three biomass feedings, showing that SSFR can significantly enhance the performance of lignocellulose fermentation when accompanied by efficient enzyme use.142
4.2.2 Consolidated bioprocesses. Recently, CBP that integrates cellulase production, lignocellulose hydrolysis, and fermentation of mixtures of sugars into a single consecutive process with a single microbe or a microbial consortium has been developed for lignocellulosic fermentation.143,144 For lignocellulosic butanol fermentation, several CBP processes have been carried out either by genetically engineering strains145–147 or co-culturing biofuel-producing strains with saccharolytic strains such as C. cellulolyticum148 and C. thermocellum.149 For example, a microbial consortium combining Thermoanaerobacterium thermosaccharolyticum M5 and C. acetobutylicum NJ4 was constructed for efficient ABE fermentation using xylan via CBP. In this study, strain M5 could efficiently degrade xylan, accumulating 19.73 g L−1 of xylose within 50 h, and strain NJ4 could efficiently utilise xylose to effectively decrease substrate inhibition on xylanase and xylosidase. As a result, the synergy of the two strains could produce 13.28 g L−1 of butanol from 70 g L−1 of xylan under optimal conditions.150
4.2.3 Two-stage and multi-stage fermentation. Compared with batch fermentation, single-stage continuous fermentation can achieve high solvent productivity, but lower solvent concentrations, indicating that single-stage continuous fermentation is not suitable for industrial-scale applications.151 However, a continuous bioreactor with immobilised cells and multistage fermentation has been applied for continuous ABE fermentation.152 Two-stage and multi-stage fermentation systems can completely utilise substrates and effectively assimilate acids to solvents, achieving increased productivity and solvent concentrations over long-term fermentation.152 For example, a four-stage continuous fermentation with cane molasses was employed to enhance butanol titre and productivity, achieving 13.75 g L−1 of solvents with a productivity of 0.439 g L−1 h−1 with a gradient dilution mode of 0.15–0.15–0.125–0.1 h−1.153 In addition, a novel two-stage fermentation process, comprising an acidogenic fermentation process followed by an ABE fermentation process, was also developed and introduced to maximise sugar utilisation and butanol yield with alkaline-pretreated rice straw.154 A sugar-rich hydrolysate (90.4 g L−1 reducing sugar) and a high acid level in the fermentation broth (33.9 g L−1 butyric acid) were obtained. Also, final butanol production was significantly increased to 15.9 g L−1 with 3-fold lower cellulase loading, yielding 149 g butanol and 36 L hydrogen gas from 1 kg rice straw, respectively.154
4.2.4 Improving fermentation processes for lignocellulosic butanol. In general, pretreatment processes have three main drawbacks:155 (1) requiring specific instruments and facilities to pretreat lignocellulosic materials under strict conditions; (2) the formation and release of inhibitors that negatively impact enzymatic hydrolysis and fermentation, resulting in the need for an additional detoxification step, which increases total costs;156 (3) using ‘non-green’ chemicals as catalysts in several pretreatment methods, with consequent negative environmental impacts.157 To overcome these drawbacks, a ‘semi-hydrolysis’ strategy was proposed to utilise lignocellulosic material containing oligosaccharides and monosaccharides using less hydrolytic enzyme, then further improving the butanol yield, enzyme loading, and overall butanol productivity.127 For example, a consolidated fermentation system was established in which enzymatic semi-hydrolysis of paper pulp (93.2% glucan) without pretreatment and with low enzyme loading produced high levels of cellobiose (13.9 g L−1) and glucose (21.3 g L−1) via ABE fermentation, which was as efficient as fermentation using commercial sugars without inhibitors.158Furthermore, physical, chemical or biological detoxification methods have been developed for eliminating toxic inhibitors, but the resulting wastewater, increased energy costs, and sugar loss remain barriers to economic viability and environmental sustainability at industrial scale.17,159 Therefore, mutagenesis, genetic manipulation and metabolic perturbations have been applied to construct inhibitor-tolerating strains that can achieve lignocellulosic butanol fermentation without detoxification or wastewater generation. For example, a mutant overexpressing PTSGlcG exhibited improved inhibitor tolerance, and produced 10.1 g L−1 of butanol using corn stover hydrolysate (CSH) culture without detoxification, an increase by 300% and 400% compared with 2.5 and 2.0 g L−1 achieved control and PTSGlcG-deficient strains, respectively.160 Similarly, introduction of a ‘push–pull’ strategy in C. cellulovorans DSM 743B diverted carbon flux from acetyl-CoA to butyryl-CoA by overexpressing a trans-enoyl-coenzyme A reductase gene (ter), and an acid re-assimilation pathway uncoupled from acetone synthesis was also constructed to redirect carbon flux from butyrate and acetate toward butyryl-CoA. Xylose metabolism was engineered by overexpressing xylT (CA_C1345) and inactivating xylR (Clocel_0594) and araR (Clocel_1253), and final production of butanol was increased to 4.96 g L−1 directly from alkali-extracted corncobs.161
4.3 A strategy for engineering physiological functionalities
Good physiological performance of microbes is crucial for successful biological fermentation. Strain improvement through physiological engineering relies on integration of knowledge on physiological functionality and efficient engineering approaches.130 For example, introduction of pro (precursor region)–mtg (microbial transglutaminase) into C. acetobutylicum improves oxidative-stress resistance, growth performance, and solvent production of the host.180 In the past, many strains obtained by metabolic engineering strategies failed to steady exhibit the expected phenotype because the physiological stress responses of microorganisms were ignored. Therefore, microorganisms should not only have strong metabolic capacity, but also possess strong physiological robustness and adaptability in order to work effectively in actual biological processes.
5. Future prospects
Clostridial species generally perform ABE fermentation using the cellulose and hemicellulose fraction of lignocellulosic feedstocks.181 However, essential pretreatment steps limit the fermentation of lignocellulosic biomass, accounting for a large portion of the process cost. Therefore, it is crucial to develop more cost-efficient pretreatment processes that minimise the formation of inhibitors, lower energy consumption, diminish operating costs, and concurrently maximise fermentable sugar production with careful consideration of feedstock properties.76 Carbon loss can occur in the form of xylose during biomass pretreatment, and a large amount of carbon in the form of CO2 is emitted during fermentation and co-generation processes.182,183 An innovative way to valorise off-gas streams is to produce alcohols through gas fermentation, in which acetogens ferment CO2 plus H2 to produce VFAs (volatile fatty acids) and alcohols via the Wood–Ljungdahl pathway, as demonstrated with Clostridium carboxidivorans.184–186 Furthermore, effective utilisation of xylose in hemicellulose is also a key factor to reduce the production cost of biobutanol. To increase the overall competitiveness of lignocellulosic butanol, cost-effective and industrially feasible strategies for lignocellulosic biomass pretreatment and hydrolysis must be further optimised. Furthermore, an ideal lignocellulosic butanol-producing strain is also needed to maintain high butanol metabolic flux activity, efficient sugar transport, the use of fermentable mixed sugar, and good substrate tolerance in the process of industrial butanol fermentation, which can further improve the fermentation performance and lower fermentation costs.
The performance of lignocellulosic fermentation could be improved as follows:
• Process integration and intensification measures to further improve the performance and economic competitiveness of lignocellulosic butanol fermentation, such as integration of (1) pretreatment with hydrolysis, (2) hydrolysis with fermentation, and even (3) hydrolysis, fermentation, and product recovery.
• Adapting and improving the physiological characteristics of target microorganisms: it can achieve rapid transformation of hexose and pentose; good tolerance to the target product butanol, the intermediate product butyric acid, and inhibitors in raw materials; under low pH stress conditions, and in the presence of organic acids and butanol, the metabolic activity can remain high; good metabolic flux can be maintained throughout the fermentation process.
• Production of butanol by lignocellulose is so complicated that might lead to unnecessary cell stress. In that case, the toxicity problem of the butanol fermentation process and the heterogeneity of industrial production should be considered although it is essential to orient at breaking through a single problem. More precisely, lignocellulose pretreatment, microbial physiological process and characteristics, carbon efficient utilization or butanol recovery technology should be adopted to improve the physiologic performance of microbes, achieving both the enhanced yield and stable output in conjunction with interleaving functions of biological robustness.
Conflicts of interest
The authors declare that they have no competing interests.
Acknowledgements
This research was financially supported by the Key Project of Guangxi Scientific Research and Technology Development Plan (AB17190534), the Natural Science Foundation of Guangxi (GXNSFAA259007), and the Basic Operating Expenses of Guangxi Academy of Sciences (2019YJJ1001).
References
- A. C. dos Santos, et al., Lignin-Enzyme Interactions in the Hydrolysis of Lignocellulosic Biomass, Trends Biotechnol., 2019, 37(5), 518–531 CrossRef CAS PubMed.
- F. Cebreiros, M. D. Ferrari and C. Lareo, Cellulose hydrolysis and IBE fermentation of eucalyptus sawdust for enhanced biobutanol production by Clostridium beijerinckii DSM 6423, Ind. Crops Prod., 2019, 134, 50–61 CrossRef CAS.
- Y. J. Jiang, et al., Clostridium sp. Strain NJ4: A Promising Solventogenic Strain for Butanol Production from Jerusalem Artichoke through Consolidated Bioprocessing, Energy Fuels, 2020, 34(3), 3406–3411 CrossRef CAS.
- Y. Jiang, et al., Current status and prospects of industrial bio-production of n-butanol in China, Biotechnol. Adv., 2015, 33(7), 1493–1501 CrossRef CAS PubMed.
- N. K. N. Al-Shorgani, et al., Continuous butanol fermentation of dilute acid-pretreated de-oiled rice bran by Clostridium acetobutylicum YM1, Sci. Rep., 2019, 9, 4622 CrossRef PubMed.
- C. Xue, et al., Recent advances and state-of-the-art strategies in strain and process engineering for biobutanol production by Clostridium acetobutylicum, Biotechnol. Adv., 2017, 35(2), 310–322 CrossRef CAS PubMed.
- M. Rastogi and S. Shrivastava, Recent advances in second generation bioethanol production: An insight to pretreatment, saccharification and fermentation processes, Renewable Sustainable Energy Rev., 2017, 80, 330–340 CrossRef.
- H. Amiri, K. Karimi and H. Zilouei, Organosolv pretreatment of rice straw for efficient acetone, butanol, and ethanol production, Bioresour. Technol., 2014, 152, 450–456 CrossRef CAS.
- J. Zhang, et al., Efficient acetone-butanol-ethanol production from corncob with a new pretreatment technology-wet disk milling, Bioenergy Res., 2013, 6(1), 35–43 CrossRef CAS.
- N. Qureshi, B. C. Saha and M. A. Cotta, Butanol production from wheat straw hydrolysate using Clostridium beijerinckii, Bioprocess Biosyst. Eng., 2007, 30(6), 419–427 CrossRef CAS.
- N. Qureshi, et al., Production of butanol (a biofuel) from agricultural residues: Part I - Use of barley straw hydrolysate, Biomass Bioenergy, 2010, 34(4), 559–565 CrossRef CAS.
- Y. Jafari, K. Karimi and H. Amiri, Efficient bioconversion of whole sweet sorghum plant to acetone, butanol, and ethanol improved by acetone delignification, J. Cleaner Prod., 2017, 166, 1428–1437 CrossRef CAS.
- H. Amiri and K. Karimi, Autohydrolysis: A promising pretreatment for the improvement of acetone, butanol, and ethanol production from woody materials, Chem. Eng. Sci., 2015, 137, 722–729 CrossRef CAS.
- A. Abedini, H. Amiri and K. Karimi, Efficient biobutanol production from potato peel wastes by separate and simultaneous inhibitors removal and pretreatment, Renewable Energy, 2020, 160, 269–277 CrossRef CAS.
- R. R. Mishra, et al., Process optimization for conversion of waste banana peels to biobutanol by a yeast co-culture fermentation system, Renewable Energy, 2020, 162, 478–488 CrossRef CAS.
- K. M. Schwarz, et al., Towards improved butanol production through targeted genetic modification of Clostridium pasteurianum, Metab. Eng., 2017, 40, 124–137 CrossRef CAS PubMed.
- N. R. Baral and A. Shah, Microbial inhibitors: formation and effects on acetone-butanol-ethanol fermentation of lignocellulosic biomass, Appl. Microbiol. Biotechnol., 2014, 98(22), 9151–9172 CrossRef CAS PubMed.
- C. Xlab, et al., High-efficient cellulosic butanol production from deep eutectic solvent pretreated corn stover without detoxification, Ind. Crops Prod., 2021, 162 DOI:10.1016/j.indcrop.2021.113258.
- Q. Jin, et al., Acetone-butanol-ethanol (ABE) fermentation of soluble and hydrolyzed sugars in apple pomace by Clostridium beijerinckii P260, Fuel, 2019, 244, 536–544 CrossRef CAS.
- B. Pratto, et al., Biobutanol production from sugarcane straw: Defining optimal biomass loading for improved ABE fermentation, Ind. Crops Prod., 2020, 148 DOI:10.1016/j.indcrop.2020.112265.
- L. D. Gottumukkala, K. Haigh and J. Gorgens, Trends and advances in conversion of lignocellulosic biomass to biobutanol: Microbes, bioprocesses and industrial viability, Renewable Sustainable Energy Rev., 2017, 76, 963–973 CrossRef CAS.
- Y. Wang, et al., Current advances on fermentative biobutanol production using third generation feedstock, Biotechnol. Adv., 2017, 35(8), 1049–1059 CrossRef CAS.
- Y. Yang, et al., Metabolic regulation in solventogenic Clostridia: regulators, mechanisms and engineering, Biotechnol. Adv., 2018, 36(4), 905–914 CrossRef CAS.
- B. Bharathiraja, J. Jayamuthunagai, T. Sudharsanaa, A. Bharghavi, R. Praveenkumar, M. Chakravarthy and D. Yuvaraj, et al., Biobutanol - An impending biofuel for future: A review on upstream and downstream processing techniques, Renewable Sustainable Energy Rev., 2017, 68(1), 788–807 CrossRef.
- K. Dutta, A. Daverey and J. G. Lin, Evolution retrospective for alternative fuels: First to fourth generation, Renewable Energy, 2014, 69, 114–122 CrossRef CAS.
- Y. N. Zheng, et al., Problems with the microbial production of butanol, J. Ind. Microbiol. Biotechnol., 2009, 36(9), 1127–1138 CrossRef CAS PubMed.
- P. Dürre, et al., Fermentative butanol production: bulk chemical and biofuel, Ann. N. Y. Acad. Sci., 2008, 1125(1), 353 CrossRef PubMed.
- J. Nölling, et al., Genome sequence and comparative analysis of the solvent-producing bacterium Clostridium acetobutylicum, J. Bacteriol., 2001, 183(16), 4823–4838 CrossRef PubMed.
- G. H. Bao, et al., Complete Genome Sequence of Clostridium acetobutylicum DSM 1731, a Solvent-Producing Strain with Multireplicon Genome Architecture, J. Bacteriol., 2011, 193(18), 5007–5008 CrossRef CAS PubMed.
- S. Hu, et al., Comparative genomic and transcriptomic analysis revealed genetic characteristics related to solvent formation and xylose utilization in Clostridium acetobutylicum EA 2018, BMC Genomics, 2011, 12(1), 93 CrossRef CAS PubMed.
- G. Gottschalk, Regulation of Bacterial Metabolism, Springer-Verlag, 1986 Search PubMed.
- E. R. Kashket and J. S. Terracciano, Intracellular Conditions Required for Initiation of Solvent Production by Clostridium acetobutylicum, Appl. Environ. Microbiol., 1986, 52(1), 86–91 CrossRef PubMed.
- F. Monot, J. M. Engasser and H. Petit De Mange, Influence of pH and undissociated butyric acid on the production of acetone and butanol in batch cultures of Clostridium acetobutylicum, Appl. Microbiol. Biotechnol., 1984, 19(6), 422–426 CrossRef CAS.
- J. B. Russell, Another explanation for the toxicity of fermentation acids at low pH: Anion accumulation versus uncoupling, J. Appl. Microbiol., 2008, 73(5), 363–370 Search PubMed.
- A. P. Mariano, et al., Assessment of in situ butanol recovery by vacuum during acetone butanol ethanol (ABE) fermentation, J. Chem. Technol. Biotechnol., 2012, 87(3), 334–340 CrossRef CAS.
- J. Liu, et al., Enhanced butanol production by increasing NADH and ATP levels in Clostridium beijerinckii NCIMB 8052 by insertional inactivation of Cbei_4110, Appl. Microbiol. Biotechnol., 2016, 100(11), 4985–4996 CrossRef CAS PubMed.
- Y. Gu, et al., Utilization of economical substrate-derived carbohydrates by solventogenic Clostridia: pathway dissection, regulation and engineering, Curr. Opin. Biotechnol., 2014, 29, 124–131 CrossRef CAS.
- S. B. Li, et al., Pathway dissection, regulation, engineering and application: lessons learned from biobutanol production by solventogenic Clostridia, Biotechnol. Biofuels, 2020, 13(1), 39 CrossRef CAS PubMed.
- A. Sawisit, et al., Optimization of sodium hydroxide pretreatment and enzyme loading for efficient hydrolysis of rice straw to improve succinate production by metabolically engineered Escherichia coli KJ122 under simultaneous saccharification and fermentation, Bioresour. Technol., 2018, 260, 348–356 CrossRef CAS PubMed.
- S. H. Han, et al., Biobutanol production from 2-year-old willow biomass by acid hydrolysis and acetone-butanol-ethanol fermentation, Energy, 2013, 61, 13–17 CrossRef CAS.
- S. Shanmugam, et al., Enhanced bioconversion of hemicellulosic biomass by microbial consortium for biobutanol production with bioaugmentation strategy, Bioresour. Technol., 2019, 279, 149–155 CrossRef CAS PubMed.
- S. Fatma, et al., Lignocellulosic Biomass: A Sustainable Bioenergy Source for the Future, Protein Pept. Lett., 2018, 25(2), 148–163 CrossRef CAS PubMed.
- K. Karimi, M. Shafiei and R. Kumar, Progress on physical and chemical pretreatment of lignocellulosic biomass, Springer, Berlin, Heidelberg, 2013, pp. 53–96 Search PubMed.
- C. Birgen, et al., Butanol production from lignocellulosic biomass: revisiting fermentation performance indicators with exploratory data analysis, Biotechnol. Biofuels, 2019, 12, 167 CrossRef CAS PubMed.
- Y. R. Wu, et al., Synergistic enzymatic saccharification and fermentation of agar for biohydrogen production, Bioresour. Technol., 2017, 241, 369–373 CrossRef CAS PubMed.
- M. Herbaut, et al., Multimodal analysis of pretreated biomass species highlights generic markers of lignocellulose recalcitrance, Biotechnol. Biofuels, 2018, 11, 52 CrossRef PubMed.
- S. Sombatpraiwan, et al., Optimization of microwave-assisted alkali pretreatment of cassava rhizome for enhanced enzymatic hydrolysis glucose yield, Food and Energy Security, 2019, 8(4), e00174 CrossRef.
- C. L. Cheng, et al., Biobutanol production from agricultural waste by an acclimated mixed bacterial microflora, Appl. Energy, 2012, 100, 3–9 CrossRef CAS.
- A. K. Kumar and S. Sharma, Recent updates on different methods of pretreatment of lignocellulosic feedstocks: a review, Bioresour. Bioprocess., 2017, 4, 7 CrossRef PubMed.
- X. Kong, et al., Biobutanol production from sugarcane bagasse hydrolysate generated with the assistance of gamma-valerolactone, Process Biochem., 2016, 51(10), 1538–1543 CrossRef CAS.
- H. Li, et al., Enhanced enzymatic hydrolysis and acetone-butanol-ethanol fermentation of sugarcane bagasse by combined diluted acid with oxidate ammonolysis pretreatment, Bioresour. Technol., 2017, 228, 257–263 CrossRef CAS PubMed.
- C. Tang, et al., Sustainable biobutanol production using alkali-catalyzed organosolv pretreated cornstalks, Ind. Crops Prod., 2017, 95, 383–392 CrossRef CAS.
- H. Su, et al., A biorefining process: Sequential, combinational lignocellulose pretreatment procedure for improving biobutanol production from sugarcane bagasse, Bioresour. Technol., 2015, 187, 149–160 CrossRef CAS PubMed.
- K. Liu, et al., Butanol production from hydrothermolysis-pretreated switchgrass: Quantification of inhibitors and detoxification of hydrolyzate, Bioresour. Technol., 2015, 189, 292–301 CrossRef CAS PubMed.
- S. K. Bhatia, et al., Current status and strategies for second generation biofuel production using microbial systems, Energy Convers. Manage., 2017, 148, 1142–1156 CrossRef CAS.
- H. Shukor, et al., Enhanced mannan-derived fermentable sugars of palm kernel cake by mannanase-catalyzed hydrolysis for production of biobutanol, Bioresour. Technol., 2016, 218, 257–264 CrossRef CAS PubMed.
- T. Bao, et al., n-Butanol and ethanol production from cellulose by Clostridium cellulovorans overexpressing heterologous aldehyde/alcohol dehydrogenases., Bioresour. Technol., 2019, 285, 121316 CrossRef CAS PubMed.
- Y. T. Tan, A. S. M. Chua and G. C. Ngoh, Deep eutectic solvent for lignocellulosic biomass fractionation and the subsequent conversion to bio-based products - A review, Bioresour. Technol., 2020, 297 DOI:10.1016/j.biortech.2019.122522.
- H. Xu, et al., Key process parameters for deep eutectic solvents pretreatment of lignocellulosic biomass materials: A review, Bioresour. Technol., 2020, 310, 123416 CrossRef CAS PubMed.
- X. Q. Lin, et al., High-efficient cellulosic butanol production from deep eutectic solvent pretreated corn stover without detoxification, Ind. Crops Prod., 2021, 162, 113258 CrossRef CAS.
- A. Procentese, et al., Pre-treatment and enzymatic hydrolysis of lettuce residues as feedstock for bio-butanol production, Biomass Bioenergy, 2017, 96, 172–179 CrossRef CAS.
- K. R. Corbin, et al., Grape marc as a source of carbohydrates for bioethanol: Chemical composition, pre-treatment and saccharification, Bioresour. Technol., 2015, 193, 76–83 CrossRef CAS.
- A. Karungi, A. Pogrebnoi and T. Kivevele, Optimization of microwave-assisted alkali pretreatment followed by acid hydrolysis of sugarcane straw for production of acetone-butanol-ethanol, Energy Sources, Part A, 2020 DOI:10.1080/15567036.2020.1760404.
- M. O. Jang and G. Choi, Techno-economic analysis of butanol production from lignocellulosic biomass by concentrated acid pretreatment and hydrolysis plus continuous fermentation, Biochem. Eng. J., 2018, 134, 30–43 CrossRef CAS.
- B. Branska, et al., Chicken feather and wheat straw hydrolysate for direct utilization in biobutanol production, Renewable energy, 2020, 145, 1941–1948 CrossRef CAS.
- B. Zghari, L. Hajji and B. Abdellatif, Effect of Moist and Dry Heat Weathering Conditions on Cellulose Degradation of Historical Manuscripts exposed to Accelerated Ageing: 13C NMR and FTIR Spectroscopy as a non-Invasive Monitoring Approach, J. Mater. Environ. Sci., 2018, 9(2), 641–654 Search PubMed.
- W. Wang, et al., Study on the decreased sugar yield in enzymatic hydrolysis of cellulosic substrate at high solid loading, Appl. Biochem. Biotechnol., 2011, 164(7), 1139–1149 CrossRef CAS PubMed.
- H. Amiri and K. Karimi, Pretreatment and hydrolysis of lignocellulosic wastes for butanol production: Challenges and perspectives, Bioresour. Technol., 2018, 270, 702–721 CrossRef CAS PubMed.
- M. Mirfakhar, et al., Co-fermentation of hemicellulosic hydrolysates and starch from sweet sorghum by Clostridium acetobutylicum: A synergistic effect for butanol production, Ind. Crops Prod., 2020, 151, 112459 CrossRef CAS.
- S. A. Survase, et al., Continuous acetone-butanol-ethanol fermentation using SO2-ethanol-water spent liquor from spruce, Bioresour. Technol., 2011, 102(23), 10996–11002 CrossRef CAS PubMed.
- E. Wyman Charles, Aqueous Pretreatment of Plant Biomass for Biological and Chemical Conversion to Fuels and Chemicals (Wyman/Aqueous Pretreatment of Plant Biomass for Biological and Chemical Conversion to Fuels and Chemicals), Laboratory Pretreatment Systems to Understand, 2013, pp. 489–521, DOI:10.1002/9780470975831.
- N. Qureshi, et al., Production of butanol (a biofuel) from agricultural residues: Part II - Use of corn stover and switchgrass hydrolysates, Biomass Bioenergy, 2010, 34(4), 566–571 CrossRef CAS.
- A. Ranjan and V. S. Moholkar, Comparative study of various pretreatment techniques for rice straw saccharification for the production of alcoholic biofuels, Fuel, 2013, 112, 567–571 CrossRef CAS.
- Z. J. Sun and S. J. Liu, Production of n-butanol from concentrated sugar maple hemicellulosic hydrolysate by Clostridia acetobutylicum ATCC824, Biomass Bioenergy, 2012, 39, 39–47 CrossRef CAS.
- M. Theiri, et al., Development of sequential and simultaneous bacterial cultures to hydrolyse and detoxify wood pre-hydrolysate for enhanced acetone-butanol-ethanol (ABE) production, Enzyme Microb. Technol., 2020, 133, 109438 CrossRef CAS PubMed.
- J. X. Zhang, et al., Recent developments in ionic liquid pretreatment of lignocellulosic biomass for enhanced bioconversion, Sustainable Energy Fuels, 2021, 5(6), 1655–1667 RSC.
- Y. Zhang, et al., The importance of engineering physiological functionality into microbes, Trends Biotechnol., 2009, 27(12), 664–672 CrossRef CAS PubMed.
- M. T. Mohedano, O. Konzock and Y. Chen, Strategies to increase tolerance and robustness of industrial microorganisms, Synth. Syst. Biotechnol., 2022, 7(1), 533–540 CrossRef CAS PubMed.
- M. S. Pino, et al., Bioreactor design for enzymatic hydrolysis of biomass under the biorefinery concept, Chem. Eng. J., 2018, 347, 119–136 CrossRef CAS.
- I. S. Maddox, et al., Production of acetone-butanol-ethanol from concentrated substrate using clostridium acetobutylicum in an integrated fermentation-product removal process - ScienceDirect, Process Biochem., 1995, 30(3), 209–215 CAS.
- P. Narueworanon, et al., Impacts of initial sugar, nitrogen and calcium carbonate on butanol fermentation from sugarcane molasses by Clostridium beijerinckii, Energies, 2020, 13(3), 1–19 CrossRef.
- H. Amiri and K. Karimi, Integration of autohydrolysis and organosolv delignification for efficient acetone, butanol, and ethanol production and lignin recovery, Ind. Eng. Chem. Res., 2016, 55(17), 4836–4845 CrossRef CAS.
- M. Yang, et al., Enhanced acetone-butanol-ethanol production from lignocellulosic hydrolysates by using starchy slurry as supplement, Bioresour. Technol., 2017, 243, 126–134 CrossRef CAS PubMed.
- C. Ren, et al., Identification and inactivation of pleiotropic regulator CcpA to eliminate glucose repression of xylose utilization in Clostridium acetobutylicum, Metab. Eng., 2010, 12(5), 446–454 CrossRef CAS PubMed.
- C. Lu, et al., Fed-batch fermentation for n-butanol production from cassava bagasse hydrolysate in a fibrous bed bioreactor with continuous gas stripping, Bioresour. Technol., 2012, 104, 380–387 CrossRef CAS PubMed.
- H. Z. Luo, et al., Recent advances and strategies in process and strain engineering for the production of butyric acid by microbial fermentation, Bioresour. Technol., 2018, 253, 343–354 CrossRef CAS PubMed.
- H. H. Liu, et al., Omics-based analyses revealed metabolic responses of Clostridium acetobutylicum to lignocellulose-derived inhibitors furfural, formic acid and phenol stress for butanol fermentation, Biotechnol. Biofuels, 2019, 12, 101 CrossRef PubMed.
- E. Palmqvist and B. Hahn-Hagerdal, Fermentation of lignocellulosic hydrolysates. II: inhibitors and mechanisms of inhibition, Bioresour. Technol., 2000, 74(1), 25–33 CrossRef CAS.
- D. H. Cho, S. J. Shin and Y. H. Kim, Effects of acetic and formic acid on ABE production by Clostridium acetobutylicum and Clostridium beijerinckii, Biotechnol. Bioprocess Eng., 2012, 17(2), 270–275 CrossRef CAS.
- S. H. Wang, et al., Formic Acid Triggers the "Acid Crash" of Acetone-Butanol-Ethanol Fermentation by Clostridium acetobutylicum, Appl. Environ. Microbiol., 2011, 77(5), 1674–1680 CrossRef CAS PubMed.
- T. Ezeji, N. Qureshi and H. P. Blaschek, Butanol production from agricultural residues: Impact of degradation products on Clostridium beijerinckii growth and butanol fermentation, Biotechnol. Bioeng., 2007, 97(6), 1460–1469 CrossRef CAS PubMed.
- N. Qureshi, et al., Effect of cellulosic sugar degradation products (furfural and hydroxymethyl furfural) on acetone–butanol–ethanol (ABE) fermentation using Clostridium beijerinckii P260, Food Bioprod. Process., 2012, 90(3), 533–540 CrossRef CAS.
- Z. Yan, H. Bei and T. C. Ezeji, Biotransformation of furfural and 5-hydroxymethyl furfural (HMF) by Clostridium acetobutylicum ATCC 824 during butanol fermentation, New Biotechnol., 2012, 29(3), 345–351 CrossRef PubMed.
- S. Maiti, A re-look at the biochemical strategies to enhance butanol production, Biomass Bioenergy, 2016, 94, 187–200 CrossRef CAS.
- W. H. Chen and Y. R. Zeng, Mathematical model to appraise the inhibitory effect of phenolic compounds derived from lignin for biobutanol production, Bioresour. Technol., 2018, 261, 44–51 CrossRef CAS PubMed.
- H. Q. Gu, et al., Physiological mechanism of improved tolerance of Saccharomyces cerevisiae to lignin-derived phenolic acids in lignocellulosic ethanol fermentation by short-term adaptation, Biotechnol. Biofuels, 2019, 12(1), 268 CrossRef PubMed.
- T. Zhao, Y. Tashiro and K. Sonomoto, Smart fermentation engineering for butanol production: designed biomass and consolidated bioprocessing systems, Appl. Microbiol. Biotechnol., 2019, 103(23–24), 9359–9371 CrossRef CAS PubMed.
- H. Z. Luo, et al., Co-production of solvents and organic acids in butanol fermentation by Clostridium acetobutylicum in the presence of lignin-derived phenolics, RSC Adv., 2019, 9(12), 6919–6927 RSC.
- C. F. D. Vieira, et al., Isopropanol-butanol-ethanol (IBE) production in repeated-batch cultivation of Clostridium beijerinckii DSM 6423 immobilized on sugarcane bagasse, Fuel, 2020, 263, 116708 CrossRef.
- S. Lee, J. H. Lee and R. J. Mitchell, Analysis of Clostridium beijerinckii NCIMB 8052’s transcriptional response to ferulic acid and its application to enhance the strain tolerance, Biotechnol. Biofuels, 2015, 8, 68 CrossRef PubMed.
- M. P. Raut, et al., Quantitative proteomic analysis of the influence of lignin on biofuel production by Clostridium acetobutylicum ATCC 824, Biotechnol. Biofuels, 2016, 9, 113 CrossRef PubMed.
- S. A. Survase, et al., Efficient strategy to alleviate the inhibitory effect of lignin-derived compounds for enhanced butanol production, ACS Sustainable Chem. Eng., 2021, 9(3), 1172–1179 CrossRef CAS.
- L. J. Jönsson, Bioconversion of lignocellulose: inhibitors and detoxification, Biotechnol. Biofuels, 2013, 6, 16 CrossRef PubMed.
- L. Encina, et al., Hydrogels derived from galactoglucomannan hemicellulose with inorganic contaminant removal properties, RSC Adv., 2021, 11(57), 35960–35972 RSC.
- Z. Xiao, et al., Effects of sugar inhibition on cellulases and β-glucosidase during enzymatic hydrolysis of softwood substrates, Appl. Biochem. Biotechnol., 2004, 116(1–3), 1115–1126 CrossRef.
- M. Galbe and G. Zacchi, Pretreatment of Lignocellulosic Materials for Efficient Bioethanol Production, Adv. Biochem. Eng./Biotechnol., 2007, 108, 41–65 CrossRef CAS PubMed.
- V. B. Barua, V. V. Goud and A. S. Kalamdhad, Microbial pretreatment of water hyacinth for enhanced hydrolysis followed by biogas production, Renewable Energy, 2018, 126, 21–29 CrossRef CAS.
- S. Maiti, et al., Hydrolytic pre-treatment methods for enhanced biobutanol production from agro-industrial wastes, Bioresour. Technol., 2018, 249, 673–683 CrossRef CAS PubMed.
- Y. J. Jiang, et al., Consolidated bioprocessing of butanol production from xylan by a thermophilic and butanologenic Thermoanaerobacterium sp. M5, Biotechnol. Biofuels, 2018, 11, 89 CrossRef PubMed.
- N. Qureshi, et al., Removal of fermentation inhibitors from alkaline peroxide pretreated and enzymatically hydrolyzed wheat straw: Production of butanol from hydrolysate using Clostridium beijerinckii in batch reactors, Biomass Bioenergy, 2008, 32(12), 1353–1358 CrossRef CAS.
- A. Boonsombuti, et al., Enhancement of ABE fermentation through regulation of ammonium acetate and D–xylose uptake from acid-pretreated corncobs, Ann. Microbiol., 2014, 64, 431–439 CrossRef CAS.
- L. Yu, et al., Metabolic engineering of Clostridium tyrobutyricum for n-butanol production through co-utilization of glucose and xylose, Biotechnol. Bioeng., 2015, 112(10), 2134–2141 CrossRef CAS PubMed.
- V. H. G. Diaz and G. O. Tost, Economic optimization of in situ extraction of inhibitors in acetone-ethanol-butanol (ABE) fermentation from lignocellulose, Process Biochem., 2018, 70, 1–8 CrossRef CAS.
- L. D. Gottumukkala, et al., Biobutanol production from rice straw by a non acetone producing Clostridium sporogenes BE01, Bioresour. Technol., 2013, 145, 182–187 CrossRef CAS PubMed.
- L. J. Jonsson and C. Martin, Pretreatment of lignocellulose: Formation of inhibitory by-products and strategies for minimizing their effects, Bioresour. Technol., 2016, 199, 103–112 CrossRef PubMed.
- S. S. Hassan, G. A. Williams and A. K. Jaiswal, Emerging technologies for the pretreatment of lignocellulosic
biomass, Bioresour. Technol., 2018, 262, 310–318 CrossRef CAS PubMed.
- N. Qureshi, et al., Cellulosic butanol (ABE) biofuel production from sweet sorghum bagasse (SSB): impact of hot water pretreatment and solid loadings on fermentation employing Clostridium beijerinckii P260, BioEnergy Res., 2016, 9(4), 1167–1179 CrossRef CAS.
- L. Zhang, et al., Ribulokinase and transcriptional regulation of arabinose metabolism in Clostridium acetobutylicum, J. Bacteriol., 2012, 194(5), 1055–1064 CrossRef CAS PubMed.
- J. Lee, et al., Evidence for the presence of an alternative glucose transport system in Clostridium beijerinckii NCIMB 8052 and the solvent-hyperproducing mutant BA101, Appl. Environ. Microbiol., 2005, 71(6), 3384–3387 CrossRef CAS PubMed.
- C. Voigt, H. Bahl and R. J. Fischer, Identification of PTS(Fru) as the major fructose uptake system of Clostridium acetobutylicum, Appl. Microbiol. Biotechnol., 2014, 98(16), 7161–7172 CrossRef CAS PubMed.
- W. Mitchell and M. Tangney, Carbohydrate uptake by the phosphotransferase system and other mechanisms, Handbook on Clostridia, 2005 Search PubMed.
- Z. Wen, et al., Recent advances in metabolic engineering of clostridia for n-butanol production, Synth. Biol. J., 2021, 2(2), 194–221 Search PubMed.
- A. Hamid and K. Keikhosro, Pretreatment and Hydrolysis of Lignocellulosic Wastes for Butanol Production: Challenges and Perspectives, Bioresour. Technol., 2018, 270, S0960852418312215 Search PubMed.
- M. Yang, et al., Co-fermentation of hemicellulose and starch from barley straw and grain for efficient pentoses utilization in acetone-butanol-ethanol production, Bioresour. Technol., 2015, 179, 128–135 CrossRef CAS PubMed.
- Y. Chen, et al., Production of butanol from glucose and xylose with immobilized cells of Clostridium acetobutylicum, Biotechnol. Bioprocess Eng., 2013, 18(2), 234–241 CrossRef CAS.
- Y. D. Wu, et al., Synergistic effect of calcium and zinc on glucose/xylose utilization and butanol tolerance of Clostridium acetobutylicum, FEMS Microbiol. Lett., 2016, 363(5), fnw023 CrossRef PubMed.
- T. Zhao, et al., Semi-hydrolysis with low enzyme loading leads to highly effective butanol fermentation, Bioresour. Technol., 2018, 264, 335–342 CrossRef CAS PubMed.
- Y. D. Wu, et al., Impact of zinc supplementation on the improved fructose/xylose utilization and butanol production during acetone-butanol-ethanol fermentation, J. Biosci. Bioeng., 2016, 121(1), 66–72 CrossRef CAS PubMed.
- X. Zhao, et al., A quantitative metabolomics study of high sodium response in Clostridium acetobutylicum ATCC 824 acetone-butanol-ethanol (ABE) fermentation, Sci. Rep., 2016, 6, 28307 CrossRef CAS PubMed.
- Y. Zhang, Y. Zhu, Y. Zhu and Y. Li, The importance of engineering physiological functionality into microbes, Trends Biotechnol., 2009, 27(12), 664–672 CrossRef CAS PubMed.
- G. de Gonzalo, et al., Bacterial enzymes involved in lignin degradation, J. Biotechnol., 2016, 236, 110–119 CrossRef CAS PubMed.
- C. H. Zhao, et al., Design and development of a “Y-shaped” microbial consortium capable of simultaneously utilizing biomass sugars for efficient production of butanol, Metab. Eng., 2019, 55, 111–119 CrossRef CAS PubMed.
- C. L. Tri and I. Kamei, Butanol production from cellulosic material by anaerobic co-culture of white-rot fungus Phlebia and bacterium Clostridium in consolidated bioprocessing, Bioresour. Technol., 2020, 305, 123065 CrossRef CAS PubMed.
- D. Haldar and M. K. Purkait, Lignocellulosic conversion into value-added products: A review, Process Biochem., 2020, 89, 110–133 CrossRef CAS.
- V. Parisutham, T. H. Kim and S. K. Lee, Feasibilities of consolidated bioprocessing microbes: From pretreatment to biofuel production, Bioresour. Technol., 2014, 161, 431–440 CrossRef CAS PubMed.
- C. R. He, Y. Y. Kuo and S. Y. Li, Lignocellulosic butanol production from Napier grass using semi-simultaneous saccharification fermentation, Bioresour. Technol., 2017, 231, 101–108 CrossRef CAS PubMed.
- T. G. Li, Y. R. Wu and J. Z. He, Heterologous expression, characterization and application of a new beta-xylosidase identified in solventogenic Clostridium sp. strain BOH3, Process Biochem., 2018, 67, 99–104 CrossRef CAS.
- Y. D. Wu, et al., High temperature simultaneous saccharification and fermentation of corn stover for efficient butanol production by a thermotolerant Clostridium acetobutylicum, Process Biochem., 2021, 100, 20–25 CrossRef CAS.
- N. Qureshi, B. C. Saha and M. A. Cotta, Butanol production from wheat straw by simultaneous saccharification and
fermentation using Clostridium beijerinckii: Part II - Fed-batch fermentation, Biomass Bioenergy, 2008, 32(2), 176–183 CrossRef CAS.
- M. Seifollahi and H. Amiri, Enhanced production of cellulosic butanol by simultaneous co-saccharification and fermentation of water-soluble cellulose oligomers obtained by chemical hydrolysis, Fuel, 2019, 263(2823), 116759 Search PubMed.
- M. Balat, Production of bioethanol from lignocellulosic materials via the biochemical pathway: A review, Energy Convers. Manage., 2011, 52(2), 858–875 CrossRef CAS.
- A. Valles, et al., Fed-batch simultaneous saccharification and fermentation including in-situ recovery for enhanced butanol production from rice straw, Bioresour. Technol., 2021, 342, 126020 CrossRef CAS PubMed.
- D. G. Olson, et al., Recent progress in consolidated bioprocessing, Curr. Opin. Biotechnol., 2012, 23(3), 396–405 CrossRef CAS PubMed.
- Y. J. Jiang, et al., Recent advances of biofuels and biochemicals production from sustainable resources using co-cultivation systems, Biotechnol. Biofuels, 2019, 12, 155 CrossRef PubMed.
- P. P. Lin, et al., Consolidated bioprocessing of cellulose to isobutanol using Clostridium thermocellum, Metab. Eng., 2015, 31, 44–52 CrossRef CAS PubMed.
- T. Bao, et al., n-Butanol and ethanol production from cellulose by Clostridium cellulovorans overexpressing heterologous aldehyde/alcohol dehydrogenases, Bioresour. Technol., 2019, 285, 121316 CrossRef CAS PubMed.
- L. Tian, et al., Metabolic engineering of Clostridium thermocellum for n-butanol production from cellulose, Biotechnol. Biofuels, 2019, 12, 186 CrossRef PubMed.
- F. Salimi and R. Mahadevan, Characterizing metabolic interactions in a clostridial co-culture for consolidated bioprocessing, BMC Biotechnol., 2013, 13, 95 CrossRef CAS PubMed.
- S. Nakayama, et al., Butanol production from crystalline cellulose by cocultured Clostridium thermocellum and Clostridium saccharoperbutylacetonicum N1-4, Appl. Environ. Microbiol., 2011, 77(18), 6470–6475 CrossRef CAS PubMed.
- Y. J. Jiang, et al., Consolidated bioprocessing performance of a two-species microbial consortium for butanol production from lignocellulosic biomass, Biotechnol. Bioeng., 2020, 117(10), 2985–2995 CrossRef CAS PubMed.
- J. Zheng, et al., Recent advances to improve fermentative butanol production: Genetic engineering and fermentation technology, J. Biosci. Bioeng., 2015, 119(1), 1–9 CrossRef CAS PubMed.
- Z. Chang, et al., Effective multiple stages continuous acetone-butanol-ethanol fermentation by immobilized bioreactors: Making full use of fresh corn stalk, Bioresour. Technol., 2016, 205, 82–89 CrossRef CAS PubMed.
- Y. Ni, et al., Continuous butanol fermentation from inexpensive sugar-based feedstocks by Clostridium saccharobutylicum DSM 13864, Bioresour. Technol., 2013, 129, 680–685 CrossRef CAS PubMed.
- X. Chi, et al., Features of a staged acidogenic/solventogenic fermentation process to improve butanol production from rice straw, Energy Fuels, 2019, 33(2), 1123–1132 CrossRef CAS.
- D. Sahoo, et al., Effect of dilute acid pretreatment of wild rice grass (Zizania latifolia) from Loktak Lake for enzymatic hydrolysis, Bioresour. Technol., 2018, 253, 252–255 CrossRef CAS PubMed.
- L. J. Jonsson, B. Alriksson and N. O. Nilvebrant, Bioconversion of lignocellulose: inhibitors and detoxification, Biotechnol. Biofuels, 2013, 6, 16 CrossRef PubMed.
- J. Singh, M. Suhag and A. Dhaka, Augmented digestion of lignocellulose by steam explosion, acid and alkaline pretreatment methods: A review, Carbohydr. Polym., 2015, 117, 624–631 CrossRef CAS PubMed.
- T. Zhao, et al., Semi-hydrolysate of paper pulp without pretreatment enables a consolidated fermentation system with in situ product recovery for the production of butanol, Bioresour. Technol., 2019, 278, 57–65 CrossRef CAS PubMed.
- C. Xue, et al., The advanced strategy for enhancing biobutanol production and high-efficient product recovery with reduced wastewater generation, Biotechnol. Biofuels, 2017, 10, 148 CrossRef PubMed.
- Y. Wu, et al., Pleiotropic regulation of a glucose-specific PTS in Clostridium acetobutylicum for high-efficient butanol production from corn stover without detoxification, Biotechnol. Biofuels, 2019, 12, 264 CrossRef PubMed.
- Z. Q. Wen, et al., Metabolic Engineering of Clostridium cellulovorans to Improve Butanol Production by Consolidated Bioprocessing, ACS Synth. Biol., 2020, 9(2), 304–314 CrossRef CAS PubMed.
- A. Ranjan, S. Khanna and V. S. Moholkar, Feasibility of rice straw as alternate substrate for biobutanol production, Appl. Energy, 2013, 103, 32–38 CrossRef CAS.
- K. Gao and L. Rehmann, ABE fermentation from enzymatic hydrolysate of NaOH-pretreated corncobs, Biomass Bioenergy, 2014, 66, 110–115 CrossRef CAS.
- F. Xia, et al., An integrated biorefinery process to produce butanol and pulp from corn straw, Ind. Crops Prod., 2019, 140, 111648 CrossRef CAS.
- K. Gao, et al., Cellulosic Butanol Production from Alkali-Pretreated Switchgrass (Panicumvirgatum) and Phragmites (Phragmites australis), Bioresour. Technol., 2014, 174, 176–181 CrossRef CAS PubMed.
- X. Sun, et al., Feasibility of using biochar as buffer and mineral nutrients replacement for acetone-butanol-ethanol production from non-detoxified switchgrass hydrolysate, Bioresour. Technol., 2020, 298, 122569 CrossRef CAS PubMed.
- I. Valdez-Vazquez, et al., Hydrogen and butanol production from native wheat straw by synthetic microbial consortia integrated by species of Enterococcus and Clostridium, Fuel, 2015, 159, 214–222 CrossRef CAS.
- G. X. Qi, et al., Enhanced butanol production from ammonium sulfite pretreated wheat straw by separate hydrolysis and fermentation and simultaneous saccharification and fermentation, Sustain. Energy Technol. Assess., 2019, 36, 100549 Search PubMed.
- N. Qureshi, M. A. Cotta and B. C. Saha, Bioconversion of barley straw and corn stover to butanol (a biofuel) in integrated fermentation and simultaneous product recovery bioreactors, Food Bioprod. Process., 2014, 92(C3), 298–308 CrossRef CAS.
- S. V. Avula, S. C. Reddy and L. V. Reddy, The Feasibility of Mango (Mangifera indica L.) Peel as an Alternative Substrate for Butanol Production, Bioresources, 2015, 10(3), 4453–4459 CrossRef.
- F. Raganati, et al., Butanol Production by Fermentation of Fruit Residues, Chem. Eng. Trans., 2016, 49, 229–234 Search PubMed.
- D. Johnravindar, et al., Waste-to-biofuel: production of biobutanol from sago waste residues, Environ. Technol., 2017, 38(13–16), 1725–1734 CrossRef CAS PubMed.
- N. Thieme, et al., Milling byproducts are an economically viable substrate for butanol production using clostridial ABE fermentation, Appl. Microbiol. Biotechnol., 2020, 104(20), 8679–8689 CrossRef CAS PubMed.
- Q. He and H. Z. Chen, Increased efficiency of butanol production from spent sulfite liquor by removal of fermentation inhibitors, J. Cleaner Prod., 2020, 263, 121356 CrossRef CAS.
- M. L. Xia, et al., Development of optimal steam explosion pretreatment and highly effective cell factory for bioconversion of grain vinegar residue to butanol, Biotechnol. Biofuels, 2020, 13(1), 111 CrossRef CAS PubMed.
- Q. Jin, et al., High acetone-butanol-ethanol production from food waste by recombinant Clostridium saccharoperbutylacetonicum in batch and continuous immobilized-cell fermentation, ACS Sustainable Chem. Eng., 2020, 8(26), 9822–9832 CrossRef CAS.
- N. Qureshi, et al., Production of acetone-butanol-ethanol (ABE) from concentrated yellow top press cake using Clostridium beijerinckii P260, J. Chem. Technol. Biotechnol., 2020, 95(3), 614–620 CrossRef CAS.
- L. V. Reddy, A. S. Veda and Y. J. Wee, Utilization of banana crop residue as an agricultural bioresource for the production of acetone-butanol-ethanol by Clostridium beijerinckii YVU1, Lett. Appl. Microbiol., 2020, 70(1), 36–41 CrossRef CAS PubMed.
- A. M. Zetty-Arenas, et al., Towards enhanced n-butanol production from sugarcane bagasse hemicellulosic hydrolysate: Strain screening, and the effects of sugar concentration and butanol tolerance, Biomass Bioenergy, 2019, 126, 190–198 CrossRef CAS.
- W. Tao, et al., Introducing transglutaminase with its precursor region into Clostridium acetobutylicum improves its tolerance to oxidative stress and solvent production, Process Biochem., 2015, 50(1), 111–118 CrossRef CAS.
- B. Bharathiraja, et al., Biobutanol - An impending biofuel for future: A review on upstream and downstream processing techniques, Renewable Sustainable Energy Rev., 2017, 68, 788–807 CrossRef.
- A. Sanchez, et al., Bidimensional sustainability analysis of lignocellulosic ethanol production processes. Method and case study, Biofuels, Bioprod. Biorefin., 2014, 8(5), 670–685 CrossRef CAS.
- I. Valdez-Vazquez, et al., A comparison of biological, enzymatic, chemical and hydrothermal pretreatments for producing biomethane from Agave bagasse, Ind. Crops Prod., 2020, 145, 112160 CrossRef CAS.
- S. H. Shen, et al., Enhanced alcohol titre and ratio in carbon monoxide-rich off-gas fermentation of Clostridium carboxidivorans through combination of trace metals optimization with variable-temperature cultivation, Bioresour. Technol., 2017, 239, 236–243 CrossRef CAS PubMed.
- S. Chakraborty, et al., Enrichment of a solventogenic anaerobic sludge converting carbon monoxide and syngas into acids and alcohols, Bioresour. Technol., 2019, 272, 130–136 CrossRef CAS PubMed.
- V. Muller, New horizons in acetogenic conversion of one-carbon substrates and biological hydrogen storage, Trends Biotechnol., 2019, 37(12), 1344–1354 CrossRef PubMed.
|
This journal is © The Royal Society of Chemistry 2022 |
Click here to see how this site uses Cookies. View our privacy policy here.